Melatonin ameliorates tauopathy and ferroptosis in P301S Tau transgenic mice
Abstract
Aim: Melatonin has been found to inhibit the induced Tau hyperphosphorylation in cellular and animal models. However, the underlying mechanisms are not fully understood, especially with respect to the ability of melatonin to control Tau-related pathologies and neuronal damage.
Methods: 6-month-old male P301S mice were employed to evaluate the effects of intranasally administered melatonin (10 mg/kg) on tauopathy progression and ferroptosis. HT22 and D1A cells were also employed to further uncover the mechanism of melatonin on Tau hyperphosphorylation and neuronal iron metabolism.
Results: We verified that intranasal melatonin administration effectively improved Tau hyperphosphorylation via modulating the activity of several kinases, accompanied by a stifling of synaptic loss, neuronal degeneration, neuroinflammation, and oxidative damage in P301S Tau transgenic mice. Moreover, restoration of ferroptosis-related indicators such as iron accumulation in hippocampal neurons, iron metabolism, and activating Nrf2/GPX4 signaling also contributed to the underlying mechanisms by which melatonin supplementation ameliorated behavioral deficits of the mice. Interestingly, we found that melatonin treatment may stimulate astrocytes to secrete hepcidin in vitro, which in turn helps alleviate neuronal iron efflux and Tau hyperphosphorylation in stressed conditions.
Conclusion: These findings provide compelling evidence that melatonin plays a role in improving tauopathies and ferroptosis in neurodegenerative disease.
Keywords
INTRODUCTION
Tauopathies are a heterogeneous group of neurologic diseases characterized by abnormal deposition of Tau protein in nerve cells, of which Alzheimer’s disease (AD) is the most common[1]. Under physiological conditions, the phosphorylation degree of Tau is in dynamic balance through the regulation of protein kinase and phosphatase, and then plays different functions according to the needs of the body, which is conducive to physiological processes such as cell morphology construction and signal transduction[2]. However, in tauopathies, Tau is abnormally hyperphosphorylated to eventually form paired helical filaments (PHF) and neurofibrillary tangles (NFTs), which are toxic to neurons[3]. In the absence of proven efficacy of Aβ targeted therapy in treating AD, therapeutic strategies aimed at slowing, preventing, or clearing tauopathy are considered promising target candidates, although there are currently no fully effective new therapies with low toxic side effects[4,5].
In fact, therapies that target Tau production are relatively lacking[5], making it particularly important to develop drugs that can directly or indirectly interfere with abnormal hyperphosphorylation of Tau. In addition to the role of glycogen synthase kinase 3β (GSK3β), cyclin-dependent kinase 5 (CDK5), and protein phosphatase 2A (PP2A), Tau phosphorylation may also be affected by metal ions, including iron[6]. As the most abundant transition metal in the brain, iron is known to accumulate excessively in the brains of patients with neurodegenerative diseases such as AD. Iron overload drives a series of events such as glial activation, the formation of Aβ plaques and Tau tangles, and even neuron loss[7-9], accelerating the progression of AD and cognitive decline. Accordingly, iron chelators have attracted attention, which play neuroprotective and neurorepair functions in multiple modes for multiple causes of AD[9-13].
A study has found that people with AD have reduced melatonin levels in the cerebrospinal fluid, serum, and brain compared to healthy people of the same age[14]. Moreover, as a natural and multifunctional neurohormone, the neuroprotection of melatonin in AD from animal models to early patients has also been confirmed by a series of studies[14-16]. The mechanism includes interfering with the maturation of amyloid precursor protein (APP), inhibiting the secretion of APP into various cells, and affecting the function of secretases by regulating the regulatory network of secretion enzyme expression, thereby inhibiting the processing of amyloidosis APP and directly inhibiting the production of Aβ. Additionally, melatonin prevents Aβ accumulation and reduces its neurotoxicity by interacting with Aβ[17]. Furthermore, melatonin can improve Aβ-induced neurotoxicity and promote Aβ clearance through lymphatic drainage, blood-brain barrier transport, and degradation pathways[17,18]. In addition, melatonin has been shown to distinctly block Tau hyperphosphorylation in neuronal cell lines and animal models. In vitro, melatonin can reduce Okadaic acid-induced Tau hyperphosphorylation[19], and also interact with Tau repetition domains to mediate its aggregation[20]. In vivo studies have shown that besides improving Aβ-induced Tau hyperphosphorylation[16,21], exogenous melatonin supplementation improves oxidative stress, neuroinflammation, and Tau hyperphosphorylation induced by intraventricular injection of AAV-hTauP301L virus vector by restoring autophagy flux[22], and the miR-504-3p and CDK5 axis is believed to be involved in the mechanism of melatonin ameliorating Tau-related pathology[23]. However, the diversity of its biological functions and the complexity of the pathogenesis of AD indicate that further research on the neuroprotective mechanism of melatonin is necessary, which will be beneficial to its application in clinical practice alone or in combination[24,25]. Interestingly, many studies have confirmed that melatonin has the efficacy of inhibiting ferroptosis with its iron chelation[26]. Melatonin is reported to inhibit hyperglycemia-induced ferroptosis by activating the nuclear factor erythroid 2-related factor 2 (Nrf2)/HO-1 signaling pathway in type 2 diabetic osteoporosis[27]; ferroptosis in steroid-induced osteoporosis can also be inhibited by activating the PI3K/AKT/mTOR signaling pathway[28]. There is also some therapeutic effect on ferroptosis in acute kidney injury models[29]. Combined with the iron-chelating activity of melatonin and its ability to directly remove free radicals[30], we speculated that melatonin plays a potential role in inhibiting tauopathies and ferroptosis in AD brain; however, the possible inhibitory effect remains to be elucidated.
In this study, 6-month-old P301S Tau transgenic (Tg) mice were administered noninvasive intranasal
METHODS
Animal treatments and sample preparation
Melatonin (M5250, Sigma-Aldrich) was dissolved in 100% ethanol and subsequently diluted to 12.5 mg/mL with normal saline before use. P301S Tau Tg mice on a C57BL/6 background, originally purchased from the Jackson Laboratory (Bar Harbor, ME, USA), were used as a model of tauopathy. The transgenic mice and wild-type (WT) mice were housed in individually ventilated cages with free access to water and chow in a temperature-controlled (22-25 °C) animal room and were maintained on a 12-hour light/dark cycle.
Six-month-old male P301S mice were intranasally administered melatonin (10 mg/kg; Sigma Aldrich, M5250) or saline in one nostril (eight mice per group) per day for 12 weeks. The general health and body weight of the mice were recorded daily. Ten weeks after the intranasal melatonin treatment, the mice were subjected to the Morris water maze (MWM) and nesting tests. After the behavioral experiments, mice were sacrificed under sodium pentobarbital anesthesia. The brains were immediately removed, and the left and right hemispheres were separated on an ice-cold plate. The left hemisphere was fixed with 4% paraformaldehyde and embedded in paraffin for morphological assessment. The right hemisphere was frozen at -80 °C for biochemical analyses.
Behavioral experiments
MWM tests, open field test (OFT), and nest construction tests were carried out to assess the cognitive and behavioral performance of P301S mice that were treated with melatonin or saline intranasally as previously described[13,31]. For each trial, the performance was monitored and analyzed with the SMART 3.0 computer program.
ICP-MS system
For the inductively coupled ground mass spectrometry (ICP-MS; Agilent Technologies Inc) analysis, WT mice were treated with melatonin (10 mg/kg) in one nostril. The mice were sacrificed at specific time points (0, 15, 30, and 60 min). The brain samples were prepared, and the concentrations of melatonin in the whole brain (including the olfactory bulb, cortex, and hippocampus) were analyzed by the ICP-MS system as previously described[24].
Histochemical staining
To survey iron accumulation in the mouse brain, 3,3,-diaminobenzidine tetrahydrochloride (DAB)-enhanced Perl’s staining was employed as previously described[13]. For immunohistochemistry, the paraffin sections of the brain tissues (5 μm) were dewaxed and rehydrated, and antigen retrieval was performed by boiling the sections in citric acid buffer for 3 min with a microwave oven. The sections were then incubated overnight with mouse anti-microtubule-associated protein 2 (MAP2; 1:400, Sigma-Aldrich), mouse anti-glial fibrillary acidic protein (GFAP; 1:200, Santa Cruz), goat anti-hepcidin (1:200, Santa Cruz), or rabbit anti-ionized calcium-binding adaptor molecule 1 (IBA1, 1:100; Abcam) at 4 °C. The next day, the sections were treated with the appropriate HRP-conjugated secondary antibodies and detected with 0.025% DAB according to the immunohistochemical kits. For Nissl staining, coronal sections (5 μm) were prepared and stained with Nissl staining solution (Beyotime Institute of Biotechnology) for 10 min. The sealed sections were observed, and images were collected using a microscope (DM4000B; Leica).
For immunofluorescence, the brain sections (10 μm) were incubated overnight at 4 °C with primary antibodies: mouse anti-Tau-p-Ser396 (1:400; Sigma) and rabbit anti-glutathione peroxidase 4 (GPX4; 1:200; Santa Cruz). For double staining, a mixture of primary antibodies was used. Antigen visualization was accomplished using fluorescein isothiocyanate-conjugated donkey anti-rabbit IgG (1:200; Jackson) and Texas-Red-conjugated donkey anti-mouse IgG (1:200; Jackson) for 2 h at room temperature. The images were examined using a confocal laser scanning microscope (SP8; Leica).
Cell culture and treatment
Mouse-derived astrocyte line D1A and mouse hippocampal neuronal cell line HT22 were used for the
For coculture, the medium was extracted from D1A cells of each group, and fresh culture medium was respectively mixed in a 1:1 ratio and used to treat HT22 cells. After 24 h, the HT22 cells were collected for western blotting immunoassay.
Western blotting immunoassay
Frozen brain tissues or cells were lysed in a sample buffer [0.1% sodium dodecyl sulfate (SDS), 0.5% deoxycholic acid, 1% Nonidet P-40, 150 mM NaCl, and 50 mM Tris, pH 8.0] supplemented with a cocktail of protease inhibitors (Sigma-Aldrich) and were processed for immunoblot analysis as previously described[9]. The primary antibodies used for this study are shown in Table 1.
List of biological reagents antibody
Antibody | Source | Manufacturer | Dilution |
Calpain1 | Rabbit | Abcam | 1:2,000 |
Caspase 3 | Rabbit | Cell Signaling Tech | 1:2,000 |
CDK5 | Rabbit | Abcam | 1:2,000 |
p-CDK5 | Rabbit | Sigma-Aldrich | 1:2,000 |
DMT1 | Mouse | Alpha diagnostic | 1:2,000 |
FPN | Rabbit | Abcam | 1:2,000 |
FTH | Rabbit | Cell Signaling Tech | 1:2,000 |
GFAP | Rabbit | Sigma-Aldrich | 1:2,000 |
GPX4 | Rabbit | Santa Cruz | 1:1,000 |
GSK3α/β | Rabbit | Cell Signaling Tech | 1:2,000 |
p-GSK3β (Ser9) | Rabbit | Cell Signaling Tech | 1:2,000 |
p-GSK3α/β (Tyr279/Tyr216) | Rabbit | Cell Signaling Tech | 1:2,000 |
Hepcidin | Goat | Santa Cruz | 1:1,000 |
IBA1 | Rabbit | Abcam | 1:1,000 |
IL-1β | Rabbit | Santa Cruz | 1:1,000 |
IL-6 | Rabbit | Santa Cruz | 1:1,000 |
MAP2 | Mouse | Sigma-Aldrich | 1:2,000 |
Nrf2 | Rabbit | Proteintech | 1:2,000 |
NeuN | Rabbit | Cell Signaling Tech | 1:2,000 |
PSD-95 | Rabbit | Cell Signaling Tech | 1:2,000 |
P35/25 | Rabbit | Cell Signaling Tech | 1:2,000 |
PP2A | Rabbit | Cell Signaling Tech | 1:2,000 |
SOD1 | Rabbit | Cell Signaling Tech | 1:2,000 |
SYP | Mouse | Sigma-Aldrich | 1:1,000 |
Total Tau | Rabbit | Cell Signaling Tech | 1:2,000 |
p-Tau (Ser202) | Rabbit | Cell Signaling Tech | 1:2,000 |
p-Tau (Ser396) | Mouse | Sigma-Aldrich | 1:2,000 |
p-Tau (Ser404) | Rabbit | Sigma-Aldrich | 1:2,000 |
p-Tau (Thr181) | Rabbit | Cell Signaling Tech | 1:2,000 |
p-Tau (Thr231) | Rabbit | Sigma-Aldrich | 1:2,000 |
xCT | Rabbit | Abcam | 1:2,000 |
TF | Rabbit | Cell Signaling Tech | 1:2,000 |
TFR1 | Mouse | Abcam | 1:1,000 |
TNFα | Mouse | Santa Cruz | 1:1,000 |
GAPDH | Mouse | Cell Signaling Tech | 1:5,000 |
β-actin | Mouse | Sigma-Aldrich | 1:10,000 |
Real-time polymerase chain reaction
The total RNA was extracted from cerebral cortex tissues using TRIzol reagents (Invitrogen). One microgram of total RNA was reverse transcribed to cDNA using GoTaqR 2-Step RT-qPCR System (Promega) and the cDNA obtained was used for subsequent polymerase chain reaction (PCR) reactions. All PCR reactions were performed in a total volume of 10 µL: DNA polymerase activation at 95 °C for 10 min, and 40 cycles of denaturing at 95 °C for 15 s, and annealing and extension at 56 °C for 10 s. The following PCR primers were used: hepcidin: forward, TGTCTCCTGCTTCTCCTCC and reverse, ATGTCTGCCCTGCTTTCTT; glyceraldehyde-3-phosphate dehydrogenase (GAPDH): forward, GCCTTCCGTGTTCCTACC and reverse, AGAGTGGGAGTTGCTGTTG. The mRNA expression was calculated using ΔΔCt (threshold cycle, Ct) values normalized to GAPDH.
Biochemical analysis
To quantify oxidative stress indicators, the brain tissues of the mice were weighed and homogenized in
Statistics
The differences between the means were counted using Student’s t-test for in vivo. One-way ANOVA was employed for in vitro. The results are expressed as the mean ± standard error of the mean (SEM). All the data were calculated using Prism (GraphPad Software Inc.), and the differences were assumed to be statistically significant if the P < 0.05.
RESULTS
Melatonin improves the autonomous behavior and cognitive ability of P301S mice
In this study, P301S transgenic mice were employed, which have a large number of NFTs and significant neuronal degeneration[32]. The experimental flow chart is shown in Figure 1A, and HPLC-MS experiments using 8-month-old WT mice were first performed to analyze the efficiency of melatonin entry into the brain. As shown in Figure 1B, at the time point we monitored, melatonin concentration peaked 15 min after administration of melatonin.
Figure 1. Melatonin improves the autonomous behavior and cognitive ability of P301S mice. (A) The experimental flow chart; (B) Melatonin content in the brain of WT mice. n = 3; (C and D) The escape latency and path length of melatonin-treated P301S mice were reduced in the hidden platform tests of the MWM; (E) The passage times of P301S mice were increased by melatonin treatment; (F) Total movement distance of P301S mice in open field exploration; (G) The time of activity was increased in the center of the open field during OFT; (H) The movement tracks of P301S mice were shown in OFT; (I) The ability to construct nests of P301S mice was ameliorated by melatonin treatment. Data represent the mean ± SEM (n = 8). *P < 0.05. WT: Wild-type; MWM: Morris water maze; OFT: open field test; SEM: standard error of the mean.
Next, several behavioral experiments were conducted. In MWM experiments, there was no significant difference in the mean escape latency between the two groups of mice in the visual platform, indicating that melatonin treatment did not affect the motor ability and vision of the mice. During the hidden platform period, melatonin-treated mice found the platform in significantly less time and with a shorter path length than vehicle-treated mice [Figure 1C and D]. In the shuttle experiment, mice in the melatonin-treated mice crossed the original platform location significantly more times than mice in the vehicle-treated mice [Figure 1E]. The data indicated that melatonin treatment can significantly improve the learning and spatial memory ability of P301S mice. In OFT, we found that there was no significant difference in the total movement distance between the two groups of mice, indicating that melatonin treatment had no effect on the motor ability of mice; however, the time of activity in the central region of mice in the melatonin-treated mice was significantly higher than that in the vehicle-treated mice [Figure 1F-H], indicating that after melatonin treatment, the anxious behavior of P301S mice was reduced and the exploration instinct was enhanced. In addition, we executed the nest construction test to survey the effect of nasal melatonin treatment on the social behavior of the P301S mice, and the melatonin-treated mice exhibited better than the vehicle-treated mice [Figure 1I].
Melatonin improves Tau hyperphosphorylation in P301S mice through multiple pathways
To investigate whether the improvement of cognitive behavior in P301 mice by nasal administration of melatonin involves inhibition of Tau hyperphosphorylation, we detected the phosphorylation levels of Tau at serine (Ser) and threonine (Thr) sites using western blotting. The results showed that compared with the vehicle-treated mice, Tau phosphorylation levels at Ser 202, Ser 396, Ser 404, and Thr181 were significantly reduced in the melatonin-treated mice [Figure 2A-E], but no significant changes were detected at Thr231
Figure 2. Melatonin ameliorates Tau hyperphosphorylation in P301S mice via multiple pathways. (A-G) Western blotting results showing the levels of phosphorylated Tau at sites Ser202, Ser396, Ser404, Thr181, and Thr231 in the brain of melatonin/-vehicle treated P301S mice; (H-K) Immunoblotting analysis of calpain1, p-CDK5, CDK5, and P35/25 in the brains after melatonin treatment; (L-O) Immunoblotting analysis of p-GSK3β (Ser9), p-GSK3α/β (Tyr279/Tyr216), and GSK3β in the brains after melatonin treatment. β-actin served as the internal control. The values are presented as mean ± SEM (n = 8). *P < 0.05. CDK5: Cyclin-dependent kinase 5; GSK3β: glycogen synthase kinase 3β; SEM: standard error of the mean.
Next, several kinases and phosphatases associated with Tau phosphorylation were detected. As shown in Figure 2H, marked reductions in calpain 1 level and the ratios of p-CDK5/CDK5 and P25/P35 were observed in the melatonin-treated mice compared with those in the vehicle-treated mice [Figure 2H-K]. Moreover, the p-GSK3α/β (Tyr279/Tyr216)/GSK3α/β ratio in the brains of the melatonin-treated mice was significantly higher than that in the brains of vehicle-treated mice [Figure 2L and M], but the level of the p-GSK3β (Ser9)/GSK3β was not significantly decreased (Figure 2L and N, P > 0.05). In addition, quantification of the western blotting data showed that the melatonin treatment significantly increased the level of PP2A
Melatonin relieves synaptic dysfunction and neuronal damage in P301S mice
Next, we verified the neuroprotective effect of melatonin treatment on P301S mice. Compared with the vehicle-treated mice, the hippocampal CA1 region of melatonin-treated P301S mice had stronger Nissl staining, suggesting increased Nissl bodies and enhanced neuronal protein synthesis [Figure 3A]. Further examination revealed that the immunohistochemical positive staining of MAP2 (a neuronal marker) was significantly enhanced after melatonin treatment [Figure 3B]; meanwhile, the expression levels of MAP2, NeuN, synaptophysin (SYP), and PSD95 were significantly increased in the brain tissue of melatonin-treated P301S mice [Figure 3C-G].
Figure 3. Melatonin relieves synaptic dysfunction and neuronal damage in P301S mice. (A) Nissl staining indicating the functional states of neurons in the hippocampal CA1 region of melatonin- and vehicle-treated P301S mice. Scale bar = 20 μm; (B) Immunohistochemical staining results of MAP2 in the hippocampal CA1 region of P301S mice. Scale bar = 50 μm; (C-F) Immunoblotting results showing the levels of MAP2, NeuN, PSD95, and SYP in the P301S mouse brains after melatonin treatment. GAPDH served as an internal control. Values are represented as mean ± SEM (n = 8). *P < 0.05. MAP2: Microtubule-associated protein 2; PSD95: postsynaptic density protein 95; SYP: synaptophysin; GAPDH: glyceraldehyde-3-phosphate dehydrogenase; SEM: standard error of the mean.
Melatonin inhibited neuroinflammation in the brain of P301S mice
Inflammatory factors, which are secreted by microglia and astrocytes, promote excessive Tau phosphorylation. Tau hyperphosphorylation further stimulates microglia and astrocytes, causing the release of more inflammatory factors, thus forming a vicious cycle and exacerbating the pathological process of AD[33]. To verify whether melatonin inhibits inflammatory responses, we used immunohistochemistry to detect the astrocyte marker GFAP in the brains of P301S mice. As shown in Figure 4A, astrocytes in both the vehicle-treated mice and the melatonin-treated mice exhibited varying degrees of cell activation, which was manifested as cell body hypertrophy, swelling, and increased processes. However, the melatonin-treated mice had fewer protrusions and significantly reduced aggregation of astrocytes, indicating that the activation of astrocytes in the P301S mouse brain was inhibited after melatonin treatment. Moreover, we also examined the expression distribution of microglial marker IBA1 in the two groups of mice. As shown in Figure 4B, compared with the vehicle-treated mice, the number and activation degree of IBA1 stained positive cells in the hippocampal CA1 region of mice in the melatonin-treated mice were significantly reduced, indicating that the excessive activation of microglia in the P301S mouse brain was also inhibited after melatonin treatment. In addition, we found that compared with the vehicle-treated mice, melatonin treatment significantly downregulated the expression levels of GFAP, IBA1, tumor necrosis factor α
Figure 4. Melatonin inhibited neuroinflammation in P301S mouse brains. (A and B) Immunohistochemical staining results of GFAP and IBA1 in the hippocampal CA1 region of P301S mice. Scale bar = 50 μm; (C-H) Western blotting analysis showing the protein expression levels of GFAP, IBA1, IL-1β, IL-6, and TNFα in the melatonin-treated P301S mice. β-actin served as the internal control. Values are represented as the means ± SEM (n = 8). *P < 0.05. GFAP: Glial fibrillary acidic protein; IBA1: ionized calcium-binding adaptor molecule 1; IL-1β: interleukin-1β; SEM: standard error of the mean.
Melatonin retards the iron deposition process in the brain of P301S mouse brain
The inhibition of Tau pathology in AD by iron chelators has been confirmed[11,13]. Based on the iron chelation ability of melatonin[34], we next examined the iron deposition using Perl’s staining in the brain of P301S mice. As shown in Figure 5A, there were brown particles in the iron-stained positive neurons in the hippocampal CA1 region of P301S mice, while the number of iron-stained positive cells in the melatonin-treated mice was reduced compared to the vehicle-treated mice. We examined the effects of melatonin on iron metabolism-related proteins in the brains of P301S mice. The results showed that ferritin heavy chain (FTH) expression was significantly decreased after melatonin treatment [Figure 5B and C], further indicating that melatonin treatment can significantly reduce the iron content in the brain of P301S mice. Moreover, the expression levels of ferroportin (FPN) were clearly upregulated [Figure 5D], and the expression levels of transferrin (TF) and transferrin receptor 1 (TFR1) were significantly downregulated
Figure 5. Melatonin retards the iron deposition process in the brain of P301S mouse brain. (A) Perl’s DAB staining of the hippocampal CA1 region in P301S mice. Scale bar = 50 μm; (B-G) Western blotting analysis and quantification of DMT1, FPN, TFR, TF, and FTH with β-actin as an internal control. Values are represented as the means ± SEM (n = 8). *P < 0.05. DAB: 3,3,-Diaminobenzidine tetrahydrochloride; DMT1: divalent metal transporter 1; FPN: ferroportin; TFR: transferrin receptor; TF: transferrin; FTH: ferritin heavy chain; SEM: standard error of the mean.
Melatonin increases hepcidin expression in the brain of P301S mice
Given the prominent role of hepcidin iron regulation, fluorescence quantitative PCR and western blotting techniques were utilized to detect the expression levels of hepcidin mRNA and protein in the P301S mouse brain. We found that compared with the vehicle-treated mice, the mRNA and protein levels of hepcidin in the brain of P301S mice dramatically increased after melatonin treatment [Figure 6A-C]. Immunohistochemical staining results showed that the cortex and hippocampus had brown-yellow positive staining in both groups of mice. Compared with the vehicle-treated mice, the positive staining intensity in the cortex was stronger in the melatonin-treated mice, while the change in the hippocampus was not obvious [Figure 6D]. These results suggest that the changes in iron metabolism in the brain of P301S transgenic mice after melatonin treatment may be regulated by in-situ synthesis of hepcidin in the brain.
Figure 6. Melatonin increases hepcidin expression in the brain of P301S mice. (A) Immunohistochemical staining results of hepcidin in the cortex and hippocampus of P301S mice. Scale bar = 50 μm; (B and C) Western blotting analysis and quantification of hepcidin with
Melatonin inhibition of iron-induced Tau hyperphosphorylation in HT22 cells involves hepcidin secreted by D1A cells
To determine which cells in the brain melatonin can stimulate upregulation of hepcidin expression, we conducted a series of cell experiments. HT22 cells were treated with FAC to induce Tau hyperphosphorylation in hippocampal neurons similar to P301S mice and to evaluate whether melatonin can regulate iron metabolism in the cells. As expected, melatonin treatment significantly inhibited FAC-induced Tau hyperphosphorylation at Ser 202, Ser 396, Ser 404, and Thr181 [Figure 7A-E]. Further detection revealed that melatonin significantly inhibited the expression of hepcidin in HT22 cells, while promoting the expression of FPN; however, hepcidin expression was significantly upregulated in HT22 cells treated with FAC, while FPN expression was only weakly downregulated. Compared with HT22 cells treated with FAC alone, hepcidin was downregulated when melatonin and FAC were co-treated, and FPN still showed an upregulated trend [Figure 7F-H]. These results indicate that melatonin treatment can directly affect the iron metabolism of HT22 cells, but its effect is diminished in cells where Tau hyperphosphorylation has been induced by FAC. In other words, the increase of hepcidin in the brain of P301S mice had little to do with neurons.
Figure 7. Melatonin inhibition of iron-induced Tau hyperphosphorylation in HT22 cells involves hepcidin secreted by D1A cells. (A-E) HT22 cells were untreated or treated with 50 μM melatonin, 100 μM FAC, 50 μM melatonin + 100 μM FAC for 24 h. Cell lysates were then collected from these cells and subjected to western blotting analysis to evaluate Tau hyperphosphorylation at Ser202, Ser396, Ser404, and Thr181 using their antibodies; (F-H) The expression levels of hepcidin and FPN were tested by immunoblotting in HT22 cells; (I-K) The expression levels of hepcidin and FPN were shown in D1A cells after melatonin and/or FAC treatment; (L-N) The HT22 cells, which were treated respectively with melatonin- and/or FAC-treated D1A cells media, were subjected to western blotting analysis using hepcidin, FPN, GPX4, p-Tau (Ser202), p-Tau (Ser396), p-Tau (Thr181), and T-Tau antibodies. β-actin was used as an internal control. Values are represented as the means ± SEM (n = 3). *P < 0.05 compared with the Control group; #P < 0.05 compared with the FAC group. FAC: Ferric ammonium citrate; FPN: ferroportin; GPX4: glutathione peroxidase 4; SEM: standard error of the mean.
In fact, in addition to neurons, hepcidin immune responses were also detected in GFAP-positive glial cells[35]. To investigate whether the upregulated hepcidin in the brain of melatonin-treated mice might originate from astrocytes, we examined the expression levels of hepcidin and FPN in D1A cells treated with FAC and/or melatonin, and HT22 cells treated with D1A cell medium. In D1A cells, compared with FAC alone, the expression level of hepcidin was significantly increased after FAC and melatonin combined culture, while the expression level of FPN was significantly decreased [Figure 7I-K]. These results indicated that melatonin treatment stimulated hepcidin expression in FAC-stressed astrocytes.
Further detection of HT22 cells cocultured with D1A showed that the expression level of hepcidin in HT22 cells in D1A medium treated with FAC and melatonin was slightly downregulated compared with that in D1A medium treated with FAC only, but the level of FPN was significantly increased [Figure 7L-N]. Interestingly, the D1A medium treated with medium and FAC significantly upregulated the levels of GPX4 but had no significant effect on Tau phosphorylation at Ser202, Ser396, and Thr181 [Figure 7O-S]. These results indicate that under an iron overload environment, astrocyte products after melatonin treatment can stimulate the efflux of iron ions from neurons, thereby inhibiting oxidative damage and ferroptosis in neurons. *P < 0.05.
Melatonin alleviates oxidative stress response and ferroptosis in the brain of P301S mice
Melatonin has been shown to rescue ferroptosis in various cellular and animal models of noncancerous diseases[26]. To investigate whether melatonin can inhibit Tau-induced ferroptosis by acting as ROS scavengers or metal chelators, we first measured the expression of GPX4 in the brain tissue of P301S mice. Immunofluorescence double labeling results showed that nasal administration of melatonin clearly enhanced the positive signal of GPX4 while reducing p-Tau (Ser396) expression [Figure 8A and B]. Then, we demonstrated that the protein expression levels of superoxide dismutase 1 (SOD1), GPX4, Nrf2, and xCT statistically increased in the brain of P301S mice after melatonin treatment [Figure 8B-G]. Accordingly, the levels of ROS and MDA in the brain of P301S mice were significantly decreased, while the content of GSH was increased after melatonin treatment [Figure 8H-J], indicating that melatonin can effectively relieve the oxidative stress caused by iron overload in the brain of P301S mice, and thus reduce the occurrence of ferroptosis.
Figure 8. Melatonin alleviates oxidative stress response and ferroptosis in P301S mouse brains. (A and B) Immunofluorescence labeling and confocal microscopy analysis showing the expression of GPX4 (red) and p-Tau (Ser396) (green) in the cortex and hippocampus of the P301S mouse brains. DAPI was used to detect the nuclei (blue). Scale bar = 25 μm; (C-G) Western blotting analysis showing that the protein expression levels of SOD1, GPX4, Nrf2, and xCT were significantly increased in melatonin-treated mice. β-actin served as the internal control; (H-J) GSH, ROS, and MDA production levels in the brains of P301S mice, as detected by the DCFH-DA method. Values are represented as mean ± SEM (n = 8). *P < 0.05. GPX4: Glutathione peroxidase 4; DAPI: 4’,6-diamidino-2-phenylindole; SOD1: superoxide dismutase 1; Nrf2: nuclear factor erythroid 2-related factor 2; xCT: cystine-glutamate antiporter; GSH: gamma-glutamyl-cysteinyl-glycine; ROS: reactive oxygen species; MDA: malondialdehyde; DCFH-DA: 2’,7’-dichlorofluorescein diacetate; SEM: standard error of the mean.
DISCUSSION
Growing evidence has confirmed that melatonin has a powerful neuroprotective effect and therapeutic potential for neurodegenerative diseases[16-25]. In this study, we confirmed that long-term treatment with melatonin can effectively alleviate behavioral deficits in P301S transgenic mice using nasal administration, which involves reducing Tau hyperphosphorylation, iron deposition and related oxidative stress, and ferroptosis.
The brain of P301S transgenic mice exhibits characteristics such as NFTs, synaptic dysfunction, and neuronal damage, which have been widely used in the study of neurodegenerative diseases such as AD[36]. Previous studies found that compared with WT mice, the brain of 8-month-old P301S transgenic mice showed relevant indications of increased iron content and ferroptosis[13]. Although it has been reported that melatonin inhibits Tau hyperphosphorylation, systematic studies have been lacking, and whether its neuroprotective mechanism is involved in inhibiting ferroptosis remains to be clarified.
As expected, the results of ICP-MS and behavioral experiments confirmed that intranasal administration resulted in a peak of melatonin concentration in the brain and effectively improved cognitive behavioral deficits in P301S mice. As evidenced by previous cell line experiments and animal studies[16,37-39], intranasal administration of melatonin also exhibits promise in mitigating Tau hyperphosphorylation at multiple sites in the brain of P301S mice. In tauopathies of AD, hyperphosphorylated Tau is related to various protein kinases and protein phosphatases, especially CDK5, GSK3β, and PP2A[40]. In fact, various mechanisms by which melatonin inhibits tauopathies (such as the inhibition of CDK5[23] and GSK3β[19], and the activation of PP2A[38,41]) have been reported. Here, we systematically examined this with the P301S Tg mice, and found that the expression levels of p-CDK5 and P25 were significantly reduced after melatonin treatment, indicating that CDK5 activity was inhibited[42]. Meanwhile, it was found that the phosphorylation level of GSK3α/β at Ser 21/9 did not change significantly, but its phosphorylation level at Tyr 279/216 was significantly reduced, indicating that melatonin inhibited the activity of GSK3β by inhibiting the autophosphorylation of Tyr 279/216[43]. In addition, compared with the vehicle-treated mice, the expression level of PP2A in the brain of melatonin-treated mice was significantly upregulated, suggesting that melatonin promoted the expression of PP2A, which may catalyze the Tau dephosphorylation[40].
Growing evidence suggests that synaptic dysfunction and neuronal loss are key pathological features of AD, and that pathological Tau dissociation from microtubules and mislocalization into presynaptic and postsynaptic neuronal compartments induce synaptic dysfunction in multiple ways[44]. In addition to the hyperphosphorylated Tau and tangle development in the brain of P301S mice, it also showed obvious synaptic dysfunction and neuron loss in the CA1 region of the hippocampus[32]. Melatonin has been found to significantly reverse D-galactose-induced synaptic dysfunction by increasing levels of memory-related presynaptic and postsynaptic protein markers[45] and restoring induced neuronal death by kainic acid[46] and prion protein[47]. Melatonin pretreatment can also significantly reduce the dendritic length and complexity of primary neurons, and decrease synaptic protein and neuronal death caused by acidosis[48]. Consistently, we found that after nasal administration of melatonin, the expression levels of neuronal dendrite marker MAP2, neuronal marker NeuN, presynaptic marker protein SYP, and postsynaptic marker PSD95 were significantly increased, and the intensity of Nissl staining was also enhanced, indicating that melatonin treatment could effectively improve synaptic plasticity and neuronal function in P301S Tg mice.
Although the mechanism of neuronal dysfunction remains unclear, tauopathy has also been linked to ferroptosis[13,49]. The main characteristic of ferroptosis is the accumulation of a large amount of iron and lipid peroxidation during the process of cell death. Its inducing factors can directly or indirectly inhibit GPX4, leading to a decrease in cellular antioxidant capacity and ROS accumulation, ultimately resulting in ferroptosis[50]. Previous studies have shown the phenomenon of increased oxidative stress[51], and we have also confirmed the presence of lipid peroxidation and elevated iron content in the brain of P301S transgenic mice[13]. Given the strong iron chelating ability[34] and free radical scavenging ability[52], we speculated that melatonin may inhibit neuronal oxidative stress and ferroptosis, thereby exerting a certain neuroprotective role. In fact, melatonin has been shown to rescue ferroptosis in various cellular and animal models of noncancerous diseases[26]. In our system, attenuated Perl’s staining intensity and reduced ferritin confirmed decreased iron deposition in the hippocampal CA1 region of melatonin-treated mice. Moreover, reduced TFR1 and increased FPN supported that melatonin may reduce the iron deposition in the brain of P301S mice by reducing cellular iron input and increasing cellular iron efflux[53]. Besides protecting hippocampal neurons by affecting iron metabolism, we found that melatonin treatment in P301S mice also reduced the production of ROS and MDA, and increased the expression of SOD1, GPX4, xCT, and Nrf2, which plays an important role in oxidative stress and ferroptosis balance[26,52]. Meanwhile, the content of GSH also increased significantly after melatonin administration, indicating that melatonin treatment can significantly improve the oxidative stress in the brain of P301S mice and inhibit the occurrence of ferroptosis[26]. Interestingly, previous studies have confirmed that melatonin has powerful anti-inflammatory properties in addition to its antioxidant and iron-chelating functions[54]. A study found that ferroptosis is implicated in inflammation in hepatocytes[55]. In this study, we detected that the expression levels of GFAP, IBA1, TNF-α, and IL-6 were significantly reduced in the melatonin treatment mice, and the protrusion and aggregation degree of astrocytes in the cortex and hippocampus were also significantly decreased, indicating that the inflammatory response of P301S mice treated with melatonin was inhibited. These results suggest that the neuroprotection of melatonin can be achieved either by directly inhibiting neuroinflammation or by reducing the release of inflammatory factors induced by ferroptosis[56]. These studies suggest that melatonin may play an anti-AD role by inhibiting ferroptosis in P301S Tg mice.
It is well-known that hepcidin reduces iron load by degrading FPN, but the role of hepcidin in regulating brain iron homeostasis is still controversial[57]. It has been found that hepcidin can contribute to iron accumulation in AD brain tissue[58]. Other studies have shown that hepcidin in the brain of AD is reduced[59,60], and when hepcidin is supplemented externally or overexpressed in the brain, the iron content and iron-induced oxidative stress in the mouse brain can be significantly reduced[61,62]. Here, we found that melatonin treatment can significantly increase the mRNA and protein expression of hepcidin in the brain of P301S Tg mice. However, immunohistochemical staining results showed a significant increase in hepcidin-positive signals in cortical cells of melatonin-treated mice, while there was no significant change in the hippocampus. Similarly, in vitro, we did not find that melatonin could increase the expression of hepcidin in hippocampal neurons of mice exposed to high iron, and even showed a downward trend. Therefore, we made a reasonable conjecture that melatonin-induced upregulation of hepcidin in the P301S mouse brain does not occur in neurons, but in other cells, such as astrocytes. In fact, it has been thought that in addition to liver cells, various types of cells in the brain can also synthesize hepcidin in situ, especially glial cells[60]. It has been found that astrocyte-derived hepcidin may interact with FPN of brain microvascular endothelial cells (BMVECs)[63], thereby regulating brain iron uptake[64]. Moreover, astrocytes respond to intracellular high iron levels and subsequently increase the secretion of hepcidin, thereby regulating total brain iron levels through the hepcidin/FPN pathway[63]. Interestingly, we found that although melatonin did not directly stimulate the expression changes of hepcidin in D1A cells, it could significantly reverse the decline in hepcidin induced by FAC and inhibit the upregulation of FPN caused by FAC, suggesting that melatonin treatment increased the expression of hepcidin in astrocytes but not neurons under iron overload environment. The increase in hepcidin in astrocytes may further mediate the endocytosis and degradation of FPN, thereby reducing the release of iron ions from BMVECs, and ultimately rescuing neurons from damage[56]. In addition, we found that melatonin directly stimulated the expression of FPN in HT22 cells, without causing significant upregulation of FPN in D1A cells. When HT22 cells were cocultured with D1A, both melatonin and FAC treatments significantly upregulated the expression of hepcidin and FPN, and the combination of the two treatments not only failed to significantly reduce hepcidin, but also significantly increased FPN. These results suggest that melatonin stimulates astrocytes to produce certain metabolites, which have a more significant impact on the expression of FPN in neurons. Of course, the current results also make us rethink the relationship between hepcidin and FPN in the AD model of mouse brain.
The limitations of this study should be considered when interpreting the results. It is important to address that the sample size of mice may limit the generalizability of the data. It would have been more interesting and important for conclusion if we had performed co-staining hepcidin and FPN with neuronal and astrocytic markers in vivo. In addition, the role of microglia and hepatic hepcidin in our experimental system may need to be explored or excluded. Of course, future studies should aim to highlight the exact mechanism by which astrocytes rescue neuronal tauopathy and ferroptosis after melatonin treatment.
In conclusion, our study demonstrates that nasal administration of melatonin can exert neuroprotective effects through its powerful free radical scavenging ability, anti-inflammatory and antioxidant activities, and iron chelation ability, thereby inhibiting Tau hyperphosphorylation and ferroptosis in P301S transgenic mice. This systematic analysis of melatonin is expected to offer vital evidence and a target for the prevention and treatment of tauopathy.
DECLARATIONS
Authors’ contributions
Performed most of the data acquisition and wrote the initial manuscript: Jia MY
Contributed to data acquisition: Wang D, Liang XT
Generated the mouse model and validation: Guo SQ, Guo SJ
Edited the manuscript: Liu X
Provided material support and supervision: Wang T, Zhong ML, Gao HL
Conceived the study and edited the manuscript: Guo C
All authors have read and approved the final manuscript.
Availability of data and materials
The data will not be shared because there are further plans, and requests for materials should be addressed to Guo C.
Financial support and sponsorship
The study was supported by the National Natural Science Foundation of China (No. 32371037, 31970967), and Key Laboratory of Bioresource Research and Development of Liaoning Province (2022JH13/10200026).
Conflicts of Interest
All authors declared that there are no conflicts of interest.
Ethical approval and consent to participate
All the animal experimental procedures in this study were approved by the experimental animal ethics committee of Northeastern University (NEU-EC-2023A014S).
Consent for publication
Not applicable.
Copyright
© The Author(s) 2025.
REFERENCES
1. Lee VM, Goedert M, Trojanowski JQ. Neurodegenerative tauopathies. Annu Rev Neurosci. 2001;24:1121-59.
3. Iqbal K, Liu F, Gong CX, Grundke-Iqbal I. Tau in Alzheimer disease and related tauopathies. Curr Alzheimer Res. 2010;7:656-64.
4. van der Kant R, Goldstein LSB, Ossenkoppele R. Amyloid-β-independent regulators of tau pathology in Alzheimer disease. Nat Rev Neurosci. 2020;21:21-35.
5. Congdon EE, Ji C, Tetlow AM, Jiang Y, Sigurdsson EM. Tau-targeting therapies for Alzheimer disease: current status and future directions. Nat Rev Neurol. 2023;19:715-36.
6. Huat TJ, Camats-Perna J, Newcombe EA, Valmas N, Kitazawa M, Medeiros R. Metal toxicity links to Alzheime’s disease and neuroinflammation. J Mol Biol. 2019;431:1843-68.
7. Singh N, Haldar S, Tripathi AK, et al. Brain iron homeostasis: from molecular mechanisms to clinical significance and therapeutic opportunities. Antioxid Redox Signal. 2014;20:1324-63.
8. Li LB, Chai R, Zhang S, et al. Iron exposure and the cellular mechanisms linked to neuron degeneration in adult mice. Cells. 2019;8:198.
9. Guo C, Wang T, Zheng W, Shan ZY, Teng WP, Wang ZY. Intranasal deferoxamine reverses iron-induced memory deficits and inhibits amyloidogenic APP processing in a transgenic mouse model of Alzheimer’s disease. Neurobiol Aging. 2013;34:562-75.
10. Gleason A, Bush AI. Iron and ferroptosis as therapeutic targets in Alzheimer’s disease. Neurotherapeutics. 2021;18:252-64.
11. Guo C, Wang P, Zhong ML, et al. Deferoxamine inhibits iron induced hippocampal tau phosphorylation in the Alzheimer transgenic mouse brain. Neurochem Int. 2013;62:165-72.
12. Guo C, Yang ZH, Zhang S, et al. Intranasal lactoferrin enhances α-secretase-dependent amyloid precursor protein processing via the ERK1/2-CREB and HIF-1α pathways in an Alzheimer’s disease mouse model. Neuropsychopharmacology. 2017;42:2504-15.
13. Zhang YH, Wang DW, Xu SF, et al. α-Lipoic acid improves abnormal behavior by mitigation of oxidative stress, inflammation, ferroptosis, and tauopathy in P301S Tau transgenic mice. Redox Biol. 2018;14:535-48.
14. Wei M, Wu T, Chen N. Bridging neurotrophic factors and bioactive peptides to Alzheimer’s disease. Ageing Res Rev. 2024;94:102177.
15. Zhang Z, Xue P, Bendlin BB, et al. Melatonin: a potential nighttime guardian against Alzheimer’s. Mol Psychiatry. 2025;30:237-50.
16. Ali T, Kim MO. Melatonin ameliorates amyloid beta-induced memory deficits, tau hyperphosphorylation and neurodegeneration via PI3/Akt/GSk3β pathway in the mouse hippocampus. J Pineal Res. 2015;59:47-59.
17. Li Y, Zhang J, Wan J, Liu A, Sun J. Melatonin regulates Aβ production/clearance balance and Aβ neurotoxicity: a potential therapeutic molecule for Alzheimer’s disease. Biomed Pharmacother. 2020;132:110887.
18. Shukla M, Govitrapong P, Boontem P, Reiter RJ, Satayavivad J. Mechanisms of melatonin in alleviating Alzheimer’s disease. Curr Neuropharmacol. 2017;15:1010-31.
19. Das R, Balmik AA, Chinnathambi S. Melatonin reduces GSK3β-mediated tau phosphorylation, enhances Nrf2 nuclear translocation and anti-inflammation. ASN Neuro. 2020;12:1759091420981204.
20. Balmik AA, Das R, Dangi A, Gorantla NV, Marelli UK, Chinnathambi S. Melatonin interacts with repeat domain of Tau to mediate disaggregation of paired helical filaments. Biochim Biophys Acta Gen Subj. 2020;1864:129467.
21. Chen C, Yang C, Wang J, et al. Melatonin ameliorates cognitive deficits through improving mitophagy in a mouse model of Alzheimer’s disease. J Pineal Res. 2021;71:e12774.
22. Luengo E, Buendia I, Fernández-Mendívil C, et al. Pharmacological doses of melatonin impede cognitive decline in tau-related Alzheimer models, once tauopathy is initiated, by restoring the autophagic flux. J Pineal Res. 2019;67:e12578.
23. Chen D, Lan G, Li R, et al. Melatonin ameliorates tau-related pathology via the miR-504-3p and CDK5 axis in Alzheimer’s disease. Transl Neurodegener. 2022;11:27.
24. Li LB, Fan YG, Wu WX, et al. Novel melatonin-trientine conjugate as potential therapeutic agents for Alzheimer’s disease. Bioorg Chem. 2022;128:106100.
25. Roy J, Wong KY, Aquili L, et al. Role of melatonin in Alzheimer’s disease: from preclinical studies to novel melatonin-based therapies. Front Neuroendocrinol. 2022;65:100986.
26. Zhang D, Jia X, Lin D, Ma J. Melatonin and ferroptosis: mechanisms and therapeutic implications. Biochem Pharmacol. 2023;218:115909.
27. Ma H, Wang X, Zhang W, et al. Melatonin suppresses ferroptosis induced by high glucose via activation of the Nrf2/HO-1 signaling pathway in type 2 diabetic osteoporosis. Oxid Med Cell Longev. 2020;2020:9067610.
28. Li M, Yang N, Hao L, et al. Melatonin inhibits the ferroptosis pathway in rat bone marrow mesenchymal stem cells by activating the PI3K/AKT/mTOR signaling axis to attenuate steroid-induced osteoporosis. Oxid Med Cell Longev. 2022;2022:8223737.
29. Qiu W, An S, Wang T, et al. Melatonin suppresses ferroptosis via activation of the Nrf2/HO-1 signaling pathway in the mouse model of sepsis-induced acute kidney injury. Int Immunopharmacol. 2022;112:109162.
30. Manchester LC, Coto-Montes A, Boga JA, et al. Melatonin: an ancient molecule that makes oxygen metabolically tolerable. J Pineal Res. 2015;59:403-19.
31. Yang YY, Ren YT, Jia MY, et al. The human islet amyloid polypeptide reduces hippocampal tauopathy and behavioral impairments in P301S mice without inducing neurotoxicity or seeding amyloid aggregation. Exp Neurol. 2023;362:114346.
32. Yoshiyama Y, Higuchi M, Zhang B, et al. Synapse loss and microglial activation precede tangles in a P301S tauopathy mouse model. Neuron. 2007;53:337-51.
33. Chen Y, Yu Y. Tau and neuroinflammation in Alzheimer’s disease: interplay mechanisms and clinical translation. J Neuroinflammation. 2023;20:165.
34. Gulcin I, Buyukokuroglu ME, Kufrevioglu OI. Metal chelating and hydrogen peroxide scavenging effects of melatonin. J Pineal Res. 2003;34:278-81.
35. Zechel S, Huber-Wittmer K, von Bohlen und Halbach O. Distribution of the iron-regulating protein hepcidin in the murine central nervous system. J Neurosci Res. 2006;84:790-800.
36. Xu H, Rösler TW, Carlsson T, et al. Memory deficits correlate with tau and spine pathology in P301S MAPT transgenic mice. Neuropathol Appl Neurobiol. 2014;40:833-43.
37. Wang DL, Ling ZQ, Cao FY, Zhu LQ, Wang JZ. Melatonin attenuates isoproterenol-induced protein kinase A overactivation and tau hyperphosphorylation in rat brain. J Pineal Res. 2004;37:11-6.
38. Li XC, Wang ZF, Zhang JX, Wang Q, Wang JZ. Effect of melatonin on calyculin A-induced tau hyperphosphorylation. Eur J Pharmacol. 2005;510:25-30.
39. Shi C, Zeng J, Li Z, et al. Melatonin mitigates kainic acid-induced neuronal tau hyperphosphorylation and memory deficits through alleviating ER stress. Front Mol Neurosci. 2018;11:5.
40. Martin L, Latypova X, Wilson CM, Magnaudeix A, Perrin ML, Terro F. Tau protein phosphatases in Alzheimer’s disease: the leading role of PP2A. Ageing Res Rev. 2013;12:39-49.
41. Zhu LQ, Wang SH, Ling ZQ, Wang DL, Wang JZ. Effect of inhibiting melatonin biosynthesis on spatial memory retention and tau phosphorylation in rat. J Pineal Res. 2004;37:71-7.
42. Liu SL, Wang C, Jiang T, Tan L, Xing A, Yu JT. The role of Cdk5 in Alzheimer’s disease. Mol Neurobiol. 2016;53:4328-42.
43. Ly PT, Wu Y, Zou H, et al. Inhibition of GSK3β-mediated BACE1 expression reduces Alzheimer-associated phenotypes. J Clin Invest. 2013;123:224-35.
44. Wu M, Zhang M, Yin X, et al. The role of pathological tau in synaptic dysfunction in Alzheimer’s diseases. Transl Neurodegener. 2021;10:45.
45. Ali T, Badshah H, Kim TH, Kim MO. Melatonin attenuates D-galactose-induced memory impairment, neuroinflammation and neurodegeneration via RAGE/NF-K B/JNK signaling pathway in aging mouse model. J Pineal Res. 2015;58:71-85.
46. Chang CF, Huang HJ, Lee HC, Hung KC, Wu RT, Lin AM. Melatonin attenuates kainic acid-induced neurotoxicity in mouse hippocampus via inhibition of autophagy and α-synuclein aggregation. J Pineal Res. 2012;52:312-21.
47. Jeong JK, Lee JH, Moon JH, Lee YJ, Park SY. Melatonin-mediated β-catenin activation protects neuron cells against prion protein-induced neurotoxicity. J Pineal Res. 2014;57:427-34.
48. Shi Y, Cai EL, Yang C, et al. Protection of melatonin against acidosis-induced neuronal injuries. J Cell Mol Med. 2020;24:6928-42.
49. Wang J, Fu J, Zhao Y, Liu Q, Yan X, Su J. Iron and targeted iron therapy in Alzheimer’s disease. Int J Mol Sci. 2023;24:16353.
50. Lane DJR, Alves F, Ayton SJ, Bush AI. Striking a NRF2: the rusty and rancid vulnerabilities toward ferroptosis in Alzheimer’s disease. Antioxid Redox Signal. 2023;39:141-61.
51. Dumont M, Stack C, Elipenahli C, et al. Behavioral deficit, oxidative stress, and mitochondrial dysfunction precede tau pathology in P301S transgenic mice. FASEB J. 2011;25:4063-72.
52. Galano A, Reiter RJ. Melatonin and its metabolites vs oxidative stress: from individual actions to collective protection. J Pineal Res. 2018;65:e12514.
53. Liu JL, Fan YG, Yang ZS, Wang ZY, Guo C. Iron and Alzheimer’s disease: from pathogenesis to therapeutic implications. Front Neurosci. 2018;12:632.
54. Joseph TT, Schuch V, Hossack DJ, Chakraborty R, Johnson EL. Melatonin: the placental antioxidant and anti-inflammatory. Front Immunol. 2024;15:1339304.
55. Tsurusaki S, Tsuchiya Y, Koumura T, et al. Hepatic ferroptosis plays an important role as the trigger for initiating inflammation in nonalcoholic steatohepatitis. Cell Death Dis. 2019;10:449.
56. Zhang X, Gou YJ, Zhang Y, et al. Hepcidin overexpression in astrocytes alters brain iron metabolism and protects against amyloid-β induced brain damage in mice. Cell Death Discov. 2020;6:113.
57. Wang F, Wang J, Shen Y, Li H, Rausch WD, Huang X. Iron dyshomeostasis and ferroptosis: a new Alzheimer’s disease hypothesis?. Front Aging Neurosci. 2022;14:830569.
58. Chaudhary S, Ashok A, McDonald D, et al. Upregulation of local hepcidin contributes to iron accumulation in Alzheimer’s disease brains. J Alzheimers Dis. 2021;82:1487-97.
59. Raha AA, Vaishnav RA, Friedland RP, Bomford A, Raha-Chowdhury R. The systemic iron-regulatory proteins hepcidin and ferroportin are reduced in the brain in Alzheimer’s disease. Acta Neuropathol Commun. 2013;1:55.
60. Vela D. Hepcidin, an emerging and important player in brain iron homeostasis. J Transl Med. 2018;16:25.
61. Gong J, Du F, Qian ZM, et al. Pre-treatment of rats with ad-hepcidin prevents iron-induced oxidative stress in the brain. Free Radic Biol Med. 2016;90:126-32.
62. Du F, Qian ZM, Luo Q, Yung WH, Ke Y. Hepcidin suppresses brain iron accumulation by downregulating iron transport proteins in iron-overloaded rats. Mol Neurobiol. 2015;52:101-14.
63. You L, Yu PP, Dong T, et al. Astrocyte-derived hepcidin controls iron traffic at the blood-brain-barrier via regulating ferroportin 1 of microvascular endothelial cells. Cell Death Dis. 2022;13:667.
Cite This Article

How to Cite
Download Citation
Export Citation File:
Type of Import
Tips on Downloading Citation
Citation Manager File Format
Type of Import
Direct Import: When the Direct Import option is selected (the default state), a dialogue box will give you the option to Save or Open the downloaded citation data. Choosing Open will either launch your citation manager or give you a choice of applications with which to use the metadata. The Save option saves the file locally for later use.
Indirect Import: When the Indirect Import option is selected, the metadata is displayed and may be copied and pasted as needed.
About This Article
Special Issue
Copyright
Data & Comments
Data
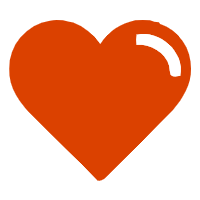
Comments
Comments must be written in English. Spam, offensive content, impersonation, and private information will not be permitted. If any comment is reported and identified as inappropriate content by OAE staff, the comment will be removed without notice. If you have any queries or need any help, please contact us at [email protected].