Supplementation with a cranberry extract favors the establishment of butyrogenic guilds in the human fermentation SHIME system
Abstract
Background: Proanthocyanidins (PAC) and oligosaccharides from cranberry exhibit multiple bioactive health properties and persist intact in the colon post-ingestion. They display a complex bidirectional interaction with the microbiome, which varies based on both time and specific regions of the gut; the nature of this interaction remains inadequately understood. Therefore, we aimed to investigate the impact of cranberry extract on gut microbiota ecology and function.
Methods: We studied the effect of a cranberry extract on six healthy participants over a two-week supplementation period using the ex vivo artificial fermentation system TWIN-M-SHIME to replicate luminal and mucosal niches of the ascending and transverse colon.
Results: Our findings revealed a significant influence of cranberry extract supplementation on the gut microbiota ecology under ex vivo conditions, leading to a considerable change in bacterial metabolism. Specifically, Bifidobacterium adolescentis (B. adolescentis) flourished in the mucus of the ascending colon, accompanied by a reduced adhesion of Proteobacteria. The overall bacterial metabolism shifted from acetate to propionate and, notably, butyrate production following PAC supplementation. Although there were variations in microbiota modulation among the six donors, the butyrogenic effect induced by the supplementation remained consistent across all individuals. This metabolic shift was associated with a rise in the relative abundance of several short-chain fatty acid (SCFA)-producing bacterial genera and the formation of a consortium of key butyrogenic bacteria in the mucus of the transverse colon.
Conclusions: These observations suggest that cranberry extract supplementation has the potential to modulate the gut microbiota in a manner that may promote overall gut health.
Keywords
INTRODUCTION
The American cranberry (Vaccinium macrocarpon) is a fruit containing various bioactive components, particularly known for being a major source of proanthocyanidins (PAC), which are oligomers and polymers of flavan-3-ols. Many in vitro, preclinical, and clinical studies have shown that PAC offers health advantages beyond merely guarding against urinary tract infections. Studies have demonstrated anti-inflammatory, cardioprotective, neuroprotective, immunomodulatory, lipid-lowering and anti-obesity, antidiabetic, and anticancer properties[1-6]. However, the exact mechanisms by which PAC delivers these health benefits are still not fully understood[2]. Increasing evidence suggests a link between the health benefits of PAC and the gut microbiota. Indeed, due to their high degree of polymerization and complex structures, 95% of PAC are not absorbed in the small intestine, are poorly metabolized in the small intestine and reach the colon intact, where they can interact with the microbiota[7-9].
Furthermore, recent research has revealed that cranberries are rich in potentially bioactive oligosaccharides. In fact, it has been suggested that (poly)phenol-rich extracts on the market could actually contain approximately 20% (w/w) oligosaccharides, primarily arabinoxyloglucans and pectic oligosaccharides[10]. These complex carbohydrates can withstand gastric digestion and bind to (poly)phenols, favoring their transport to the colon for subsequent utilization by the gut microbiota[10,11].
Recent studies have emphasized the capacity of (poly)phenols to impact the composition and functionality of the gut microbiota[8,12,13]. Our group has recently proposed that (poly)phenols exhibit a “duplibiotic” action. This refers to their capacity to alter the composition of the intestinal microbiota by exhibiting antimicrobial properties against specific pathobionts, while also having a prebiotic effect through their metabolism by the microbiota[14]. However, this bidirectional interaction has not been fully described, and the way in which PAC/oligosaccharides influence the gut microbiota remains inadequately characterized. We are just at the initial stages of identifying the commensal bacterial species responsible for PAC catabolism and understanding their role in (poly)phenol metabolism[8].
Recent studies have begun to explore the ways in which PAC might influence the composition of the gut microbiota and its potential effects on the health of the host. This includes in vitro and in vivo studies, as well as some clinical trials involving PAC supplementations from different sources (e.g., grape[15], aronia berry[16], blueberry[17], avocado[18]). These studies have consistently observed a stimulation of bifidobacteria and lactobacilli[19-22]. Moreover, Roseburia species and Faecalibacterium praustnitzii seem to flourish after PAC supplementation, while the response of Enterobacteriaceae is not consistent across studies[19,22]. PAC also favorably affect the bacteria Akkermansia muciniphila[19-22]. This bacterium is considered a next-generation probiotic and prefers the intestinal mucosal environment, where it can positively impact immunoprotection through stimulation of mucus turnover and enhancement of gut barrier function[17,23-25]. There is increasing interest in the microbiota associated with the intestinal mucus layer, as this ecological niche is essential for preventing direct contact between harmful bacterial species and epithelial cells, thus playing a critical role in maintaining gut homeostasis[20,26].
We surmise that a cranberry extract, rich in both PAC and oligosaccharides, beneficially alters the gut microbiota and fosters the development of a niche conducive to beneficial gut microbiota. The objective of this study was to investigate the impact of cranberry extract on the gut microbiota of six healthy individuals, utilizing the artificial fermentation system TWIN-M-SHIME. This is an established in vitro system able to simulate the microbial ecosystem of the human gut dynamically operating two systems in parallel at the same time, remaining faithful to the original donor’s microbiota[27]. The system also included a simulated intestinal interface providing mucus-associated microbiotas mimicking mucosal microbial colonization. Specifically, the study sought to examine how cranberry extract influences the composition of commensal bacteria ex vivo, both within the lumen and in the mucus layer of the ascending and transverse section of the colon. In addition, we analyzed the production of fermentation by-products, specifically short-chain fatty acids (SCFA), to understand potential shifts in bacterial metabolic functions. Finally, we delved deeper into the ecological interactions through the examination of co-occurrence networks.
Our study revealed that cranberry extract supplementation significantly impacted the gut microbiota ecology, leading to a substantial shift in bacterial metabolism. Notably, Bifidobacterium adolescentis (B. adolescentis) thrived in the mucus of the ascending colon. The overall bacterial metabolism shifted from acetate to propionate and, especially, butyrate production. This shift was associated with the establishment of a consortium of keystone butyrogenic bacteria in the mucus of the transverse colon.
METHODS
Supplement
A cranberry extract (PrebiocranTM) was supplied by Symrise (Diana Food Canada Inc.). The composition of this (poly)phenolic extract rich in PAC can be found in previously published studies[28,29]. The dose was set to 86.8 mg PAC/day/donor, which corresponds to two capsules per day, or the equivalent of consuming
TWIN-M-SHIME® system
The TWIN-M-SHIME® simulates the microbial ecosystems of the human large intestine[27]. The first vessel replicated the stomach and the small intestine according to a fill-and-draw principle with the delivery of nutritional medium and pancreatic/bile juices, followed by vessels representing two repetitions of the ascending and transverse colon [Figure 1A] with fixed volumes. The detailed functioning of the system has been published[29] and it is described in Supplementary Data.
Figure 1. Cranberry extract, colon regions and lumen/mucus niches dynamically affect the microbiota in the TWIN-M-SHIME. (A) Schematic representation of the TWIN-M-SHIME® system setup. Each colonic region was inoculated in duplicate for each donor; (B-D) Partial distance-based redundancy analysis (db-RDA) of the microbial community composition based on 16S rRNA gene amplicon sequencing showing that treatment (B), colon region (C) and lumen/mucus niche (D) explain the differences in the microbiota. Explanatory variables are indicated in black (as shown in legend) and response variables are labeled in blue (genera abundances, only the principal explanatory variables are displayed for visibility). Percent on x- and y-axes indicate contribution to the total variance, while “Vexplained” corresponds to the variability of the gut microbiota composition explained by the variables (***P < 0.001 significance) assessed from the distance matrix PERMANOVA; (E) Stacked bar plots of gut microbiota genus-level relative abundance during specified days of fermentation: following two weeks of stabilization and one week of control period, the extract was added from the 21st day, at 86.8 mg PAC/day/donor. The graphic indicates the one-week control period (C; blue line), the first week of cranberry treatment (T; light red line), and the second week of cranberry treatment (T; dark red line). The top graph shows the proportion of the 20 most abundant genera in the lumen, while the bottom one of the mucus in the respective ascending and transverse colon. The stacked bar plots show the mean composition of six donors in duplicate. PAC: Proanthocyanidins.
A total of six healthy individuals with no recent history of antibiotic usage provided fecal samples across three experiments. Consent for fecal donation was obtained under registration number 2019-312 (Laval University, Canada). The selection of these donors was based on a prior screening of cranberry PAC urinary metabolite production on 11 subjects. Information regarding the gender, ethnicity, and dietary habits of the six donors is included in Supplementary Table 1. The candidates were selected as the most contrasting producers and non-producers of PAC urinary metabolites (phenyl-γ-valerolactones). The methods for fecal inoculum collection, preparation, and inoculation, along with details on the system’s overall operation, replacement of the mucin carrier, and the composition of the media, were outlined in a prior study[29] and are detailed in Supplementary Data.
The overall TWIN-M-SHIME® fermentation process lasted 33 days, consisting of a 12-day period to allow the microbiota to stabilize and adapt to the in vitro conditions, and a 7-day control phase in which the microbiota are only fed the SHIME feed and the pancreatic juices. The samplings during this period (3) are considered the control to assess the effect of the cranberry supplement fed to the system during the following 14 days. The treatment involved the supplementation of a cranberry extract (PrebiocranTM), providing 86.6 mg of flavan-3-ols (determined by phloroglucinolysis method[30,31]) per day, administered in the SHIME stomach with the second meal of the day.
DNA extraction
DNA extraction of the SHIME samples was previously described[29,32-34]. In the case of mucus microcosm samples, an additional step using sputolysin 0.1 M was included to enhance DNA yield and disrupt the disulfide bonds present in the mucins[35]. Finally, a purification step of DNA was added using the OneStepTM PCR Inhibitor Removal Kit (Zymo Research Corp., Orange, CA, United States) following the manufacturer’s protocol.
SCFA production
The effluents from the colon vessels of the SHIME were subjected to centrifugation at 18,000 × g for 8 min at 4 °C. SCFA were extracted using diethyl ether as previously described[29] and subsequently analyzed using a gas chromatograph system connected to a flame ionization detector. The concentration of SCFA was quantified in mM units.
Microbial community analysis
Following the DNA extraction from the SHIME samples, we performed next-generation sequencing on the V3-V4 region (341F-805R) of the 16S rRNA gene. For bioinformatics analysis, the Divisive Amplicon Denoising Algorithm (DADA2) workflow from the dada2 R package 1.26 was utilized[29]. When species identification using the SILVA database was incomplete, a manual refinement was conducted using the RDP SeqMatch classifier and NDPI Microbes BLASTN tools.
Statistical analysis
Statistical analysis was performed using R (v. 4.3.0). The Chao and Shannon indices were computed to assess the evolution of microbial community α-diversity between conditions using vegan package (v. 2.6-4) and ggpubr (v. 0.6.0) to compare means statistics using the Kruskal-Wallis test. The db-RDA method was used to determine the influence of treatment over time, gut regions, donors, and niches. The significance of group separation was assessed using PERMANOVA. DESeq2 package (v. 3.17) was used to find statistically significant differences in species/genera abundance between control and supplementation periods. P-values were adjusted for multiple testing using the Benjamini-Hochberg post-hoc test. Significant differences were visualized using box plots and volcano plots, as previously described[36]. The co-occurrence matrices were constructed with the SPIEC-EASI R package (v. 1.1.0)[37] based on the filtered and normalized abundance matrix of each condition, colon region, and niche. The resulting adjacency matrices were then uploaded in Cytoscape (v. 3.10.0) to visualize the microbial networks and analyze the networks, allowing for the keystone identification based on the high degree, low betweenness centrality, and high closeness centrality parameters, as previously described[38]. To evaluate the impact of the cranberry extract on the metabolic activity (SCFA), statistical analysis was conducted using the Kruskal-Wallis rank sum test with post hoc Dunn’s test. The Spearman’s correlations between the concentration of the three major SCFA and the relative abundance of the main bacterial genera were calculated using the corrr R package (v. 0.4.4).
RESULTS
The response of gut microbiota to the cranberry extract varies based on the specific colon region and ecology
The TWIN-M-SHIME® model was used to study changes in the microbial community composition in the ascending and transverse colon regions, encompassing both luminal and mucus content, over a two-week period of supplementation with cranberry extract, compared to a one-week control period [Figure 1A].
Six different healthy donors were tested over three different experiments, and their microbiota was first analyzed separately (data available in Supplementary Figures 1-5) and then pooled to evaluate the effect on the overall population. Distance-based redundancy analysis (db-DRA) confirmed that the supplementation had a strong impact on the overall differences observed in the microbial composition at the genus level (representing 5.5% of the total variation, P = 0.001, PERMANOVA) [Figure 1B]. The gut modulation effect was gradual over time, and therefore, the two weeks of supplementation were treated separately in subsequent analysis [Figure 1B]. As expected, the inter-individual variability accounted for a substantial percentage of the dissimilarities (35.9%, P = 0.001), but there was no apparent clustering between the donors [Supplementary Figure 6]. The colon regions (12.1%, P = 0.001) and the lumen/mucus environments (10.2%, P = 0.001) were significant explanatory variables as well [Figure 1C and D]; thus, we evaluated the average shifts in the relative abundance of the microbial community under supplementation separately for each specific region/environment [Figure 1E]. Distinct microbiota signatures were identified based on the top 20 most abundant genera. The genera Megasphaera and Bifidobacterium preferred the ascending colon region (Figure 1E, top left), while the transverse colon displayed a more diverse microbiota, including genera like Akkermansia, as anticipated (Figure 1E, top right). The Roseburia and Bifidobacterium genera effectively colonized a significant portion of the mucus environment in the transverse and ascending colon, respectively (Figure 1E, bottom).
The cranberry extract induces a stronger microbiota modulation in the ascending colon than in the transverse colon
Supplementation with cranberry extract led to a notable enhancement in α-diversity species richness, as measured by the Chao index, exclusively in the lumen (P = 0.05, Kruskal-Wallis) and mucus (P = 0.001) environments of the ascending colon. However, there was a simultaneous decline in species evenness in the mucus of the same colon region (P = 0.001), according to the Shannon index, as detailed in Table 1.
Changes in species richness and evenness induced by the supplementation with the cranberry extract
Condition | Mean Chao index (X10000) | Mean Shannon index |
Lumen ascending colon control week | 27.07 (± 20.28) | 2.95 (± 0.54) |
Lumen ascending colon treatment week 1 | 30.86 (± 1.84) | 3.03 (± 0.66) |
Lumen ascending colon treatment week 2 | 32.45 (± 20.96)* | 2.79 (± 0.56) |
Mucus ascending colon control week | 33.25 (± 19.36) | 3.13 (± 0.82) |
Mucus ascending colon treatment week 1 | 54.27 (± 25.90)* | 2.60 (± 1.33)** |
Mucus ascending colon treatment week 2 | 61.00 (± 24.06)*** | 2.16 (± 0.42)*** |
Lumen transverse colon control week | 51.23(± 8.60) | 4.12 (± 0.18) |
Lumen transverse colon treatment week 1 | 51.89 (± 6.46) | 4.11 (± 0.36) |
Lumen transverse colon treatment week 2 | 50.48 (± 13.65) | 3.99 (± 0.38) |
Mucus transverse colon control week | 42.34 (± 21.90) | 3.68 (± 0.67) |
Mucus transverse colon treatment week 1 | 38.98 (± 22.83) | 3.80 (± 0.48) |
Mucus transverse colon treatment week 2 | 41.55 (± 24.24) | 3.70 (± 1.02) |
The DESeq analysis additionally revealed significant variations in the relative abundance of numerous genera within the luminal and mucus microbiota of the ascending colon when comparing the control week to the second week supplemented with the cranberry extract (Figure 2A-C, Supplementary figures 7 and 8 for donor-specific results).
Figure 2. The supplementation with a cranberry extract exhibits distinct effects on microbiotas across different colon regions. Volcano plots displaying the significantly enriched genera after supplementation as determined using a Wald test with Benjamini-Hochberg multiple testing correction in the lumen (A) and mucus (C) of the ascending colon and the lumen (B) and mucus (D) of the transverse colon, respectively. Deseq2 analysis of log2 fold-changes displays on the left of the x-axis (in blue) the genera depleted after the two weeks of supplementation, while on the right (in red), the ones enriched after the two weeks of supplementation. The y-axis shows the log-transformed adjusted P-value with the dashed line indicating the α = 0.05 significance threshold. Statistical differences between the control and the second week of supplementation were determined using a Wald Test with Benjamini-Hochberg multiple correction test.
Strong enrichment (> 4 log2FC, P < 0.05, Wald test) of genera belonging to Proteobacteria (Shigella, Escherichia-Shigella, and Klebsiella) (6/6 donors), Anaerostipes (2/6 donors), and Lactiplantibacillus (4/6 donors) was specifically observed in the luminal ascending colon and, to a lesser but still significant extent, Phascolarctobacterium (3/6 donors), Acidaminococcus (1/6 donors), and Roseburia (1/6 donors) were also stimulated, while Bifidobacterium (5/6 donors), Parabacteroides (3/6 donors), and Faecalibacterium (1/6 donors) were depleted (> -4 log2FC, P < 0.05) [Figure 2A]. In the mucus environment of the same region, the genera Escherichia-Shigella (3/4 donors), Anaerostipes (3/4 donors), and Lactobacillus (2/4 donors) responded positively to the treatment (> 4 log2FC, P < 0.05), alongside Bifidobacterium (4/4 donors) that strongly colonized the mucus [Figure 2C]. Although no change in α-diversity was observed in the transverse colon following supplementation, DESeq analysis revealed that several genera were affected in both the luminal and mucus environments of this region (Figure 2B-D, Supplementary figures 7 and 8 for donor-specific results). In the lumen, the relative proportions of Anaerostipes (3/6 donors), Akkermansia (3/6 donors), and Lactiplantibacillus (4/6 donors) increased after supplementation alongside some Proteobacteria genera (Aquamonas, Shigella, Escherichia-Shigella, and Klebsiella) (> 3 log2FC, P < 0.05) (5/6 donors), while Prevotella (3/6 donors) and some genera of the Oscillospiraceae family
Bifidobacterium genus responds differently to the cranberry extract in the ascending colon, showing a preference for B. adolescentis in the mucus environment
The cranberry extract had a significant impact on the Bifidobacterium genus in the ascending colon, showing a distinctive and varied response depending on the intestinal niche observed [Figure 3A and B].
Figure 3. Bifidobacterium species shifts induced by the supplementation with a cranberry extract in the ascending colon. (A and B) Bifidobacterium genus behavior following the supplementation in the lumen (A) and mucus (B), respectively, as evaluated by Deseq2 analysis. The changes between the control and the second week of supplementation are significantly different at α = 0.05, as determined using a Wald Test with Benjamini-Hochberg multiple testing correction; (C and D) Volcano plots showing the ASVs assigned to Bifidobacterium species significantly affected by the supplementation as determined by the Wald test with Benjamini-Hochberg multiple testing correction in the lumen (C) and mucus (D) of the ascending colon. Deseq2 analysis of log2 fold-changes displays on the left of the x-axis (in blue) the ASVs depleted after the two weeks of supplementation, while on the right (in red), the ones enriched after the two weeks of supplementation. Each ASV sequence was assigned to the corresponding species based on RDP classifier. The y-axis shows the log-transformed adjusted P-value with the dashed line indicating the α = 0.05 significance threshold.
In the lumen, the relative abundance of this species gradually decreased over the two-week supplementation period (5/6 donors) (P < 0.05) [Figure 3A], while it exhibited a remarkable propensity to flourish within the mucus of the same colon region (4/4 donors) (P < 0.05) [Figure 3B], attaining roughly 40% of the total relative abundance during the second week of treatment (Figure 1E, bottom left). Upon closer examination, the RDP and NBLAST assignations revealed that several ASVs (ASV_1, ASV_23, ASV_633, ASV_1417) associated with the B. adolescentis species were the main colonizers, making up over 50% of the Bifidobacterium species found in the mucus [Supplementary Figure 9]. Several other ASVs associated with different Bifidobacterium species [B. faecale (ASV_58, ASV_341), B. longum (ASV_20, ASV_74),
The extent to which the cranberry extract stimulates known PAC-degrading bacterial species is largely influenced by the donors’ microbiota baseline composition
So far, only a limited number of gut bacterial strains have been shown to metabolize PAC, namely Adlercreutzia equolifaciens, Eggerthella lenta, Slackia equolifaciens, Flavonifractor plautii, Eubacterium oxidoreducens, and Lactiplantibacillus plantarum [Table 2].
Characterization of species known to be implicated in the PAC metabolism
Species | Phylum (class) | Reaction | Ref. |
Adlercreutzia equolifaciens | Actinobacteria (Coriobacteriia) | Dehydroxylation C4’ C-ring cleavage | [8,39] |
Eggerthella lenta [ASV_1702, (100%)] | Actinobacteria (Coriobacteriia) | C-ring cleavage | [8,39-41] |
Slackia equolifaciens | Actinobacteria (Coriobacteriia) | C-ring cleavage | [8,39] |
Flavonifractor plautii [ASV_655, (98.02%)] | Firmicutes (Clostridia) | Further degradation | [8,41,42] |
Eubacterium oxidoreducens | Firmicutes (Clostridia) | A-ring cleavage | [8,43] |
Lactiplantibacillus plantarum [ASV_69 (100%), ASV_238 (99.77%), ASV_617 (91.86%), ASV_1511 (99.65%), ASV_1979 (91.63%)] | Firmicutes (Bacilli) | C-ring cleavage | [8,44] |
While 16S rRNA gene sequencing limits our ability to identify organisms beyond the species level, we have closely monitored the progression of ASVs assigned to these species throughout the fermentation process. This approach has enabled us to semiquantitatively track their presence and response to the supplementation. The DESeq analysis revealed that both the presence of these species and their response to the supplementation are significantly influenced by the individual donor and the specific region or niche within the intestinal environment [Figure 4].
Figure 4. Follow-up of bacterial species known to be involved in the PAC metabolism. Evolution of bacteria species potentially involved in PAC metabolism during the TWIN-M-SHIME® fermentation for the six donors. Deseq2 analysis was performed separately in the lumen of the (A) ascending and (B) transverse colon and in the mucus of the (C) ascending and (D) transverse colon. Statistical differences between the control and cranberry extract supplementation across gut regions were determined using a Wald Test with Benjamini-Hochberg multiple testing correction. Only species with statistical differences (α = 0.05) between the control and the second week of treatment are presented. PAC: Proanthocyanidins.
In the case of the ascending colon, various ASVs linked to the species L. plantarum/L. fabifermentans (such as ASV_69, ASV_238, ASV_617, ASV_1979) showed a significant positive response to the cranberry extract supplementation. This response was observed both in the lumen and in the mucus environment [Figure 4A-C]. In the transverse colon, we also observed a significant growth of L. plantarum/fabifermentas (ASV_69, ASV_238, ASV_617, ASV_1511), alongside F. plautii (ASV_655) and E. lenta (ASV_1702) that bloomed in the mucus of only one specific donor [Figure 4B and D].
The administration of cranberry extract led to the development of butyrogenic keystone bacterial guilds in both the luminal and mucus regions of the transverse colon, resulting in a marked increase in butyrate production
To determine strong bacterial associations, we evaluated the evolution of co-occurrence interactions over the fermentation period. By organizing the ASV table into subsections for the two colon regions, the two environments, and the week of control/treatment, we conducted an analysis that resulted in 12 distinct networks [Supplementary Figure 10]. The topological properties of these co-occurrence networks are available in Supplementary Table 2. This method represents ASVs as interconnected nodes when a co-occurrence relationship is statistically significant between them. The visualization of the resulting networks showed that the cranberry extract improved the co-occurrence patterns after two weeks of supplementation, resulting in more frequent significant correlations between different ASVs [Supplementary Figure 10]. By analyzing these co-occurrence networks, we were able to identify two bacterial guilds specific to the lumen and the mucus environments of the transverse colon [Figure 5], despite not being able to reveal interesting patterns in the ascending colon [Supplementary Figure 10A-F].
Figure 5. Establishment of butyrogenic guilds and identification of keystone species following the cranberry extract supplementation. (A and B) Networks of significant co-occurrences between the bacterial species (ASV) based on 16S rRNA gene amplicon sequencing in the transverse colon for the lumen (A) and the mucus (B) during the second week of supplementation. The nodes are shaped according to the corresponding phylum and the edges indicate a positive (full lines) or negative (dotted lines) correlation between the network nodes. From the networks, bacterial guilds are displayed. Red nodes indicate the ASVs identified as keystone species according to network analysis of high degree, low betweenness centrality, and high closeness centrality simultaneously significant at the α = 0.05 interval.
Each guild revolved around key members (i.e., keystone species), identified through three topological network parameters: high degree, low betweenness centrality, and high closeness centrality [Supplementary Table 3], as previously shown[38]. Megasphaera micronuciformis (ASV_6), Bacteroides vulgatus (ASV_25),
In fact, in both colon regions, butyrate concentrations increased progressively during fermentation and were significantly higher after two weeks of supplementation (6/6 donors) (P < 0.001), even surpassing acetate concentrations and ratios in the ascending colon [Figure 6A and B].
Figure 6. SCFA modulation by cranberry extract supplementation and correlations with bacterial genera. (A) Mean ratio and (B) mean concentration ±SD over time of the three major SCFA for the six donors in the ascending and transverse colon during the control week (blue line) and the two subsequent weeks of treatment (week 1: light red, week 2: dark red). Statistically significant differences between control and treatment period are denoted for P < 0.05 (*), P < 0.01 (**), and P < 0.001 (***) as determined by Kruskal-Wallis followed by post-hoc Dunn’s test. (C-F) Spearman correlations between the three major SCFA and bacterial genera in the transverse colon. Correlations were performed in the lumen (C and E) and mucus (D and F) environments separately during the first (C and D) and second week (E and F) of supplementation. Only negative and positive significant correlations (P < 0.05 Benjamini-Hochberg adjusted) that are greater than a -0.7 or 0.7 coefficient are shown, denoted in shades of orange and purple, respectively. SCFA: Short-chain fatty acid.
During the control period, both regions of the colon maintained a physiological ratio of 3:1:1 for acetate, propionate, and butyrate [Figure 6A], but in response to the cranberry extract supplementation, the SCFA profile changed. The ratios of propionate and, notably, butyrate increased concurrently [Figure 6A]. This outcome was attributed to a rapid and substantial decrease in acetate concentration during the initial week of treatment (6/6 donors) (P < 0.001). Although the rate of decline stabilized, it remained significantly lower compared to the control period throughout the second week as well [Figure 6B]. The effect of the supplementation on acetate production is more pronounced in the ascending colon, but still present to a lesser extent in the transverse colon (5/6 donors) [Figure 6B]. The changes in propionate production were not prominent, but still showed a significant increase in response to the cranberry extract in the transverse colon after two weeks of supplementation (5/6 donors) (P < 0.01) [Figure 6B]. During the first week of supplementation in the ascending colon, there was a slight decrease in propionate production. However, by the second week, it returned to the same levels observed during the control period (5/6 donors) [Figure 6B]. Minor SCFA (e.g., branched-chain fatty acids and valerate) mean production was also assessed
DISCUSSION
Given the growing body of evidence underscoring the significance of gut microbiota in health and disease[7,45,46], the research emphasis on the health implications of (poly)phenols has evolved. Instead of concentrating on their direct antioxidant properties, current studies are increasingly examining their interactions with the gut microbiota[8,12-14]. This is particularly relevant to PAC, since they are poorly absorbed in the upper digestive tract and reach the colon intacts[7-9]. This interaction is reciprocal; that is, PAC both alter the composition and are presumed to be metabolized by the gut microbiota. However, this relationship is yet to be fully characterized, and may provide key insight into the crucial mechanism underlying their health benefits. Moreover, the interplay between oligosaccharides, present at approximately 120 mg/day in our study[28], and PAC needed further examination since few studies have taken into account this combination[10,11].
To advance our understanding of the influence of supplementing the microbiota with a cranberry extract, we conducted a two-week study in the ex vivo TWIN-M-SHIME®. This system was inoculated with fecal samples from six healthy individuals to ensure an adequate representation of the population and provide a suitable sample size. The TWIN-M-SHIME® enabled us to closely replicate the physiology of the gastrointestinal tract. It not only captured its inherent dynamism, but also provided us the opportunity to investigate the mucosal environment, which is typically inaccessible when conducting in vivo human studies.
Although the luminal and mucosal environments have distinct microbiota profiles, it is worth noting that regional variations along the length of the colon are equally significant. These regional differences can influence how nutrients are metabolized[47]. The M-SHIME system used in the experiments was set up to replicate the conditions of the ascending and transverse colon, as flavan-3-ols monomers and dimers are typically broken down in these specific regions of the colon[15]. Our research conclusively showed that a two-week regimen of cranberry extract supplementation significantly influenced the gut microbiota. The effects of this supplementation varied depending on the particular niche and region within the gastrointestinal tract.
The impact of the supplementation varied notably between the two regions of the colon. In the ascending colon, there was a more pronounced decrease in acetate and a generally more significant modulation of the microbiota. This observation might be attributed to two factors: a rapid metabolism of a fresh batch of unmodified compound upon the transfer of the stomach content directly in the ascending colon, and a dilution impact due to the unique fluid dynamics of the SHIME system[48]. The ultimate result of the microbial modulation induced by the cranberry extract was a significant increase in butyrate levels throughout both regions of the colon. This finding aligns with other in vitro and in vivo studies that have investigated the effects of PAC from various fruits (such as cranberry, aronia berry, and grape) on microbial metabolism, which have also noted an increase in butyrate levels[15,16,49,50]. This response is, however, not unique to cranberry (poly)phenols as the ethanolic extract also contains a significant amount of oligosaccharides, which have also been correlated to an increase in the production of butyrate in literature[11,51]. In the ascending colon lumen, the supplementation led to an increased relative abundance
The analysis of co-occurrence networks further revealed the importance of butyrogenic species in the community. Examining co-occurrence patterns and identifying node species can offer more comprehensive insights compared to solely depending on data about species abundance. These approaches can help us infer not only the structure of the microbial community but also its ecological functions and dynamics[57-60]. Furthermore, these methods enable the identification of keystone species, which play a pivotal role in the community, irrespective of their abundance[38,57,60,61]. Among the species identified as keystones in the lumen of the transverse colon, Anaerobutyricum soehngenii has been recently identified as a next-generation probiotic due to its capacity to regulate insulin sensitivity by producing butyrate from acetate and D,L-lactate and influencing bile acid metabolism[62]. Megasphaera micronuciformis was also identified as a keystone species capable of producing SCFA, including acetate, propionate, butyrate, and, notably, valerate. This species was predominant in the microbiota of only one donor, which also had an elevated production of valerate. Therefore, its importance could be derived from this specific donor data. F. plautii emerged as another keystone species. Known for its butyrogenic properties[63], this species has been documented to be involved in the metabolism of catechins[8] and in the attenuation of gut inflammation[64-66]. The last keystone of the lumen environment, B. vulgatus, has been shown to attenuate gut microbiota lipopolysaccharides production and can utilize succinate to produce propionate[67,68]. Propionate plays a role in the regulation of satiety, the reduction of cholesterol and lipogenesis, and the maintenance of metabolic homeostasis[16,67]. The production of this SCFA was also increased after supplementation and several other genera were recognized as propionate-producers, such as Akkermansia, Alistipes, Anaerobutyricum, Phascolarctobacterium, and Roseburia, which were stimulated in the mucus environment of the transverse colon[50-56]. In the mucus environment, A. hallii also produces butyrate as well as propionate from the same secondary acids or from metabolites of previously degraded complex carbohydrates[69]. It has been documented that A. hallii engages in cross-feeding by utilizing acetate and lactate generated by lactobacilli and bifidobacteria species in culture[52,69]. This cross-feeding interaction has been observed, particularly with B. adolescentis[53]. Additionally, these Anaerobutyricum species also partake in symbiotic relationships with mucin-degrading bacteria[69,70]. In particular, A. hallii leverages 1,2-propanediol generated by A. muciniphila from fucose to synthesize propionate[70]. These two bacteria hold great potential as next-generation probiotics. In fact, specific strains are already available on the market as components of a dietary supplement that demonstrated improved post-prandial glucose control in subjects with type 2 diabetes[71]. The enhancement of A. muciniphila by PAC and (poly)phenol-rich foods has been well documented in scientific literature[20,22,24,70], and associated with a range of beneficial health effects[23,24,69,72,73], including the stimulation of mucus production and the amelioration of gut barrier integrity[17,23,24]. The other identified keystones in the mucus environment, R. faecis, I. butyriciproducens, and A. butyriciproducens, are also butyrate-producers[50,51,74-76], while H. saccharivorans is a carbohydrate consumer that may contribute to cross-feeding in the ecosystem by producing H2 and acetate[77].
The existence of a consortium of butyrate-producing bacteria within the mucus environment strongly suggests a specialized ecological role. The presence of butyrate in this ecological niche is important as it facilitates its delivery near the epithelial layer, where it can enhance the integrity of the barrier[50,67,78]. The importance of the mucosal niche is extremely important in the interplay of Bifidobacteria/Proteobacteria. The mucus provided a favorable environment for Bifidobacteria to thrive during the supplementation, unlike the Escherichia-Shigella, which did not proliferate as they had in the lumen. The increased abundance of beneficial bacteria, including Bifidobacteria and other butyrate-producing genera like Lactiplantibacillus, Roseburia, and Anaerostipes, is concordant with various indicators suggestive of a healthy microbial community associated with mucus. Such a microbiota composition could potentially blunt intestinal inflammation[79-82]. Conversely, several other genera, such as Bifidobacteria, could not withstand the elevated PAC concentration in the lumen of the ascending colon, leading to a substantial decline. This is consistent with other ex vivo studies using the SHIME system with various other (poly)phenols supplementations such as grape seed, red wine and black tea, aronia berry, and pomegranate[83]. However, in the mucus, Bifidobacteria managed to not only survive but also prosper. Such a dual relationship has been documented in past studies, where Bifidobacteria succeeded in establishing a niche within the mucus[84-86]. B. adolescentis emerged as the dominant Bifidobacteria species across the fermentation system and responded positively to the supplementation, particularly in the mucus environment. This result was also recently confirmed in a human clinical trial conducted by our group, where a bloom of B. adolescentis was observed in participants who consumed the same supplementation regimen as in the present experiment[28]. Research has demonstrated that several strains can adhere to the epithelium and metabolize host mucin glycans[50,87-89], in line with our observed colonization of the mucus. The favorable reaction of B. adolescentis to the supplementation could also be linked to oligosaccharides contained in the cranberry extract. Bifidobacteria are capable of metabolizing this particular carbon source for growth[50,87,88]. Recent research has revealed multiple health benefits attributed to B. adolescentis, including regulation of inflammation, immune responses[87,89-92], and alleviating chronic metabolic disorders[92,93]. B. adolescentis breaks down non-digestible carbohydrates or mucin glycoproteins to produce acetate and lactate. These compounds then serve as substrates for butyrate-producing strains like F. prausnitzii, A. hallii, A. caccae, or Roseburia sp.[52-55], all of which showed an increase in response to our PAC supplementation. Ecologically speaking, this underscores the significance of cross-feeding among the diverse inhabitants of the mucosal ecological niche, leading to the ultimate production of butyrate and its ensuing physiological impacts.
Interestingly, the combined effect of PAC and oligosaccharides may be more impactful than either component alone. The binding of (poly)phenols to carbohydrates not only enhances their solubility, making it easier for them to reach the colon[10,56], but also uniquely influences the composition of the gut microbiota through their simultaneous metabolism[11]. For example, it has been demonstrated that L. plantarum can grow on oligosaccharides alone, but utilizes them more efficiently in the presence of PAC[56]. Hence, the significant increase in L. plantarum, another species known for its butyrate-producing abilities[94,95] observed in all colon compartments and environments in our study, could potentially be attributed to this synergistic effect between PAC and oligosaccharides.
L. plantarum is also one of the species involved in PAC metabolism. Two other species known to be active in this metabolic process were stimulated by the supplementation, but only in the transverse colon. Eggerthella lenta in the mucus environment is capable of converting (+)catechin and (-)epicatechin into
The presence of PAC-degrading species is one example of inter-individual variability observed in the present study: E. lenta was stimulated only in one donor out of six. We observed several differences between the six subjects, not only in the baseline microbiota but also in response to the PAC supplementation. Notably, distinct SCFA-producing species have been stimulated depending on the donors, such as Akkermansia, Alistipes, Lactiplantibacillus, Phascolarctobacterium, Faecalibacterium, Subdoligranulum alongside several members of the Lachnospiraceae family. Nevertheless, these differences did not impact the final biological response, as shown by the homogeneous response in SCFA modulation. It is to be anticipated that other biological effects will most probably be different since host-microbiome interaction is a crucial factor to be taken into account.
While this study offers valuable insights into the interaction between cranberry flavan-3-ols, oligosaccharides, and gut microbiota, several limitations should be acknowledged to contextualize the findings and suggest avenues for future research. Firstly, the ex vivo TWIN-M-SHIME system, while sophisticated and able to simulate the human gastrointestinal tract, cannot completely replicate the complexity of in vivo conditions. The physiological interactions that occur within a living organism are absent in this model. Factors such as the immune system, mucosal barrier function, and host metabolic responses play significant roles in shaping gut microbiota and their metabolic outputs. Additionally, metabolites accumulate in bioreactors due to the lack of dialysis, leading to higher absolute concentrations than physiological levels, which could impact gut microbiota dynamics. Oxygen concentrations, pressure, and REDOX potential in the gut rise from the lumen to the mucus, parameters that cannot be accurately replicated in ex vivo systems. This can affect the colonization of the mucus, favoring facultative anaerobes such as species from Actinobacteria or Proteobacteria phyla in the colon mucosa. Moreover, SCFAs can accumulate in the mucus of the microcosms of the M-SHIME system, thereby affecting local pH and favoring more acidophilic species. Thus, while the system provides valuable mechanistic insights, the findings may not fully translate to human physiology. Furthermore, the study involved fecal samples from only six healthy individuals. While this number is adequate for mechanistic analysis in an ex vivo system, it limits the generalizability of the results. Inter-individual variability in gut microbiota composition and response to dietary interventions could mean that the findings are not representative of the broader population. Nevertheless, the effects of the treatment on microbiota modulation in our experiment are comparable to those observed in a recent human clinical study on 28 subjects conducted by our group, in which we observed a strong bifidogenic effect that corroborates the in vitro model observations and reliability. Finally, although the study provided mechanistic insights into the interactions between cranberry extract and gut microbiota, these mechanisms were not confirmed in the context of host interactions. Incorporating host cells and innate immune responses in future studies would help to confirm and expand upon the potential effects observed in the ex vivo model.
Overall, this study provides novel insights into the intricate relationship between cranberry PAC/oligosaccharides, gut microbiota composition, and their potential health benefits. We realized a host-independent study to gain mechanistic insights into the duplibiotic relation between a cranberry extract and the gut microbiota. The findings suggest that supplementing with a cranberry extract has the potential to modulate the gut microbiota in ways that could promote gut health by ameliorating the SCFA production profile and favoring the establishment of butyrogenic guilds, specifically in the mucus environment. Given the overlooked oligosaccharides content in cranberry products, more mechanistic studies are needed to investigate the interplay between these dietary components and the gut microbiota. Additional research, combined with the study of host cells and their associated innate immune cells, could provide confirmation of the potential effects of the intricate microbial metabolome on the intestinal epithelium.
DECLARATIONS
Acknowledgments
This research was funded by the NSERC-Diana Food Industrial Chair on prebiotic effects of fruits and vegetables. We thank Symrise (Diana Food Canada Inc.) for the supply of the PrebiocranTM extract and for the scientific inputs. We would like to acknowledge Patricia Savard and Camille Cavestri for their assistance in performing in vitro fermentations. We are grateful to the INAF analytical platform, especially Perrine Feutry and Roxanne Nolet, for their assistance in SCFA measurements. Thanks to Joseph Lupien-Meilleur for the illustrations of the SHIME bioreactors and Mérilie Gagnon for assistance in creating the graphical abstract.
Authors’ contributions
Conceived and designed the research: Cattero V, Roussel C, Roy D, Desjardins Y
Performed the research, acquired, analyzed, and interpreted the data: Cattero V
Supervised and helped the entire process of performing research, acquiring, analyzing and interpreting data: Roussel C
Supervised the project: Desjardins Y
Characterized the cranberry extract and performed the metabolomics analysis to select the donors: Lessard-Lord J
All authors revised the manuscript.
Availability of data and materials
The dataset supporting the conclusions of this article is available in the NCBI repository (SRA Bioproject number PRJNA1046640 at https://www.ncbi.nlm.nih.gov/bioproject/PRJNA1046640.
Financial support and sponsorship
This research was funded by the NSERC-Diana Food Industrial Chair on prebiotic effects of fruits and vegetable polyphenols (project number: CRSNG IRCPJ 531009-17).
Conflicts of interest
Desjardins Y holds an NSERC-Symrise Industrial Chair on prebiotic effects of fruit and vegetable (poly)phenols, while the other authors have declared that they have no conflicts of interest.
Ethical approval and consent to participate
Consent for fecal donation was obtained under registration number 2019-312 (Laval University Ethical Committee, Canada) and ethical approbation number 2019-312/22-01-2020. Informed consent was obtained from fecal donors.
Consent for publication
Not applicable.
Copyright
© The Author(s) 2024.
Supplementary Materials
REFERENCES
1. Smeriglio A, Barreca D, Bellocco E, Trombetta D. Proanthocyanidins and hydrolysable tannins: occurrence, dietary intake and pharmacological effects. Br J Pharmacol 2017;174:1244-62.
2. Mannino G, Chinigò G, Serio G, et al. Proanthocyanidins and where to find them: a meta-analytic approach to investigate their chemistry, biosynthesis, distribution, and effect on human health. Antioxidants 2021;10:1229.
3. Rauf A, Imran M, Abu-Izneid T, et al. Proanthocyanidins: a comprehensive review. Biomed Pharmacother 2019;116:108999.
4. Favari C, Mena P, Curti C, Del Rio D, Angelino D. Flavan-3-ols: catechins and proanthocyanidins. In: Tomás-barberán FA, González-sarrías A, García-villalba R, editors. Dietary polyphenols. Wiley; 2020. pp. 283-317.
5. Feldman F, Koudoufio M, El-Jalbout R, et al. Cranberry proanthocyanidins as a therapeutic strategy to curb metabolic syndrome and fatty liver-associated disorders. Antioxidants 2022;12:90.
6. de la Iglesia R, Milagro FI, Campión J, Boqué N, Martínez JA. Healthy properties of proanthocyanidins. Biofactors 2010;36:159-68.
7. Rowland I, Gibson G, Heinken A, et al. Gut microbiota functions: metabolism of nutrients and other food components. Eur J Nutr 2018;57:1-24.
8. Tao W, Zhang Y, Shen X, et al. Rethinking the mechanism of the health benefits of proanthocyanidins: absorption, metabolism, and interaction with gut microbiota. Compr Rev Food Sci Food Saf 2019;18:971-85.
9. Lessard-Lord J, Roussel C, Guay V, Desjardins Y. Assessing the gut microbiota’s ability to metabolize oligomeric and polymeric flavan-3-ols from aronia and cranberry. Mol Nutr Food Res 2024;68:e2300641.
10. Coleman CM, Ferreira D. Oligosaccharides and complex carbohydrates: a new paradigm for cranberry bioactivity. Molecules 2020;25:881.
11. Karboune S, Davis EJ, Fliss I, Spadoni Andreani E. In-vitro digestion and fermentation of cranberry extracts rich in cell wall oligo/polysaccharides. J Funct Foods 2022;92:105039.
12. Sallam IE, Abdelwareth A, Attia H, et al. Effect of gut microbiota biotransformation on dietary tannins and human health implications. Microorganisms 2021;9:965.
13. Ma G, Chen Y. Polyphenol supplementation benefits human health via gut microbiota: a systematic review via meta-analysis. J Funct Foods 2020;66:103829.
14. Rodríguez-Daza MC, Pulido-Mateos EC, Lupien-Meilleur J, Guyonnet D, Desjardins Y, Roy D. Polyphenol-mediated gut microbiota modulation: toward prebiotics and further. Front Nutr 2021;8:689456.
15. van Dorsten FA, Peters S, Gross G, et al. Gut microbial metabolism of polyphenols from black tea and red wine/grape juice is source-specific and colon-region dependent. J Agric Food Chem 2012;60:11331-42.
16. Wu T, Grootaert C, Pitart J, et al. Aronia (aronia melanocarpa) polyphenols modulate the microbial community in a simulator of the human intestinal microbial ecosystem (SHIME) and decrease secretion of proinflammatory markers in a caco-2/endothelial cell coculture model. Mol Nutr Food Res 2018;62:e1800607.
17. Rodríguez-Daza MC, Daoust L, Boutkrabt L, et al. Wild blueberry proanthocyanidins shape distinct gut microbiota profile and influence glucose homeostasis and intestinal phenotypes in high-fat high-sucrose fed mice. Sci Rep 2020;10:2217.
18. Cires MJ, Navarrete P, Pastene E, et al. Effect of a proanthocyanidin-rich polyphenol extract from avocado on the production of amino acid-derived bacterial metabolites and the microbiota composition in rats fed a high-protein diet. Food Funct 2019;10:4022-35.
19. Chen Y, Wang J, Zou L, Cao H, Ni X, Xiao J. Dietary proanthocyanidins on gastrointestinal health and the interactions with gut microbiota. Crit Rev Food Sci Nutr 2023;63:6285-308.
20. Dueñas M, Muñoz-González I, Cueva C, et al. A survey of modulation of gut microbiota by dietary polyphenols. Biomed Res Int 2015;2015:850902.
21. Taibi A, Lofft Z, Laytouni-Imbriaco B, Comelli EM. The role of intestinal microbiota and microRNAs in the anti-inflammatory effects of cranberry: from pre-clinical to clinical studies. Front Nutr 2023;10:1092342.
22. Redondo-Castillejo R, Garcimartín A, Hernández-Martín M, et al. Proanthocyanidins: impact on gut microbiota and intestinal action mechanisms in the prevention and treatment of metabolic syndrome. Int J Mol Sci 2023;24:5369.
23. Anhê FF, Pilon G, Roy D, Desjardins Y, Levy E, Marette A. Triggering Akkermansia with dietary polyphenols: a new weapon to combat the metabolic syndrome? Gut Microbes 2016;7:146-53.
24. Rodríguez-Daza MC, de Vos WM. Polyphenols as drivers of a homeostatic gut microecology and immuno-metabolic traits of Akkermansia muciniphila: from mouse to man. Int J Mol Sci 2022;24:45.
25. Everard A, Belzer C, Geurts L, et al. Cross-talk between Akkermansia muciniphila and intestinal epithelium controls diet-induced obesity. Proc Natl Acad Sci U S A 2013;110:9066-71.
26. Andersen-Civil AIS, Arora P, Williams AR. Regulation of enteric infection and immunity by dietary proanthocyanidins. Front Immunol 2021;12:637603.
27. Dupont D, Alric M, Blanquet-Diot S, et al. Can dynamic in vitro digestion systems mimic the physiological reality? Crit Rev Food Sci Nutr 2019;59:1546-62.
28. Lessard-Lord J, Roussel C, Lupien-Meilleur J, et al. Short term supplementation with cranberry extract modulates gut microbiota in human and displays a bifidogenic effect. NPJ Biofilms Microbiomes 2024;10:18.
29. Roussel C, Chabaud S, Lessard-Lord J, et al. UPEC colonic-virulence and urovirulence are blunted by proanthocyanidins-rich cranberry extract microbial metabolites in a gut model and a 3D tissue-engineered urothelium. Microbiol Spectr 2022;10:e0243221.
30. Dufour C, Villa-Rodriguez JA, Furger C, et al. Cellular antioxidant effect of an aronia extract and its polyphenolic fractions enriched in proanthocyanidins, phenolic acids, and anthocyanins. Antioxidants 2022;11:1561.
31. Jakobek L, García-villalba R, Tomás-barberán FA. Polyphenolic characterisation of old local apple varieties from Southeastern European region. J Food Compos Anal 2013;31:199-211.
32. Roussel C, De Paepe K, Galia W, et al. Spatial and temporal modulation of enterotoxigenic E. coli H10407 pathogenesis and interplay with microbiota in human gut models. BMC Biol 2020;18:141.
33. Van de Wiele T, Van den Abbeele P, Ossieur W, Possemiers S, Marzorati M. The simulator of the human intestinal microbial ecosystem (SHIME®). In: Verhoeckx K, Cotter P, López-expósito I, Kleiveland C, Lea T, Mackie A, Requena T, Swiatecka D, Wichers H, editors. The Impact of food bioactives on health. Cham: Springer International Publishing; 2015. pp. 305-17.
34. Geirnaert A, Wang J, Tinck M, et al. Interindividual differences in response to treatment with butyrate-producing Butyricicoccus pullicaecorum 25-3T studied in an in vitro gut model. FEMS Microbiol Ecol 2015;91:fiv054.
35. Roussel C, Anunciação Braga Guebara S, Plante PL, Desjardins Y, Di Marzo V, Silvestri C. Short-term supplementation with ω-3 polyunsaturated fatty acids modulates primarily mucolytic species from the gut luminal mucin niche in a human fermentation system. Gut Microbes 2022;14:2120344.
36. De Paepe K, Verspreet J, Verbeke K, Raes J, Courtin CM, Van de Wiele T. Introducing insoluble wheat bran as a gut microbiota niche in an in vitro dynamic gut model stimulates propionate and butyrate production and induces colon region specific shifts in the luminal and mucosal microbial community. Environ Microbiol 2018;20:3406-26.
37. Kurtz ZD, Müller CL, Miraldi ER, Littman DR, Blaser MJ, Bonneau RA. Sparse and compositionally robust inference of microbial ecological networks. PLoS Comput Biol 2015;11:e1004226.
38. Banerjee S, Schlaeppi K, van der Heijden MGA. Keystone taxa as drivers of microbiome structure and functioning. Nat Rev Microbiol 2018;16:567-76.
39. Takagaki A, Nanjo F. Bioconversion of (-)-epicatechin, (+)-epicatechin, (-)-catechin, and (+)-catechin by (-)-epigallocatechin-metabolizing bacteria. Biol Pharm Bull 2015;38:789-94.
40. Takagaki A, Kato Y, Nanjo F. Isolation and characterization of rat intestinal bacteria involved in biotransformation of
42. Kutschera M, Engst W, Blaut M, Braune A. Isolation of catechin-converting human intestinal bacteria. J Appl Microbiol 2011;111:165-75.
43. Stoupi S, Williamson G, Drynan JW, Barron D, Clifford MN. Procyanidin B2 catabolism by human fecal microflora: partial characterization of ‘dimeric’ intermediates. Arch Biochem Biophys 2010;501:73-8.
44. Sánchez-Patán F, Tabasco R, Monagas M, et al. Capability of Lactobacillus plantarum IFPL935 to catabolize flavan-3-ol compounds and complex phenolic extracts. J Agric Food Chem 2012;60:7142-51.
45. Fan Y, Pedersen O. Gut microbiota in human metabolic health and disease. Nat Rev Microbiol 2021;19:55-71.
46. Vos WM, Tilg H, Van Hul M, Cani PD. Gut microbiome and health: mechanistic insights. Gut 2022;71:1020-32.
47. Firrman J, Liu L, Tanes C, et al. Metabolic analysis of regionally distinct gut microbial communities using an in vitro platform. J Agric Food Chem 2020;68:13056-67.
48. Lessard-Lord J, Lupien-Meilleur J, Roussel C, et al. Mathematical modeling of fluid dynamics in in vitro gut fermentation systems: a new tool to improve the interpretation of microbial metabolism. FASEB J 2024;38:e23398.
49. Cheng H, Zhang D, Wu J, et al. Interactions between gut microbiota and polyphenols: a mechanistic and metabolomic review. Phytomedicine 2023;119:154979.
50. Louis P, Flint HJ. Formation of propionate and butyrate by the human colonic microbiota. Environ Microbiol 2017;19:29-41.
51. Hotchkiss AT Jr, Renye JA Jr, White AK, et al. Cranberry arabino-xyloglucan and pectic oligosaccharides induce lactobacillus growth and short-chain fatty acid production. Microorganisms 2022;10:1346.
52. Rivière A, Selak M, Lantin D, Leroy F, De Vuyst L. Bifidobacteria and butyrate-producing colon bacteria: importance and strategies for their stimulation in the human gut. Front Microbiol 2016;7:979.
53. Belenguer A, Duncan SH, Calder AG, et al. Two routes of metabolic cross-feeding between Bifidobacterium adolescentis and butyrate-producing anaerobes from the human gut. Appl Environ Microbiol 2006;72:3593-9.
54. Rios-Covian D, Gueimonde M, Duncan SH, Flint HJ, de los Reyes-Gavilan CG. Enhanced butyrate formation by cross-feeding between Faecalibacterium prausnitzii and Bifidobacterium adolescentis. FEMS Microbiol Lett 2015;362:fnv176.
55. Moens F, Weckx S, De Vuyst L. Bifidobacterial inulin-type fructan degradation capacity determines cross-feeding interactions between bifidobacteria and Faecalibacterium prausnitzii. Int J Food Microbiol 2016;231:76-85.
56. Özcan E, Rozycki MR, Sela DA. Cranberry proanthocyanidins and dietary oligosaccharides synergistically modulate Lactobacillus plantarum physiology. Microorganisms 2021;9:656.
57. Gao C, Xu L, Montoya L, et al. Co-occurrence networks reveal more complexity than community composition in resistance and resilience of microbial communities. Nat Commun 2022;13:3867.
58. Blanchet FG, Cazelles K, Gravel D. Co-occurrence is not evidence of ecological interactions. Ecol Lett 2020;23:1050-63.
59. Berry D, Widder S. Deciphering microbial interactions and detecting keystone species with co-occurrence networks. Front Microbiol 2014;5:219.
60. Weiss AS, Niedermeier LS, von Strempel A, et al. Nutritional and host environments determine community ecology and keystone species in a synthetic gut bacterial community. Nat Commun 2023;14:4780.
61. Tudela H, Claus SP, Saleh M. Next generation microbiome research: identification of keystone species in the metabolic regulation of host-gut microbiota interplay. Front Cell Dev Biol 2021;9:719072.
62. Wortelboer K, Koopen AM, Herrema H, de Vos WM, Nieuwdorp M, Kemper EM. From fecal microbiota transplantation toward next-generation beneficial microbes: the case of Anaerobutyricum soehngenii. Front Med 2022;9:1077275.
63. Tourlousse DM, Sakamoto M, Miura T, et al. Complete genome sequence of Flavonifractor plautii JCM 32125T. Microbiol Resour Announc 2020;9:e00135-20.
64. Mikami A, Ogita T, Namai F, Shigemori S, Sato T, Shimosato T. Oral administration of Flavonifractor plautii, a bacteria increased with green tea consumption, promotes recovery from acute colitis in mice via suppression of IL-17. Front Nutr 2020;7:610946.
65. Ogita T, Yamamoto Y, Mikami A, Shigemori S, Sato T, Shimosato T. Oral administration of Flavonifractor plautii strongly suppresses Th2 immune responses in mice. Front Immunol 2020;11:379.
66. Mikami A, Ogita T, Namai F, Shigemori S, Sato T, Shimosato T. Oral administration of Flavonifractor plautii attenuates inflammatory responses in obese adipose tissue. Mol Biol Rep 2020;47:6717-25.
67. El Hage R, Hernandez-Sanabria E, Calatayud Arroyo M, Props R, Van de Wiele T. Propionate-producing consortium restores antibiotic-induced dysbiosis in a dynamic in vitro model of the human intestinal microbial ecosystem. Front Microbiol 2019;10:1206.
68. Yoshida N, Emoto T, Yamashita T, et al. Bacteroides vulgatus and Bacteroides dorei reduce gut microbial lipopolysaccharide production and inhibit atherosclerosis. Circulation 2018;138:2486-98.
69. Kumari M, Singh P, Nataraj BH, et al. Fostering next-generation probiotics in human gut by targeted dietary modulation: an emerging perspective. Food Res Int 2021;150:110716.
70. Belzer C, Chia LW, Aalvink S, et al. Microbial metabolic networks at the mucus layer lead to diet-independent butyrate and vitamin B12 production by intestinal symbionts. mBio 2017;8:e00770-17.
71. Perraudeau F, McMurdie P, Bullard J, et al. Improvements to postprandial glucose control in subjects with type 2 diabetes: a multicenter, double blind, randomized placebo-controlled trial of a novel probiotic formulation. BMJ Open Diabetes Res Care 2020;8:e001319.
72. Cani PD, Depommier C, Derrien M, Everard A, de Vos WM. Author Correction: Akkermansia muciniphila: paradigm for next-generation beneficial microorganisms. Nat Rev Gastroenterol Hepatol 2022;19:682.
73. Daniel N, Gewirtz AT, Chassaing B. Akkermansia muciniphila counteracts the deleterious effects of dietary emulsifiers on microbiota and host metabolism. Gut 2023;72:906-17.
74. Bui TPN, Shetty SA, Lagkouvardos I, et al. Comparative genomics and physiology of the butyrate-producing bacterium Intestinimonas butyriciproducens. Environ Microbiol Rep 2016;8:1024-37.
75. Bui TPN, Troise AD, Nijsse B, Roviello GN, Fogliano V, de Vos WM. Intestinimonas-like bacteria are important butyrate producers that utilize Nε-fructosyllysine and lysine in formula-fed infants and adults. J Funct Foods 2020;70:103974.
76. Ahn S, Jin TE, Chang DH, et al. Agathobaculum butyriciproducens gen. nov. sp. nov., a strict anaerobic, butyrate-producing gut bacterium isolated from human faeces and reclassification of Eubacterium desmolans as Agathobaculum desmolans comb. nov. Int J Syst Evol Microbiol 2016;66:3656-61.
77. Song L, Dong X. Hydrogenoanaerobacterium saccharovorans gen. nov., sp. nov., isolated from H2-producing UASB granules. Int J Syst Evol Microbiol 2009;59:295-9.
78. Huang X, Oshima T, Tomita T, Fukui H, Miwa H. Butyrate alleviates cytokine-induced barrier dysfunction by modifying claudin-2 levels. Biology 2021;10:205.
79. Daniel N, Lécuyer E, Chassaing B. Host/microbiota interactions in health and diseases - time for mucosal microbiology! Mucosal Immunol 2021;14:1006-16.
80. Etienne-Mesmin L, Chassaing B, Desvaux M, et al. Experimental models to study intestinal microbes-mucus interactions in health and disease. FEMS Microbiol Rev 2019;43:457-89.
81. Sicard JF, Le Bihan G, Vogeleer P, Jacques M, Harel J. Interactions of intestinal bacteria with components of the intestinal mucus. Front Cell Infect Microbiol 2017;7:387.
82. Sevrin G, Massier S, Chassaing B, et al. Adaptation of adherent-invasive E. coli to gut environment: impact on flagellum expression and bacterial colonization ability. Gut Microbes 2020;11:364-80.
83. Loo YT, Howell K, Chan M, Zhang P, Ng K. Modulation of the human gut microbiota by phenolics and phenolic fiber-rich foods. Compr Rev Food Sci Food Saf 2020;19:1268-98.
84. Gutierrez A, Pucket B, Engevik MA. Bifidobacterium and the intestinal mucus layer. Microbiome Res Rep 2023;2:36.
85. Monteagudo-Mera A, Rastall RA, Gibson GR, Charalampopoulos D, Chatzifragkou A. Adhesion mechanisms mediated by probiotics and prebiotics and their potential impact on human health. Appl Microbiol Biotechnol 2019;103:6463-72.
86. Zhang D, Verstrepen L, De Medts J, et al. A cranberry concentrate decreases adhesion and invasion of Escherichia coli (AIEC) LF82 in vitro. Pathogens 2021;10:1217.
87. Duranti S, Milani C, Lugli GA, et al. Evaluation of genetic diversity among strains of the human gut commensal Bifidobacterium adolescentis. Sci Rep 2016;6:23971.
88. Mulualem DM, Agbavwe C, Ogilvie LA, et al. Metagenomic identification, purification and characterisation of the Bifidobacterium adolescentis BgaC β-galactosidase. Appl Microbiol Biotechnol 2021;105:1063-78.
89. Yu R, Zuo F, Ma H, Chen S. Exopolysaccharide-producing Bifidobacterium adolescentis strains with similar adhesion property induce differential regulation of inflammatory immune response in Treg/Th17 axis of DSS-colitis mice. Nutrients 2019;11:782.
90. Fan L, Qi Y, Qu S, et al. B. adolescentis ameliorates chronic colitis by regulating Treg/Th2 response and gut microbiota remodeling. Gut Microbes 2021;13:1-17.
91. Lin Y, Fan L, Qi Y, et al. Correction: Bifidobacterium adolescentis induces Decorin+ macrophages via TLR2 to suppress colorectal carcinogenesis. J Exp Clin Cancer Res 2023;42:172.
92. Wang B, Kong Q, Cui S, et al. Bifidobacterium adolescentis isolated from different hosts modifies the intestinal microbiota and displays differential metabolic and immunomodulatory properties in mice fed a high-fat diet. Nutrients 2021;13:1017.
93. Qian X, Si Q, Lin G, et al. Bifidobacterium adolescentis is effective in relieving type 2 diabetes and may be related to its dominant core genome and gut microbiota modulation capacity. Nutrients 2022;14:2479.
94. Botta C, Acquadro A, Greppi A, et al. Genomic assessment in Lactobacillus plantarum links the butyrogenic pathway with glutamine metabolism. Sci Rep 2017;7:15975.
95. Aiello A, Pizzolongo F, De Luca L, et al. Production of butyric acid by different strains of Lactobacillus plantarum
Cite This Article
Export citation file: BibTeX | EndNote | RIS
OAE Style
Cattero V, Roussel C, Lessard-Lord J, Roy D, Desjardins Y. Supplementation with a cranberry extract favors the establishment of butyrogenic guilds in the human fermentation SHIME system. Microbiome Res Rep 2024;3:34. http://dx.doi.org/10.20517/mrr.2024.17
AMA Style
Cattero V, Roussel C, Lessard-Lord J, Roy D, Desjardins Y. Supplementation with a cranberry extract favors the establishment of butyrogenic guilds in the human fermentation SHIME system. Microbiome Research Reports. 2024; 3(3): 34. http://dx.doi.org/10.20517/mrr.2024.17
Chicago/Turabian Style
Valentina Cattero, Charlène Roussel, Jacob Lessard-Lord, Denis Roy, Yves Desjardins. 2024. "Supplementation with a cranberry extract favors the establishment of butyrogenic guilds in the human fermentation SHIME system" Microbiome Research Reports. 3, no.3: 34. http://dx.doi.org/10.20517/mrr.2024.17
ACS Style
Cattero, V.; Roussel C.; Lessard-Lord J.; Roy D.; Desjardins Y. Supplementation with a cranberry extract favors the establishment of butyrogenic guilds in the human fermentation SHIME system. Microbiome. Res. Rep. 2024, 3, 34. http://dx.doi.org/10.20517/mrr.2024.17
About This Article
Copyright
Data & Comments
Data
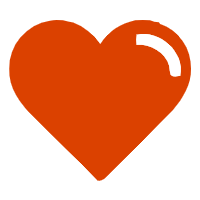
Comments
Comments must be written in English. Spam, offensive content, impersonation, and private information will not be permitted. If any comment is reported and identified as inappropriate content by OAE staff, the comment will be removed without notice. If you have any queries or need any help, please contact us at support@oaepublish.com.