Fibril-forming motif of non-expanded ataxin-3 revealed by scanning proline mutagenesis
Abstract
Aims: The misfolding of ataxin-3 in neurons is the hallmark of a neurodegenerative disease, spinocerebellar ataxia type 3 (SCA3), also known as Machado-Joseph disease (MJD). Ataxin-3 consists of a N-terminal Josephin domain and a C-terminal polyglutamine (polyQ) tract. The length of the polyQ tract is positively correlated with the disease. The aggregation of ataxin-3 in vitro is a two-step process, with the first step involving the aggregation of the Josephin domain and the second step involving an expanded polyQ tract. However, the fibril-forming motif of the Josephin domain is not well understood.
Methods: In this study, we employed 3D profile algorithm and scanning proline mutagenesis to identify the fibril-forming motif of non-expanded ataxin-3.
Results: By using thioflavin T fluorescence kinetics, sarkosyl-insoluble SDS-PAGE, transmission electron microscopy (TEM), and Fourier transform infrared spectroscopy (FTIR), we identified the fibril-forming motif of the Josephin domain of non-expanded ataxin-3 as 79VISNAL84.
Conclusions: We demonstrated that the proline mutation in the fibril-forming motif of the Josephin domain could inhibit the aggregation of expanded ataxin-3, which shows some therapeutic promise.
Keywords
INTRODUCTION
Protein misfolding is a pathological characteristic of neurodegenerative diseases, leading to the formation of amyloid fibrils. Polyglutamine (polyQ) diseases are a group of neurodegenerative diseases caused by the misfolding of proteins containing expanded polyQ tracts[1]. There are nine members in the polyQ disease family: spinal bulbar muscular atrophy, Huntington’s disease, dentatorubral-pallidoluysian atrophy, and spinocerebellar ataxia (SCA) types 1, 2, 3, 6, 7, and 17[1-3].
Early research on polyQ diseases has focused on the relationship between the length of polyQ tract and diseases. Researchers have identified several shared features among polyQ diseases. First, the length of polyQ tract is in positive correlation with the risk of disease onset and in negative correlation with the age of disease onset. Second, intracellular aggregates and inclusions containing polyglutamine protein have been detected in tissues of all polyQ disease patients. Third, in vitro studies have shown that polyglutamine protein can form amyloid fibrils and the aggregation rate increases as the length of polyQ tract increases. Hereby, researchers have proposed the toxic gain of function hypothesis that polyQ diseases are caused by the aggregation of expanded polyQ tracts[4].
Although polyQ diseases share some features, each polyQ disease possesses distinctive symptomatology and pathology. PolyQ proteins are different in size, cellular localization, and biological function, implying that the toxic effect of a given polyQ expansion depends on the specific protein context[3].
SCA3 or Machado-Joseph disease (MJD) is the most common form of spinocerebellar ataxia. The gene associated with SCA3 is ATXN3, which is mapped to chromosome 14q32.1[5]. It encodes the protein
Some in vitro studies show that non-expanded ataxin-3 forms fibrils under certain conditions[1], including exposure to metal ions[12], high temperatures, and high pressures[13], as well as under physiological conditions[14-16]. The aggregation of non-expanded ataxin-3 is mainly contributed by the N-terminal globular Josephin domain. Researchers proposed a two-step process hypothesis for the misfolding of expanded ataxin-3. The first step is that the Josephin domain assembles into SDS soluble aggregates, and the second step is the SDS insoluble aggregates formed by an expanded polyQ tract[15].
Researchers have identified some important residues in the aggregation of the Josephin domain. Some bioinformatics methods including Aggrescan[17], Tango[18], Waltz[19], Pasta[20], and Zyggregator[21] indicated that residues 73-96 of the Josephin sequence have a high aggregation propensity[22]. Seeding experiments indicated that residues 73-96 can significantly reduce the aggregation lag time of non-expanded ataxin-3 and expanded ataxin-3[23]. Mutation I77K/Q78K and W87K can inhibit the aggregation of the Josephin domain[22]. Molecular dynamics simulations indicated that 84LKVW87 exposes to solution after the structural global rearrangements caused by the interaction of Josephin domains[24].
The structure of the amyloid fibril contains cross- core and non-core domains. The sequence in the cross- core is called the fibril-forming motif that contains about 6 residues[25,26]. However, the fibril-forming motif of the Josephin domain is not well understood. In this study, we employed 3D profile algorithm and scanning proline mutagenesis to identify the fibril-forming motif of non-expanded ataxin-3. By using thioflavin T (ThT) fluorescence kinetics, transmission electron microscopy (TEM), Fourier transform infrared spectroscopy (FTIR), and other biophysical methods, we identified the fibril-forming motif of the Josephin domain as 79VISNAL84. We also demonstrated that the proline mutation in the fibril-forming motif of the Josephin domain inhibited the misfolding of expanded ataxin-3.
MATERIALS AND METHODS
Materials
ThT was purchased from Sigma-Aldrich (St. Louis, MO, USA). Dithiothreitol (DTT), sodium azide (NaN3), and sarkosyl were obtained from Amresco (Solon, OH, USA).
Plasmid construction and protein purification
For prokaryotic expression, wild-type human Ataxin-3(22Q), Ataxin-3(45Q), and their mutations were constructed into the pET-22b(+) plasmids. Each kind of the protein included a C-terminal His tag (LEHHHHHH). All the His-tag fused proteins were overexpressed in E. coli BL21 (DE3) strain (Novagen) and purified by a Ni2+-NTA column (GE Healthcare, NY, USA). Protein was stored at -80 °C.
3D profile algorithm prediction
To predict the fibril-forming motif of non-expanded ataxin-3, we used the 3D profile algorithm developed by Eisenberg’s lab[27,28]. We searched the protein ataxin-3 in the database ZipperDB on line. The URL of the ZipperDB is http://services.mbi.ucla.edu/zipperdb/.
Fibril formation
50 µM ataxin-3 and its mutations were incubated at pH 7.4 in Tris-buffered saline (TBS) (10 mM Tris,
ThT binding assays
A 2.5 mM ThT stock solution was freshly prepared in 10 mM TBS buffer (pH 7.0) and passed through a 0.22-μm pore size filter before use to remove insoluble particles. A series of 80 μL samples were taken in chronological order, and mixed with 320 μL of 10 mM TBS buffer and 3.2 μL of ThT stock solution before measurements. The fluorescence of ThT was excited at 440 nm with a slit width of 5.0 nm and the emission was measured at 480 nm with a slit width of 5.0 nm on an LS-55 luminescence spectrometer (PerkinElmer Life Sciences, Shelton, CT). Kinetic parameters were determined by fitting ThT fluorescence intensity vs. time to a Hill equation:
Here F(∞) is the fluorescence intensity in the long time limit, t50 is the elapsed time at which F is equal to one-half of F(∞), and n is a cooperativity parameter.
Sarkosyl-insoluble SDS-PAGE
A series of 80 µL human Ataxin samples were taken in chronological order during fibril formation corresponding to ThT binding assays. 80 µL samples were added with 320 µL 1% sarkosyl. The mixture was left at room temperature for 2 h and then centrifugated on a CP100NX ultracentrifuge (Hitachi, Japan) at 150,000 g for 30 min. The supernatant (sarkosyl-soluble ataxin-3) was removed, and the pellet (sarkosyl-insoluble ataxin-3) was resuspended in 30 mL of 2× SDS loading buffer containing 5% β-mercaptoethanol and subjected to 15% SDS-PAGE. After the electrophoresis, the gels were stained by Coomassie blue R250.
TEM
Sample aliquots of 10 µL were placed on copper grids and left at room temperature for 1-2 min, rinsed twice with H2O, and then stained with 2% (w/v) uranyl acetate for another 1-2 min. The stained samples were examined using an H-8100 (or an H-7000 FA) transmission electron microscope (Hitachi, Tokyo, Japan) operating at 100 kV.
Circular dichroism spectroscopy
Circular dichroism (CD) spectra were obtained by using a Jasco J-810 spectropolarimeter (Jasco Corp., Tokyo, Japan) with a thermostated cell holder. Quartz cell with a 1 mm light path was used for measurements in the far-ultraviolet (far-UV) region. Spectra were recorded from 195 to 250 nm for far-UV CD.
FTIR
Attenuated total reflection FTIR spectra were recorded using a Nicolet 5700 FTIR spectrophotometer (Thermo Electron, Madison, WI).
Cell culture and transfection
SH-SY5Y neuroblastoma cells were cultured in Dulbecco’s modified Eagle’s medium (DMEM) supplemented with 10% (v/v) fetal bovine serum (FBS), 100 U/mL penicillin, and 100 U/mL streptomycin in 5% CO2 at 37 °C. SH-SY5Y cells were transiently transfected with FLAG-tagged human ataxin-3 in phage vector using Lipofectamine® 2000 (Invitrogen, Carlsbad, CA) according to the manufacturer’s protocol.
Confocal microscopy
SH-SY5Y cells transiently over-expressing FLAG-tagged wild-type ataxin-3 and mutants were cultured in media for 48 h. Cells were fixed with 4% paraformaldehyde, ruptured with 0.25% Triton-X 100, stained with 0.1% thioflavin S (ThS), coimmunostained with primary monoclonal antibodies anti-FLAG and secondary Alexa Fluo-546, and visualized by confocal microscopy.
RESULTS
Fibril-forming motif predicted by 3D profile algorithm
To predict the fibril-forming motif of non-expanded ataxin-3, we used the 3D profile algorithm developed by Eisenberg’s lab[27,28]. This algorithm has been successfully used to predict the fibril-forming motif of Tau[27] and SOD1[29]. 3D profile algorithm is a structure-based algorithm. This algorithm uses the crystal structure of the fibril-forming peptide NNQQNY from the sup35 prion protein which makes up the cross- spine of amyloid-like fibrils[30]. Each six-residue peptide not containing a proline from a putative protein sequence is threaded onto the NNQQNY structure backbone, and the energetic fit is evaluated by using the RosettaDesign program[31]. To avoid problems with their disulfide bonding abilities, cysteines were substituted for serines during modeling. Segments with energies below the threshold of -23 kcal/mol are deemed to have high fibrillation propensity[28].
The Rosetta energies of sequential hexapeptides of non-expanded ataxin-3 are plotted in Figure 1. The green columns mean that the Rosetta energies are above the threshold of -23 kcal/mol (gray line), and the red columns mean that the Rosetta energies are below the threshold of -23 kcal/mol. We found that most of the columns are green, indicating that most of the hexapeptides do not tend to form fibrils [Figure 1]. Some of the hexapeptides are not included in the profile because they contain prolines which are β-strand breakers.
Figure 1. Most of the hexapeptides of non-expanded ataxin-3 did not tend to form fibrils. Diagram of the 3D-profile Rosetta energies
We arranged the hexapeptides from low to high according to the Rosetta energies. The results of the top ten hexapeptides are shown in Table 1. We found that five of the top ten hexapeptides are located at 74-84. They are 76SIQVIS81, 79VISNAL84, 75FSIQVI80, 74FFSIQV79, and 78QVISNA83. Ricchelli et al. have predicted that 73-96 is the aggregation-prone region of ataxin-3 by other computer algorithms, supporting our conclusion[12].
The top ten hexapeptides with the lowest Rosetta energy
Position | Sequence | Rosetta energy (kcal/mol) |
76-81 | SIQVIS | -28.300 |
111-116 | SFICNY | -27.600 |
159-164 | GYSIFV | -27.400 |
12-17 | SLCAQH | -26.500 |
79-84 | VISNAL | -26.200 |
75-80 | FSIQVI | -26.100 |
33-38 | LSSIAH | -25.500 |
74-79 | FFSIQV | -25.300 |
161-166 | SIFVVK | -25.200 |
78-83 | QVISNA | -25.200 |
Fibril-forming motif identified by scanning proline mutagenesis
The secondary structure of the core of amyloid is the β-sheet. As the β-sheet breaker, proline inhibits the fibrillization of proteins[32]. Scanning proline mutagenesis has been successfully used in identifying the fibril-forming motif of α-crystallin[33], Sup35 prion protein[34], and Aβ40[35]. Thus, we investigated every scanning proline mutation from F74P to L89P in this manuscript.
Based on the bioinformatics method of 3D-profile, we constructed proline mutation from F74, using the ataxin-3(22Q) as a template. We expressed and purified the proline mutations from Escherichia coli and incubated them under physiological conditions. We initially analyzed ThT fluorescence kinetics for the aggregation of all ataxin-3 mutations. The results of ThT fluorescence kinetics are shown in Figure 2 and the fitting data in Table 2. The wild-type ataxin-3(22Q) forms ThT-positive aggregates under physiological conditions, and the kinetic curve of the wild-type follows an S pattern, consistent with a nucleation-polymerization model [Figure 2]. Different mutations have different influences on ataxin-3(22Q) and the main affected parameter is F(∞) [Figure 2]. Figure 2 presents the intuitive comparison of the F(∞) of different mutations. The F(∞) of wild-type ataxin-3(22Q) is the highest, and from ataxin-3(22Q)/F74P, the
Figure 2. Different mutations have different influences on ataxin-3(22Q). Kinetic curves for the aggregation of ataxin-3(22Q) (black), F74P (red), F75P (green), S76P (blue), I77P (magenta), I80P (wine), A83P (navy), and V86P (pink), monitored by ThT fluorescence. The concentration of ataxin-3(22Q) and mutations was 50 M, and 10 mM TBS buffer (pH 7.4) containing 5 mM DTT was used. The assays were carried out at 37 °C, and the observation time was 168 h. DTT: Dithiothreitol.
Kinetic parameters for the aggregation of ataxin-3(22Q), ataxin-3(45Q), and their mutations
F (∞) | t50 (h) | n | |
22Q | 656.9 ± 9.9 | 26.7 ± 0.9 | 3.5 ± 0.5 |
22Q/F74P | 523.6 ± 8.3 | 24.6 ± 0.9 | 2.9 ± 0.4 |
22Q/I77P | 425.8 ± 59.7 | 93.3 ± 21.4 | 1.3 ± 0.2 |
22Q/I80P | 114.3 ± 15.1 | 16.9 ± 4.2 | 1.2 ± 0.8 |
22Q/A83P | 139.1 ± 22.2 | 23.5 ± 6.0 | 1.3 ± 0.8 |
22Q/V86P | 206.8 ± 8.8 | 19.1 ± 2.8 | 2.3 ± 1.0 |
22Q/L89P | 210.0 ± 7.5 | 10.5 ± 2.0 | 1.2 ± 0.4 |
22Q/I92P | 484.3 ± 25.2 | 60.8 ± 3.6 | 4.4 ± 1.0 |
22Q/I80A | 440.2 ± 8.7 | 17.4 ± 8.0 | 4.5 ± 6.5 |
22Q/6G | 245.3 ± 18.0 | 20.3 ± 4.0 | 2.1 ± 1.3 |
45Q | 808.4 ± 4.5 | 7.1 ± 0.7 | 2.4 ± 0.4 |
45Q/I80P | 189.1 ± 14.0 | 6.8 ± 3.6 | 1.4 ± 1.1 |
We then analyzed the aggregation kinetics by sarkosyl-insoluble SDS-PAGE [Figure 3]. Sarkosyl is commonly used to purify proteins from inclusion bodies[36]. As a mild detergent, sarkosyl has also been used to extract the fibrils of Tau protein from the brain tissue of Alzheimer’s disease[37] and the fibrils of
Figure 3. Different mutations have different influences on ataxin-3(22Q) based on time-dependent SDS-PAGE analysis. 50 µM ataxin-3(22Q) or proline mutations were incubated in 10 mM TBS buffer (pH 7.4) at 37 °C. Aliquots were taken at 0, 1, 2, 3, 4, 5, 6, and 7 days, respectively, and then incubated with TBS buffer containing 1% sarkosyl followed by centrifuging at 150,000 × g for 30 min. Pellets were resuspended with sample buffer containing 5% 2-mercaptoethanol and subjected to 15% SDS-PAGE. Gels were stained with Coomassie Blue. TBS: Tris-buffered saline.
Next, we observed the fibril morphology of different proline mutations by TEM [Figure 4]. The amyloid fibrils of wild-type ataxin-3(22Q) were worm-like with a diameter of 10-20 nm and a length of 100-200 nm, and the fibrils of ataxin-3(22Q)/F74P were not significantly different from the wild-type ataxin-3(22Q)
Figure 4. Different mutations have different influences on ataxin-3(22Q) fibril morphology based on TEM measurements of the Ataxin-3(22Q) and proline mutations. Negative-stain transmission electron micrographs of the following mutants: (A) ataxin-3(22Q); (B) ataxin-3(22Q)/F74P; (C) ataxin-3(22Q)/S81P; (D) ataxin-3(22Q)/N82P; (E) ataxin-3(22Q)/L89P; (F) and ataxin-3(22Q)/I92P. The concentration of ataxin-3(22Q) and mutations was 50 M, and 10 mM TBS buffer (pH 7.4) containing 5 mM DTT was used. The assays were carried out at 37 °C, and the observation time was 3 days. The scale bars represent 200 nm. TEM: Transmission electron microscopy; DTT: dithiothreitol.
The changes in secondary structures of the wild-type ataxin-3(22Q) and its proline mutations were monitored using far-UV CD spectroscopy [Figure 5] and FTIR [Figure 6]. On day 0, the wild-type ataxin-3(22Q) and its proline mutations showed similar CD spectra, having double minima at 208 and 222 nm
Figure 5. The fibril-forming motif of the Josephin domain was identified based on CD measurements of ataxin-3(22Q) and proline mutations. Far-UV CD spectra of the following mutations: (A) ataxin-3(22Q); (B) ataxin-3(22Q)/F74P; (C) ataxin-3(22Q)/S81P; (D) ataxin-3(22Q)/N82P; (E) ataxin-3(22Q)/L89P; and (F) ataxin-3(22Q)/I92P. The experimental conditions are the same as those in Figure 4. CD: Circular dichroism; far-UV: far-ultraviolet.
Figure 6. The fibril-forming motif of the Josephin domain was identified based on FTIR measurements of ataxin-3(22Q) and proline mutations. FTIR of the following mutations: (A) ataxin-3(22Q); (B) ataxin-3(22Q)/F74P; (C) ataxin-3(22Q)/S81P; (D) ataxin-3(22Q)/N82P; (E) ataxin-3(22Q)/L89P; and (F) ataxin-3(22Q)/I92P. The experimental conditions are the same as those in Figure 4. FTIR: Fourier transform infrared spectroscopy.
Collectively, by using ThT fluorescence kinetics, sarkosyl-insoluble SDS-PAGE, TEM, circular dichroism, and FTIR, we identified the fibril-forming motif of the Josephin domain as 79VISNAL84.
Alanine mutation in fibril-forming motif did not affect the aggregation of non-expanded ataxin-3
Alanine mutation has been frequently used in neutral mutation to study the function of amino acids because the side chain of alanine is methyl. We used alanine mutation as a control to demonstrate that the aggregation of non-expanded ataxin-3 is caused by the fibril-forming motif sequence but not hydrophobic residues. We mutated the 80th amino acid isoleucine to alanine. The Rosetta energy of the new sequence VASNAL is -25.600 kcal/mol, which is higher than VISNAL [Table 2] but lower than the threshold of
The kinetic parameter F(∞) of ataxin-3(22Q)/I80A was remarkably lower than that of wild-type ataxin-3(22Q), consistent with the Rosetta energy [Figure 7A and Table 2]. The fibril morphology of ataxin-3(22Q)/I80A was worm-like, consistent with that of wild-type ataxin-3(22Q) [Figure 7B]. The sarkosyl-insoluble fibrils formed after 2 days, which is later than the wild-type ataxin-3(22Q) [Figure 7C]. The CD spectra of ataxin-3(22Q)/I80P were similar to the wild-type ataxin-3(22Q). After incubated under physiological conditions for 3 days, the ellipticity between 215 to 220 nm reduced [Figure 7D], indicating the formation of amyloid fibrils with predominant β-sheet structure, similar to wile-type ataxin-3(22Q). From the FTIR results, we reached the same conclusion [Figure 7E]. Collectively, alanine mutation in fibril-forming motif did not affect the misfolding of non-expanded ataxin-3.
Figure 7. All residues in fibril-forming motif mutated to glycine inhibit the aggregation of non-expanded ataxin-3. (A) ThT binding assays of ataxin-3(22Q), ataxin-3(22Q)/I80A, and ataxin-3(22Q)/6G. The kinetic curves are fitted with Hill function; (B) TEM measurements of ataxin-3(22Q)/I80A (left) and ataxin-3(22Q)/6G (right); (C) Time-dependent SDS-PAGE analysis of sarkosyl-insoluble ataxin-3(22Q), ataxin-3(22Q)/I80A, and ataxin-3(22Q)/6G. The scale bars represent 200 nm; (D) CD measurements of ataxin-3(22Q)/I80A (left) and ataxin-3(22Q)/6G (right); (E) FTIR measurements of ataxin-3(22Q)/I80A (left) and ataxin-3(22Q)/6G (right). ThT: thioflavin T; TEM: transmission electron microscopy; CD: circular dichroism; FTIR: Fourier transform infrared spectroscopy.
All residues in fibril-forming motif mutated to glycine inhibit the misfolding of non-expanded ataxin-3
According to the steric zipper model, cross-β structure is constituted by the complementary side chains of two β-sheets[43]. Because glycine is the smallest amino acid whose side chain is a hydrogen atom, glycine residue is not beneficial to the formation of cross-β structure.
Therefore, polyglycine mutation is usually used as a negative control for research of fibril-forming motifs[43]. We mutated VISNAL to GGGGGG and named the mutation ataxin-3(22Q)/6G. The Rosetta energy of GGGGGG calculated by 3D profile algorithm is -18.200 kcal/mol, which is significantly higher than the threshold of -23 kcal/mol. Therefore, we speculated that ataxin-3(22Q)/6G would not form fibrils or remarkably inhibit aggregation.
The kinetic parameter F(∞) of ataxin-3(22Q)/6G was significantly lower than the wild-type ataxin-3(22Q), consistent with the Rosetta energy [Figure 7A and Table 2]. The worm-like fibril morphology could be observed by TEM for ataxin-3(22Q)/6G, but the fibril length was shorter and the fibrils were fewer than the wild-type ataxin-3(22Q) [Figure 7B]. The sarkosyl-insoluble fibrils formed after 3 days, which is later than the wild-type ataxin-3(22Q) [Figure 7C]. After being incubated under physiological conditions for 3 days, the ellipticity between 215 and 220 nm decreased [Figure 7D], indicating the formation of amyloid fibrils with predominant β-sheet structure, similar to wile-type ataxin-3(22Q). From the FTIR results, we reached the same conclusion [Figure 7E]. Taken together, all residues in fibril-forming motif mutated to glycine inhibited the misfolding of non-expanded ataxin-3.
Proline mutation in fibril-forming motif also inhibits the misfolding of expanded ataxin-3
All our research objects above were non-expanded ataxin-3. We also want to know the character of the fibril-forming motif in the aggregation of expanded ataxin-3. Bottomley’s lab has found that small heat shock protein αB-crystallin interacts with the Josephin domain to inhibit the aggregation of the Josephin domain and the aggregation of ataxin-3(64Q)[44]. Therefore, we speculated that proline mutation in fibril-forming motifs would also inhibit the misfolding of expanded ataxin-3. We constructed mutation ataxin-3(45Q)/I80P and compared its aggregation ability with ataxin-3(45Q).
Compared with ataxin-3(22Q), the kinetic parameter F(∞) of ataxin-3(45Q) was higher and t50 was lower [Table 2], indicating that the fibrillization rate of ataxin-3(45Q) is faster than ataxin-3(22Q). The kinetic parameter F(∞) of ataxin-3(45Q)/I80P was significantly lower than ataxin-3(45Q) [Figure 8A and Table 2]. After 2 days, ataxin-3(45Q)/I80P produced very small amounts of sarkosyl-insoluble fibrils [Figure 8B]. The fibril morphology of ataxin-3(45Q) was significantly different from ataxin-3(22Q), and the fibril length of ataxin-3(45Q) was more than 200 nm, which was longer than ataxin-3(22Q) [Figure 8B], suggesting that the expanded polyQ participated in fibril-forming of expanded ataxin-3 and changed the fibril morphology. Ataxin-3(45Q)/I80P also formed fibril observed by TEM, and the fibril morphology of ataxin-3(45Q)/I80P was similar to but shorter than ataxin-3(45Q) [Figure 8B]. The sarkosyl-insoluble fibrils formed after 2 days, which is later than ataxin-3(45Q) [Figure 8C]. The CD spectra of ataxin-3(45Q)/I80P was similar to ataxin-3(45Q). After being incubated under physiological conditions for 3 days, the ellipticity between 215 nm to 220 nm reduced [Figure 8D], indicating the formation of amyloid fibrils with predominant β-sheet structure, similar to ataxin-3(45Q). From the FTIR results, we reached the same conclusion [Figure 8E]. Collectively, mutation I80P inhibited the aggregation of ataxin-3(45Q).
Figure 8. Proline mutation in fibril-forming motif inhibits the aggregation of expanded ataxin-3. (A) ThT binding assays of ataxin-3(45Q) and ataxin-3(45Q)/I80P. The kinetic curves are fitted with Hill function; (B) TEM measurements of ataxin-3(45Q) (left) and ataxin-3(45Q)/I80P (right); (C) Time-dependent SDS-PAGE analysis of sarkosyl-insoluble ataxin-3(45Q) and ataxin-3(45Q)/I80P. The scale bars represent 200 nm; (D) CD measurements of ataxin-3(45Q) (left) and ataxin-3(45Q)/I80P (right); (E) FTIR measurements of ataxin-3(45Q) (left) and ataxin-3(45Q)/I80P (right). TEM: Transmission electron microscopy; CD: circular dichroism; FTIR: Fourier transform infrared spectroscopy.
Sequence-dependent abnormal aggregation of non-expanded ataxin-3 and expanded ataxin-3 in SH-SY5Y cells
We wanted to know the character of the fibril-forming motif in the aggregation of non-expanded ataxin-3. We found that wild-type ataxin-3(22Q) [Figure 9A] and ataxin-3(22Q)-F74P [Figure 9B] over-expressed in SH-SY5Y neuroblastoma cells did form aggregates in the cells. In sharp contrast, no aggregates were observed in either ataxin-3(22Q)-I80P [Figure 9C] or ataxin-3(22Q)-A83P [Figure 9D]. The above results demonstrated the sequence-dependent abnormal aggregation of ataxin-3(22Q) in SH-SY5Y cells and identified the fibril-forming motif of the Josephin domain in cells as 79VISNAL84.
Figure 9. Sequence-dependent abnormal aggregation of human ataxin-3(22Q) takes place in SH-SY5Y cells. (A) Wild-type ataxin-3(22Q) and (B) ataxin-3(22Q)-F74P over-expressed in SH-SY5Y neuroblastoma cells formed aggregates in the cells. In contrast, no aggregates were observed in either (C) ataxin-3(22Q)-I80P or (D) ataxin-3(22Q)-A83P. SH-SY5Y cells were stained with 0.1% ThS, coimmunostained with primary monoclonal antibodies Anti-FLAG and secondary Alexa Fluo-546, and visualized by confocal microscopy. The scale bar represents 10 m. ThS: Thioflavin S.
We also wanted to know the character of the fibril-forming motif in the aggregation of expanded ataxin-3. We found that wild-type ataxin-3(45Q) [Figure 10A], ataxin-3(45Q)-F74P mutant [Figure 10B], and ataxin-3(45Q)-I92P mutant [Figure 10C] overexpressed in SH-SY5Y cells did form aggregates in the cells. In sharp contrast, no aggregates were observed in ataxin-3(45Q)-I80P [Figure 10D]. The above results demonstrated the sequence-dependent abnormal aggregation of ataxin-3(45Q) in SH-SY5Y cells.
Figure 10. Sequence-dependent abnormal aggregation of human ataxin-3(45Q) takes place in SH-SY5Y cells. (A) Wild-type ataxin-3(45Q), (B) ataxin-3(45Q)-F74P mutant, (C) and ataxin-3(45Q)-I92P mutant overexpressed in SH-SY5Y cells formed aggregates in the cells; In contrast, no aggregates were observed in (D) ataxin-3(45Q)-I80P. The experimental conditions are the same as those in Figure 9.
It should be mentioned that in this manuscript, we defined ataxin-3(45Q) as an expanded ataxin-3. But this can only be considered an intermediate amplification range. The pathogenic expansion (full penetrance) of ataxin-3 requires more than 60 repeats. We plan to perform the experiments larger than ataxin-3(60Q) in the near future.
DISCUSSION
The misfolding of ataxin-3 in neurons is the hallmark of SCA3. Ataxin-3 is a polyQ protein, and the length of polyQ tract positively correlates with the risk of disease onset[6]. Expanded polyQ tract can aggregate itself or drive the context proteins to aggregate[15]. It has been found that the N-terminal Josephin domain plays a key role in the aggregation of non-expanded and expanded ataxin-3[14-16]. The Josephin domain aggregates before the expanded poly tract, suggesting that there are some other fibril-forming motifs besides polyQ tract. We further discuss the role of the fibril-forming motif of the Josephin domain in non-expanded and expanded ataxin-3. The fibril-forming motif of the Josephin domain determines the stability and aggregation properties of non-expanded ataxin-3[45], but the expansion of a polyglutamine tract in ataxin-3 leads to SCA3[46]. In this study, we explored the impact of specific amino acids on the misfolding properties of ataxin-3, the protein involved in SCA3 when polyglutamine expanded. We employed 3D profile algorithm and scanning proline mutagenesis to identify the fibril-forming motif of non-expanded ataxin-3 and elucidated the fibril-forming motif as 79VISNAL84. Our conclusion is supported by the following literature:
(1) Bioinformatics studies suggest that the sequence 73-96 has the highest aggregation propensity, and point mutation experiments suggest that I77K/Q78K and W87K inhibit the aggregation of the Josephin domain[22].
(2) Molecular dynamics simulation suggests that the interaction of the Josephin domain is mainly driven by Arg101, followed by slower structural global rearrangements, involving the exposure to the solvent of
(3) Limited proteolysis experiments combined with mass spectrometry analyses indicate that the trypsin proteolysis efficiency at Lys85 of expanded ataxin-3 is higher than non-expanded ataxin-3, providing evidence that an expanded polyQ tract alters the conformational dynamics of the Josephin domain. In addition, seeding experiments prove that peptide 73-96 promotes the aggregation of non-expanded and expanded ataxin-3[23].
(4) Hydrogen deuterium exchange coupled with mass spectrometry demonstrates that expanded polyQ tract increases the molecular mobility of two juxtaposed helices, 75-91 and 113-133. Fragment analysis shows that 76-81 in the two helices has the highest aggregation propensity, and proteinase K digestion indicates that residues 76-89 are the core of the Josephin domain fibril[47].
Since peptide 79VISNAL84 is the fibril-forming motif of non-expanded ataxin-3, why is this peptide retained during evolution? We suggest that the peptide 79VISNAL84 could participate in the following functions. The first possible function is the interaction of the Josephin domain and ubiquitin. Ataxin-3 is a ubiquitin-specific cysteine protease in the ubiquitin-proteasome system, and the residues Gln9, Cys14, His119, and Asn134 form a catalytic pocket to catalyze ubiquitination and Cys14 is the active site[48,49]. Ataxin-3 can bind to polyubiquitin chains with at least four ubiquitin units, and the N-terminal Josephin domain has two independent ubiquitin-binding sites. Ubiquitin-binding site 1 is near the catalytic pocket including Gln16, Ser76, Ile77, Gln78, Thr122, and Leu133, while ubiquitin-binding site 2 resides on the opposite side including Tyr27, Phe28, Val86, and Trp87[48,49]. The fibril-forming motif 79VISNAL84 is just located between the ubiquitin-binding site 1 (Ser76, Ile77, and Gln78) and the ubiquitin-binding site 2 (Val86 and Trp87). The second possible function is nuclear export signal (NES). Josephin domain has two nuclear export signals, NES77 (77IQVISNALKVWGL89) and NES141 (141ELISDTYLALFLAQLQQ E158)[50]. The fibril-forming motif 79VISNAL84 is just located inside the NES77. The mutation of this fibril-forming motif could influence the cellular localization of ataxin-3, which could interact with mutant SOD1 and promote SOD1 aggregation[51].
A key limitation of this study is that we used in vitro reconstitution approaches to demonstrate that the proline mutation in the fibril-forming motif of the Josephin domain inhibits the aggregation of expanded ataxin-3, which shows some therapeutic promise. Direct in vivo biology investigations to connect this in vitro biophysical study with functions of fibril-forming motif of non-expanded ataxin-3 in living neurons are needed in the future.
In summary, we identified the fibril-forming motif of the Josephin domain as 79VISNAL84. By several structural and bioinformatic analyses, we discovered that proline mutation of the fibril-forming motif inhibited the misfolding of the expanded ataxin-3 in cells. This result is quite significant in the context of the present knowledge in the field that aggregation of ataxin-3 is the hallmark of the neurodegenerative disease SCA3. Since this shows a proline mutation can inhibit such fibril formation, the study shows some therapeutic promise.
DECLARATIONS
Authors’ contributions
Supervised the project: Hu HY, Liang Y
Designed the experiments: Meng SR, Hu HY, Liang Y
Performed the experiments:Meng SR, Ma C, Chen J, Wang LQ
Collected and analyzed the data, wrote the manuscript: Meng SR, Liang Y
All authors proofread and approved the manuscript.
Availability of data and materials
The data that support the findings of this study are available from authors.
Financial support and sponsorship
Liang Y acknowledges fundings from the National Natural Science Foundation of China (Nos. 32271326, 32071212, and 31770833); Wang LQ acknowledges financial support from the National Natural Science Foundation of China (No. 32201040), China Postdoctoral Science Foundation (Nos. 2021TQ0252 and 2021M700103), and the Fundamental Research Funds for the Central Universities (2042022kf1047); Hu HY acknowledges fundings from the National Natural Science Foundation of China (Nos. 31700669 and 31870764).
Conflicts of interest
All authors declared that there are no conflicts of interest.
Ethical approval and consent to participate
Not applicable.
Consent for publication
Not applicable.
Copyright
© The Author(s) 2024.
REFERENCES
1. Figueiredo F, Lopes-Marques M, Almeida B, et al. A robust assay to monitor ataxin-3 amyloid fibril assembly. Cells 2022;11:1969.
2. Toulis V, Casaroli-Marano R, Camós-Carreras A, et al. Altered retinal structure and function in Spinocerebellar ataxia type 3. Neurobiol Dis 2022;170:105774.
3. do Carmo Costa M, Paulson HL. Toward understanding Machado-Joseph disease. Prog Neurobiol 2012;97:239-57.
4. Orr HT. Polyglutamine neurodegeneration: expanded glutamines enhance native functions. Curr Opin Genet Dev 2012;22:251-5.
5. Takiyama Y, Nishizawa M, Tanaka H, et al. The gene for Machado-Joseph disease maps to human chromosome 14q. Nat Genet 1993;4:300-4.
6. Matos CA, de Macedo-Ribeiro S, Carvalho AL. Polyglutamine diseases: the special case of ataxin-3 and Machado-Joseph disease. Prog Neurobiol 2011;95:26-48.
7. Margolis RL, Ross CA. Expansion explosion: new clues to the pathogenesis of repeat expansion neurodegenerative diseases. Trends Mol Med 2001;7:479-82.
8. Haas E, Incebacak RD, Hentrich T, et al. A novel SCA3 knock-in mouse model mimics the human SCA3 Disease phenotype including neuropathological, behavioral, and transcriptional abnormalities especially in oligodendrocytes. Mol Neurobiol 2022;59:495-522.
9. Paulson H, Shakkottai V. Spinocerebellar ataxia type 3. GeneReviews. 2020. Available from: https://www.ncbi.nlm.nih.gov/books/NBK1196/. [Last accessed on 6 Jun 2024].
10. Lima M, Costa MC, Montiel R, et al. Population genetics of wild-type CAG repeats in the Machado-Joseph disease gene in Portugal. Hum Hered 2005;60:156-63.
11. Ganev Y, Buhr TJ, Mahmood H. Manipulations of protective post-translational modifications of ataxin-3 as a possible treatment of SCA3. 2018. Available from: https://api.semanticscholar.org/CorpusID:195185159. [Last accessed on 6 Jun 2024].
12. Ricchelli F, Fusi P, Tortora P, et al. Destabilization of non-pathological variants of ataxin-3 by metal ions results in aggregation/fibrillogenesis. Int J Biochem Cell Biol 2007;39:966-77.
13. Marchal S, Shehi E, Harricane MC, et al. Structural instability and fibrillar aggregation of non-expanded human ataxin-3 revealed under high pressure and temperature. J Biol Chem 2003;278:31554-63.
14. Gales L, Cortes L, Almeida C, et al. Towards a structural understanding of the fibrillization pathway in Machado-Joseph’s disease: trapping early oligomers of non-expanded ataxin-3. J Mol Biol 2005;353:642-54.
15. Ellisdon AM, Thomas B, Bottomley SP. The two-stage pathway of ataxin-3 fibrillogenesis involves a polyglutamine-independent step. J Biol Chem 2006;281:16888-96.
16. Masino L, Nicastro G, De Simone A, Calder L, Molloy J, Pastore A. The Josephin domain determines the morphological and mechanical properties of ataxin-3 fibrils. Biophys J 2011;100:2033-42.
17. Conchillo-Solé O, de Groot NS, Avilés FX, Vendrell J, Daura X, Ventura S. AGGRESCAN: a server for the prediction and evaluation of “hot spots” of aggregation in polypeptides. BMC Bioinformatics 2007;8:65.
18. Fernandez-Escamilla AM, Rousseau F, Schymkowitz J, Serrano L. Prediction of sequence-dependent and mutational effects on the aggregation of peptides and proteins. Nat Biotechnol 2004;22:1302-6.
19. Maurer-Stroh S, Debulpaep M, Kuemmerer N, et al. Exploring the sequence determinants of amyloid structure using position-specific scoring matrices. Nat Methods 2010;7:237-42.
20. Trovato A, Seno F, Tosatto SC. The PASTA server for protein aggregation prediction. Protein Eng Des Sel 2007;20:521-3.
21. Tartaglia GG, Vendruscolo M. The Zyggregator method for predicting protein aggregation propensities. Chem Soc Rev 2008;37:1395-401.
22. Masino L, Nicastro G, Calder L, Vendruscolo M, Pastore A. Functional interactions as a survival strategy against abnormal aggregation. FASEB J 2011;25:45-54.
23. Scarff CA, Almeida B, Fraga J, Macedo-Ribeiro S, Radford SE, Ashcroft AE. Examination of ataxin-3 (atx-3) aggregation by structural mass spectrometry techniques: a rationale for expedited aggregation upon polyglutamine (polyQ) expansion. Mol Cell Proteomics 2015;14:1241-53.
24. Deriu MA, Grasso G, Licandro G, et al. Investigation of the Josephin Domain protein-protein interaction by molecular dynamics. PLoS One 2014;9:e108677.
25. Sawaya MR, Sambashivan S, Nelson R, et al. Atomic structures of amyloid cross-beta spines reveal varied steric zippers. Nature 2007;447:453-7.
26. Meng SR, Zhu YZ, Guo T, Liu XL, Chen J, Liang Y. Fibril-forming motifs are essential and sufficient for the fibrillization of human Tau. PLoS One 2012;7:e38903.
27. Thompson MJ, Sievers SA, Karanicolas J, Ivanova MI, Baker D, Eisenberg D. The 3D profile method for identifying fibril-forming segments of proteins. Proc Natl Acad Sci U S A 2006;103:4074-8.
28. Goldschmidt L, Teng PK, Riek R, Eisenberg D. Identifying the amylome, proteins capable of forming amyloid-like fibrils. Proc Natl Acad Sci U S A 2010;107:3487-92.
29. Ivanova MI, Sievers SA, Guenther EL, et al. Aggregation-triggering segments of SOD1 fibril formation support a common pathway for familial and sporadic ALS. Proc Natl Acad Sci U S A 2014;111:197-201.
30. Nelson R, Sawaya MR, Balbirnie M, et al. Structure of the cross-beta spine of amyloid-like fibrils. Nature 2005;435:773-8.
31. Kuhlman B, Baker D. Native protein sequences are close to optimal for their structures. Proc Natl Acad Sci U S A 2000;97:10383-8.
32. Toyama BH, Weissman JS. Amyloid structure: conformational diversity and consequences. Annu Rev Biochem 2011;80:557-85.
33. Kannan R, Raju M, Sharma KK. The critical role of the central hydrophobic core (residues 71-77) of amyloid-forming αA66-80 peptide in α-crystallin aggregation: a systematic proline replacement study. Amyloid 2014;21:103-9.
34. Chang HY, Lin JY, Lee HC, Wang HL, King CY. Strain-specific sequences required for yeast [PSI+] prion propagation. Proc Natl Acad Sci U S A 2008;105:13345-50.
35. Williams AD, Portelius E, Kheterpal I, et al. Mapping abeta amyloid fibril secondary structure using scanning proline mutagenesis. J Mol Biol 2004;335:833-42.
36. Tao H, Liu W, Simmons BN, Harris HK, Cox TC, Massiah MA. Purifying natively folded proteins from inclusion bodies using sarkosyl, Triton X-100, and CHAPS. Biotechniques 2010;48:61-4.
37. Fitzpatrick AWP, Falcon B, He S, et al. Cryo-EM structures of tau filaments from Alzheimer’s disease. Nature 2017;547:185-90.
38. Yang Y, Shi Y, Schweighauser M, et al. Structures of α-synuclein filaments from human brains with Lewy pathology. Nature 2022;610:791-5.
39. Hu JY, Zhang DL, Liu XL, et al. Pathological concentration of zinc dramatically accelerates abnormal aggregation of full-length human Tau and thereby significantly increases Tau toxicity in neuronal cells. Biochim Biophys Acta Mol Basis Dis 2017;1863:414-27.
40. Xu WC, Liang JZ, Li C, et al. Pathological hydrogen peroxide triggers the fibrillization of wild-type SOD1 via sulfenic acid modification of Cys-111. Cell Death Dis 2018;9:67.
41. Wang K, Liu JQ, Zhong T, et al. Phase separation and cytotoxicity of tau are modulated by protein disulfide isomerase and
42. Dai B, Zhong T, Chen ZX, et al. Myricetin slows liquid-liquid phase separation of Tau and activates ATG5-dependent autophagy to suppress Tau toxicity. J Biol Chem 2021;297:101222.
43. Teng PK, Eisenberg D. Short protein segments can drive a non-fibrillizing protein into the amyloid state. Protein Eng Des Sel 2009;22:531-6.
44. Robertson AL, Headey SJ, Saunders HM, et al. Small heat-shock proteins interact with a flanking domain to suppress polyglutamine aggregation. Proc Natl Acad Sci U S A 2010;107:10424-9.
45. Masino L, Nicastro G, Menon RP, Dal Piaz F, Calder L, Pastore A. Characterization of the structure and the amyloidogenic properties of the Josephin domain of the polyglutamine-containing protein ataxin-3. J Mol Biol 2004;344:1021-35.
46. Sicorello A, Różycki B, Konarev PV, Svergun DI, Pastore A. Capturing the conformational ensemble of the mixed folded polyglutamine protein ataxin-3. Structure 2021;29:70-81.e5.
47. Lupton CJ, Steer DL, Wintrode PL, Bottomley SP, Hughes VA, Ellisdon AM. Enhanced molecular mobility of ordinarily structured regions drives polyglutamine disease. J Biol Chem 2015;290:24190-200.
48. Nicastro G, Masino L, Esposito V, et al. Josephin domain of ataxin-3 contains two distinct ubiquitin-binding sites. Biopolymers 2009;91:1203-14.
49. Nicastro G, Menon RP, Masino L, Knowles PP, McDonald NQ, Pastore A. The solution structure of the Josephin domain of ataxin-3: structural determinants for molecular recognition. Proc Natl Acad Sci U S A 2005;102:10493-8.
50. Antony PMA, Mäntele S, Mollenkopf P, et al. Identification and functional dissection of localization signals within ataxin-3. Neurobiol Dis 2009;36:280-92.
Cite This Article
How to Cite
Download Citation
Export Citation File:
Type of Import
Tips on Downloading Citation
Citation Manager File Format
Type of Import
Direct Import: When the Direct Import option is selected (the default state), a dialogue box will give you the option to Save or Open the downloaded citation data. Choosing Open will either launch your citation manager or give you a choice of applications with which to use the metadata. The Save option saves the file locally for later use.
Indirect Import: When the Indirect Import option is selected, the metadata is displayed and may be copied and pasted as needed.
About This Article
Copyright
Data & Comments
Data
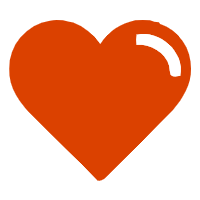
Comments
Comments must be written in English. Spam, offensive content, impersonation, and private information will not be permitted. If any comment is reported and identified as inappropriate content by OAE staff, the comment will be removed without notice. If you have any queries or need any help, please contact us at [email protected].