A pilot case-control study on the fecal microbiota of pediatric functional abdominal pain-not otherwise specified and the role of early life stress
Abstract
Background: Gut microbial features and the role of early life stress in pediatric functional abdominal pain-not otherwise specified (FAP-NOS) have never been investigated before. Here, we hypothesize that early life stress is more prevalent in FAP-NOS compared to healthy controls and that fecal microbial profiles and related metabolites differ between groups.
Methods: In an international multicenter case-control study, FAP-NOS patients (n = 40) were compared to healthy controls (n = 55). Stool samples and demographic and clinical data including early life traumatic events and antibiotics treatments were collected from children aged four to twelve years. Fecal microbial profiles were assessed with 16S rRNA gene amplicon sequencing. Microbial metabolite concentrations in fecal supernatant, including short-chain fatty acids and amino acids, were detected via liquid chromatography.
Results: Microbial richness was increased in FAP-NOS compared to healthy controls and microbial composition (unweighted UniFrac) differed between groups. Three distinct amplicon sequencing variants and two distinct species were enriched in FAP-NOS compared to controls, with no observed changes at higher taxonomic levels. No differences in microbial metabolites and early life stress were observed between groups.
Conclusion: The presented hypothesis could not be proven, with no observed differences in occurrence of early life stress, and fecal microbial metabolic profiles between pediatric FAP-NOS and healthy controls. Pediatric FAP-NOS patients exhibited mild differences in the fecal microbial community compared with controls. Further large-scale studies with high-resolution techniques are warranted to address the biological relevance of present observations.
Keywords
INTRODUCTION
Pediatric functional abdominal pain disorders (FAPDs), characterized by visceral hypersensitivity and dysmotility, are affecting approximately 13.5% of children worldwide, with differences observed depending on sex and country[1,2]. According to Rome IV criteria, they include functional dyspepsia, abdominal migraine, irritable bowel syndrome (IBS) and functional abdominal pain not otherwise specified (FAP-NOS)[3]. To date, diagnosis is strictly based on symptoms and exclusion of other medical conditions, with reliable biomarkers still lacking. In general, FAPDs are referred to as disorders of the gut-brain-microbiota interaction, though etiology and pathophysiology are not yet fully understood[2-4]. Genetic, psychosocial, and physiological factors might contribute to the disruption and/or impairment of the gut-brain-microbiota interaction. As such, risk-associated genes and altered gene expressions have been reported[5,6] and a family history of FAPDs has been identified as a risk factor in adults and children alike[2,7]. The latter may not only result from heredity, but also from social and/or environmental factors[2,8].
In early life, where infants undergo rapid development, the gut-brain-microbiota axis is thought to be especially vulnerable to perturbations[2,9]. Thus, early life events, ranging from childhood trauma to early life gastrointestinal infections and antibiotic treatments, have been suggested to be associated with FAPDs[2,3,10,11]. Increasing evidence indicates that gut microbial diversity and composition in adult functional gastrointestinal disorders, especially IBS, differ substantially from healthy controls[12]. Various taxa ratios, including the Bacillota/Bacteroidota and the Faecalibacterium/Bacteroides ratios, were previously suggested as diagnostic markers to differentiate between IBS and controls[12,13]. Pediatric research has also mostly been focusing on IBS, revealing increased relative abundances of genera such as Dorea, Haemophilus, and Flavonifractor in fecal bacterial communities of pediatric IBS patients compared to controls[14,15]. Additionally, a study combining metabolomics and metagenomics revealed enriched microbial metabolic pathways related to amino acid metabolism and depletion of pathways related to carbohydrate metabolism in pediatric IBS compared to controls[15]. Gut microbiota-derived short-chain fatty acids (SCFAs) and neuroactive compounds, such as γ-aminobutyric acid (GABA) and dopamine, are bioactive small molecules that may cross the mucosal layer and exert their effect on underlying neurons and, as such, are thought to contribute to visceral hypersensitivity in FAPDs[2].
Of all FAPD subgroups, FAP-NOS and IBS are most similar, with the only discriminative criteria being the lack of association of abdominal pain with bowel movement and no change in the nature of stool in FAP-NOS[3]. Nevertheless, FAP-NOS and IBS have been shown to exhibit similar clinical and psychological features[16]. To our knowledge, there are no studies describing the gut microbial composition and associated metabolites in pediatric FAP-NOS and evaluating the occurrence of early life events (i.e., early life antibiotics treatment and traumatic events). Here, we hypothesize that the occurrence of early life events is more frequent in FAP-NOS compared to healthy controls and that fecal microbial profile and related metabolites differ distinctly between FAP-NOS and controls.
MATERIALS AND METHODS
Study population
In an international multicenter study, children (with FAP-NOS or healthy) between four to twelve years of age were recruited at the Children’s Hospital Zürich (Zürich, Switzerland) and Children’s Hospital Zagreb (Zagreb, Croatia). Diagnosis of children suffering from FAP-NOS was performed by pediatric specialists in accordance with Rome IV criteria[3]. Written informed consent was obtained from the caregivers. Ethical approval was given by the committees in Zurich (2018-00523) and Zagreb (02-23/31-2-18) for the purpose of processing biological samples and health-related data as performed in this study.
Exclusion criteria for all subjects included: (i) history of severe mental and/or psychiatric illness such as attention deficit hyperactivity disorder or autism; (ii) obesity or anorexia (BMI > P97; BMI < P3); and (iii) diabetes type 1. In addition, for healthy controls, the following exclusion criteria applied: (iv) abdominal pain; (v) intestinal infection or diarrhea in the last three months; (vi) non-regular bowel movements; (vii) intestinal diseases; (viii) lactose intolerance; and (ix) celiac disease.
Caregivers were asked about factors potentially affecting the microbiota of participants, including delivery mode, infant diet, preterm birth, current special food habits [i.e., low fermentable oligosaccharides, disaccharides, monosaccharides and polyols (FODMAP), vegetarian, vegan, gluten-free, lactose-free, or low-lactose diet], and consumption of alcohol and/or smoking. In addition, administration of loperamide, proton pump inhibitors (PPI), polyethylene glycol (PEG), probiotics, and antibiotics in the last three months was registered [Supplementary Materials]. Caregivers were asked about children’s stress in the first three years of life. As such, general early life events using the Life Event Scale[17] adapted for early life, and early life antibiotics treatments were inquired [Supplementary Materials]. Early life traumatic events were assessed, using caregiver reports of the Young Children Posttraumatic Stress Disorder (PTSD) Checklist (YCPC)[18] or the University of California at Los Angeles (UCLA) PTSD Reaction Index for DSM-IV[19,20] modified to register the age during which specific events occurred. For subsequent data analysis, early life traumatic events were considered positive if at least one event was experienced by the participants, as indicated in YCPC or UCLA-PTSD caregiver reports in the first three years of life. Finally, FAP-NOS patients were asked to rate pain severity (from one to ten) using a visual analog scale (VAS) at the time of FAP-NOS diagnosis, and the duration of symptoms (in months) was registered.
Participants collected stool specimens at home using the provided stool catcher and sample tube. Subsequently, they stored the sample at -20 °C until transportation to research facilities (one week at the utmost). Transportation of specimens was carried out by trained professionals using a cooling container. To ensure the stability of microbial and metabolic profiles during long-term storage, the specimens were stored at -80 °C until analysis.
Fecal dry weight and pH quantification
To determine dry weight, fecal sample aliquots of 200 mg wet weight (ww) were dried at 80 °C in a ventilated oven (VWR International AG, Dietikon, Switzerland) until stable weight was achieved (no weight change within 24 h). Fecal pH was measured as described previously[21]. In short, fecal slurries were prepared by homogenization of samples in double distilled water at a 1:10 ratio (ww/v) and pH was measured using a pH electrode (Metrohm Schweiz AG, Zofingen, Switzerland).
Bacterial metabolites quantification
Fecal supernatant was prepared aerobically by homogenizing samples in 100 mM HClO4 at a 1:3 ratio
Metabarcoding of fecal bacterial community and enterotype assignment
Bacterial DNA was extracted from stool specimens using the FastDNA Spin kit for soil (MP Biomedicals, Illkirch, France) according to the manufacturer’s instructions.
Quantitative PCR (qPCR) was performed to assess the total 16S rRNA gene copies per sample, using primers Eub338F (5’-ACTCCTACGGGAGGCAGCAG-3’) and Eub518R (5’-ATTACCGCGGCTGCTGG-3’). Reactions were carried out in technical triplicates using the Roche Light Cycler 490 (Hoffmann-La Roche, Basel, Switzerland). Each reaction mixture consisted of 5 µL SensiFast SYBR No-ROX mix (Labgene Scientific Instruments, Châtel-Saint-Denis, Switzerland), 0.5 µL each of forward and reverse primer (10 µM, Microsynth, Balgach, Switzerland), 3 µL nuclease-free water, and 1 µL of diluted DNA template. qPCR was carried out by applying 3 min of initial denaturation at 95 °C, followed by 40 cycles of 5 seconds at 95 °C and 30 s at 65 °C. Subsequent melting curve analysis was performed from 65 to
The V4 16S rRNA gene region was amplified using primers 515F (5’-GTGCCAGCMGCCGCGGTAA-3’) and 806R (5’-GGACTACHVGGGTWTCTAAT-3’), followed by tag-encoded MiSeq-based (Illumina, CA, USA) high throughput sequencing using an Illumina MiSeq System v2 including a flow cell with 2 × 250-bp paired-end Nextera chemistry supplemented with 10% of PhiX as sequencing control.
Raw Illumina sequencing data were processed using the R package metabaRpipe[23]. In short, Atropos[24] was used to remove adaptors and V4 primers, and the DADA2 pipeline[25] was used to construct Amplicon Sequencing Variants (ASV). Taxonomic assignment was performed using DADA2-formatted SILVA reference base (v138). Raw sequences were deposited on the European Nucleotide Archive at EMBL-EBI under the accession number PRJEB57328. Enterotypes were identified based on total sequences (i.e., controls and FAP-NOS). For reference-based identification of enterotypes, processed microbiota sequences were compared to a reference space of adult gut microbiota from the Human Microbiome Project (HMP)[26] and Metagenomics of the Human Intestinal Tract project (MetaHIT)[27] according to Arumugam et al.[28]. For de novo identification of enterotypes, a partitioning around medoid (PAM) clustering based on relative genus abundance using Jensen-Shannon divergence was performed[28].
Data analysis, visualization, and statistical analysis
All data and statistical analyses were performed in R (v4.2.0)[29] using the packages speedyseq (v0.5.3.9018)[30], vegan (v2.5.7)[31], ape (v5.5)[32], ampvis2 (v2.7.9)[33], DivComAnalyses (v0.9)[34], and Maaslin2 (v1.10.0)[35]. Prediction of functional metabolic potential was performed with Phylogenetic Investigation of Communities by Reconstruction of Unobserved States (PICRUSt2) software[36], calculating MetaCyc pathway abundances[37]. Prediction accuracy was evaluated using the abundance-weighted nearest sequenced taxon index (NSTI) to summarize the extent to which ASVs in a sample are related to reference 16S rRNA genes.
For the calculation of significant differences between the two groups, a Wilcoxon rank-sum test was used for continuous variables and Fisher’s exact test was used for categorical variables. To control for false discovery rate (FDR) during multiple testing, the Benjamini-Hochberg method was applied. Fecal sample characteristics such as stool consistency (related to fecal dry weight and water content) and pH have been associated with fecal microbial features (i.e., diversity and abundance of taxa)[38,39]. Thus, potential confounding effects of fecal characteristics, and demographic and clinical data were addressed in multiple linear regression models [Supplementary Material and Methods] The significance level was set to P ≤ 0.05. Data visualization was done in R using ggplot2 (v3.3.5)[40] and ComplexHeatmap (v2.13.1)[41]. Mean or median values were stated, including standard deviation (sd) or interquartile range (iqr), in brackets, respectively. In boxplots, outliers are indicated and defined as values greater than 1.5 times the iqr over the 75th percentile and values smaller than 1.5 iqr under the 25th percentile.
RESULTS
Patient characteristics
In total, 123 children were recruited, of whom some were excluded due to incomplete data sets (n = 4), smoking (n = 1), detected parasites (n = 2), being part of siblings (n = 6), and antibiotics treatment in the last 6 months (n = 15). This resulted in 55 control and 40 FAP-NOS participants [Table 1 and Supplementary Data 1]. Age distribution significantly differed between groups, with children diagnosed with FAP-NOS being significantly older [8.6 (sd: 2.3); P < 0.05; FDR-adjusted] than in controls [7.1 (sd: 2.3); Table 1 and Supplementary Figure 1A]). Though not significant (P > 0.05; FDR-adjusted), FAP-NOS group showed a higher percentage of females (62.5%) compared to controls (56.4%). FAP-NOS patients followed special food habits significantly more often (27.5%; P < 0.001; FDR-adjusted) than participants of control group (0%), mainly by maintaining a lactose-free or low-lactose diet [Table 1]. Ingestion of medication such as PPI and/or PEG in the last three months was increased in FAP-NOS (15%) compared to control participants (1.8%), though this was not significant (P > 0.05; FDR-adjusted; Table 1). Occurrence of general early life events did not significantly differ between FAP-NOS and control groups (P > 0.05; FDR-adjusted), with similar values for FAP-NOS and control groups [Supplementary Table 1]. Similarly, no differences in individual early life trauma events were detected between groups (P > 0.05; FDR-adjusted; Supplementary Table 2), with comparable occurrence of early life trauma in FAP-NOS (12.5%) and controls (12.7%; Table 1). No significant differences in early life antibiotics treatment were detected (P > 0.05; FDR-adjusted; Table 1), with lower trends of treatment in FAP-NOS (57.5%) compared to controls (67.3%).
Group demographics and clinical data
Healthy controls | FAP-NOS | P-value* | |
Group size (n) | 55 | 40 | |
Age (years): mean (sd) | 7.1 (2.3) | 8.6 (2.3) | 0.015 |
Sex (%): female/male | 56.4/43.6 | 62.5/37.5 | ns |
Country (%): Croatia/Switzerland | 50.9/49.1 | 27.5/72.5 | ns |
Mode of delivery (%): caesarian/vaginal | 40.0/60.0 | 37.5/62.5 | ns |
Main infant diet in the first 4 months of life (%): breast-fed/formula-fed/both | 89.1/10.9/0 | 70.0/22.5/7.5 | ns |
Preterm birth (%) | 10.9 | 12.5 | ns |
Early life antibiotics treatment (in first 3 years of life; %) | 67.3 | 57.5 | ns |
Early life traumatic events (in first 3 years of life; %) | 12.7 | 12.5 | ns |
Probiotics treatment in last 3 months (%) | 27.3 | 45.0 | ns |
Special dietary habits (%): low FODMAP/vegetarian/vegan/lactose specific/gluten-free | 0/0/0/0/0 | 0/2.5/0/25.0/0 | 0.0003 |
Medication in last 3 months (%): PEG/PPI/loperamide | 1.8/0/0 | 10.0/5.0/0 | ns |
Pain severity (VAS)†: mean (sd) | na | 6.6 (1.9) | na |
Duration of symptoms (month)††: mean (sd) | na | 28.7 (21.0) | na |
To evaluate synergistic effects of demographic and clinical data on data distribution, a multiple factor analysis was performed. This revealed a significant difference in dispersion (P = 0.001; R2 = 0.125, Supplementary Figure 1) between FAP-NOS and control groups, indicating that healthy controls are more similar amongst each other in terms of demographic and clinical data than FAP-NOS participants. In addition, the centroid distance between FAP-NOS and control groups was significant (P = 0.001,
Characteristics of fecal samples
Fecal pH was identical in FAP-NOS and controls, with mean values of 7.2 (sd: 0.6) [Table 2 and Supplementary Data 1]. Fecal dry weight was similar between FAP-NOS and control groups, with approximately 30% of dry fraction in fecal samples of both groups (P > 0.05; FDR-adjusted; Table 2). Likewise, bacterial load - based on a total of 16S rRNA gene copies per dry weight - was comparable between groups, with mean values of 1012 copies for both groups [Table 2]. To evaluate a potential difference in general microbiota type between groups, identification of enterotypes was performed. Reference-based enterotype assignment resulted in 67% of samples showing major compositional dissimilarity to the reference space based on the adult microbiota of HMP[26] and MetaHIT[27]. Therefore,
Characteristics of fecal samples
Healthy controls | FAP-NOS | P-value* | |
Fecal pH: mean (sd) | 7.2 (0.6) | 7.2 (0.6) | ns |
Fecal dry weight (%): mean (sd) | 27.9 (6.8) | 28.4 (6.9) | ns |
Log10 16S rRNA gene copies per g dry weight: mean (sd) | 12.05 (0.40) | 11.86 (0.43) | ns |
De novo enterotype (%): enterotype 1/enterotype 2 | 60/40 | 75/25 | ns |
Increased fecal bacterial richness in FAP-NOS group compared to controls
Alpha diversity - diversity within the community - was investigated at the ASV and species levels via evaluation of gut microbiota richness (observed ASVs or observed species; number of ASVs or species in a sample), and evenness (Pielou’s index, a measure indicating how evenly ASVs or species are distributed in a sample). Potential confounding effects of demographic and clinical data [Table 1], and fecal characteristics
At the ASV level, gut microbiota richness was significantly higher in FAP-NOS patients compared to controls (P < 0.0001; R2 = 0.263), with medians of observed ASVs being 390 (iqr: 50) and 332 (iqr: 45), respectively [Figure 1A]. Similarly, probiotics treatment in the last three months significantly (P < 0.05;
Figure 1. Alpha diversity at ASV level. Comparison of (A) richness and (E) evenness between FAP-NOS and control groups; Visualization of the effect of (B) probiotics treatment in the last three months (FALSE vs. TRUE); (C) fecal dry weight; and (D) fecal pH on gut microbial richness. Boxplot with box elements showing upper quantile, lower quantile, and median. Whiskers extend from the upper/lower quantile to ± 1.5 iqr range or the highest/lowest value. Outliers are indicated as black points. Points in the scatterplot display values for individual microbiota. Regression line is based on multiple linear regression model, and 95% confidence interval is displayed. All significances were calculated using a multiple linear regression model [Supplementary Table 3]. *P < 0.05; ****P < 0.0001. FAP-NOS: Functional abdominal pain-not otherwise specified; ns: not significant; ASV: amplicon sequence variant; iqr: interquartile range.
Similar results were observed at the species level, with higher richness in FAP-NOS compared to controls
Mild differences in fecal microbial composition between FAP-NOS and control groups
Beta diversity - diversity between communities – was investigated at the ASV and species levels via evaluation of the UniFrac distance. This beta diversity metric includes phylogenetic (i.e., evolutionary relationship) information. Unweighted (qualitative) UniFrac distance served as a read-out for gut microbial composition, and weighted (quantitative) UniFrac distance served as a read-out for gut microbiota structure. Potential confounding effects of demographic and clinical data [Table 1], and fecal characteristics [Table 2] on beta diversity were addressed in a linear model fitted to various distance metrics.
At the ASV level, gut microbiota composition based on unweighted UniFrac was significantly different between FAP-NOS and controls (P = 0.001), though with low explanatory power (R2 = 0.020; Figure 2A). Country, de novo enterotype, fecal dry weight, and fecal pH all explained a similar amount of variability observed in unweighted UniFrac (0.014 ≤ R2 ≤ 0.020; Figure 2 and Supplementary Table 5). Gut microbiota structure based on weighted UniFrac did not differ significantly between FAP-NOS and control groups
Figure 2. Beta diversity metrics at ASV level. Comparison of (A) unweighted and (B) weighted UniFrac between FAP-NOS (orange triangle) and control groups (blue circle); Visualization of effect of (C) country, (D) fecal dry weight, (E) enterotype, and (F) fecal pH on unweighted UniFrac; Visualization of effect of (G) enterotype, (H) bacterial load, and (I) fecal pH on weighted UniFrac. Small symbols display individual microbiota, large symbols display centroids, and ellipses indicate 95% confidence intervals. All significances were calculated using a linear model fitted to various distance metrics [Supplementary Tables 5 and 6]. FAP-NOS: Functional abdominal pain-not otherwise specified; E1: enterotype 1; E2: enterotype 2; ASV: amplicon sequence variant.
Similar results were observed at the species level, with significant differences between FAP-NOS and controls for unweighted UniFrac (microbiota composition; P < 0.002; R2 = 0.029) and no differences in weighted UniFrac (microbiota structure; P > 0.05; Supplementary Figure 9). However, at the species level, fecal dry weight, fecal pH, and bacterial load had no effect on observed variability [Supplementary Figure 9, Supplementary Tables 7 and 8].
To further investigate microbial composition and structure, common taxa ratios were explored and fitted to various demographic and clinical data, and fecal characteristics. Both the Bacillota/Bacteroidota and the Faecalibacterium/Bacteroides ratio did not significantly differ between FAP-NOS and control groups
To identify specific taxa that are differentially abundant in FAP-NOS compared to controls, a differential abundance analysis was performed by applying a linear model. Three significantly (P < 0.05; FDR-adjusted) differential abundant ASVs between FAP-NOS and controls were detected, and all increased in FAP-NOS in the range of 2 to 3 log2 fold-changes. The relative abundance of these ASVs was low, with individual microbiota values ranging between 0% and 2.5% [Figure 3A]. ASV0873, belonging to an unknown species of the RF39 order, was present in 36.7% and 65.0%, and ASV2480, belonging to an unknown species of the genus Christensenellaceae R-7 group, was present in 34.5% and 72.5% of control and FAP-NOS samples, respectively. ASV0267, identified as a Bifidobacterium bifidum ASV, was present in all tested samples.
Figure 3. Differential abundance of ASVs and species in FAP-NOS vs. controls. Abundance log2 fold-changes and relative abundance of significantly (P < 0.05) increased (A) ASVs and (B) species in FAP-NOS compared to controls. Points display individual log2 fold-changes and bars display standard errors. Boxplot with box elements showing upper quantile, lower quantile, and median. Whiskers extend from the upper/lower quantile to ± 1.5 iqr or the highest/lowest value. Colored points display values for individual microbiota, while outliers are indicated as black points. Significances were calculated using a log2 transformed linear model with FDR correction for multiple testing. *P < 0.05. FAP-NOS: Functional abdominal pain-not otherwise specified; ASVs: amplicon sequence variants; iqr: interquartile range; FDR: false discovery rate.
Sarcina ventriculi and Bifidobacterium bifidum were both significantly (P < 0.05; FDR-adjusted) increased in FAP-NOS compared to controls, with observed log2 fold-changes of 1.5 and 1.4, respectively. Overall, the relative abundance of Sarcina ventriculi was low, with the highest observed value of 0.4% [Figure 3B]. Sarcina ventriculi was present in 16.4% of control and 57.5% of FAP-NOS samples, while B. bifidum was present in all tested samples. The highest observed abundance of B. bifidum was 5.2%, with median values of 1.3% (iqr: 2.0) and 0.1% (iqr: 1.5) for FAP-NOS and controls, respectively.
No significantly differentially abundant taxa (P > 0.05; FDR-adjusted) between FAP-NOS and controls were identified at higher taxonomic levels [Supplementary Data 2]. The abundance of ASVs and/or species was different when comparing de novo enterotypes (E1 vs. E2), or countries (Switzerland vs. Croatia), or probiotics treatment status (probiotic intake: FALSE vs. TRUE) [Figure 4]. Bacterial load and fecal dry weight both negatively correlated with specific ASVs or species, respectively [Figure 4]. Pain severity and duration of symptoms did not correlate with abundance of any taxa (P > 0.05; FDR-adjusted). A subsequent analysis of FAP-NOS microbiota alone was performed to examine the potential effect of age, given the broad age range in the present study. No significant effects (P > 0.05) of age on taxa abundances at genus and family level were observed.
Figure 4. Differential abundance of ASVs and species in demographic and clinical data and fecal characteristics. Heatmaps of all significantly (P < 0.05) differentially abundant (A) ASVs and (B) species in demographic and clinical data and fecal characteristics. Log2 fold-change per unit of continuous variables or compared to a corresponding categorical variable is displayed. Additional taxonomic information is indicated at the family level. Significances were calculated using a log2 transformed linear model with FDR correction for multiple testing. FAP-NOS: Functional abdominal pain-not otherwise specified; E2: enterotype 2; ASVs: amplicon sequence variants; FDR: false discovery rate.
No differences in fecal microbial metabolites between FAP-NOS and control groups
The metabolic profiles of FAP-NOS and controls [Supplementary Data 3] were evaluated and compared under the inclusion of various demographic and clinical data and fecal characteristics. No significant differences (P > 0.05; FDR-adjusted) between FAP-NOS and controls in fecal total and specific organic acid, amine, amino acid, and ammonia concentrations were detected, including 34 different metabolites, i.e., succinate, lactate, formate, acetate, propionate, isobutyrate, butyrate, isovalerate, valerate, alanine, ammonia, arginine, asparagine, aspartic acid, cadaverine, dopamine, GABA, glutamic acid, glutamine, glycine, histidine, isoleucine, lysine, methionine, ornithine, phenylalanine/leucine, proline, putrescine, serine, tryptophan, tyramine, tyrosine, valine, and phenylethylamine [Supplementary Figures 12 and 13]. However, country, fecal dry weight, fecal pH, de novo enterotype, and main infant diet had a significant effect on the fecal metabolite profile [Supplementary Figure 14].
To further elaborate on fecal metabolic potential, a preliminary explorative prediction of bacterial metabolic pathways based on 16S rRNA microbial profiling was performed [Supplementary Data 4]. Abundance-weighted average NSTI scores were not significantly different between FAP-NOS and controls (P > 0.05). Individual microbiota values ranged from 0.03 to 0.18, indicating that, on average, prediction was performed based on taxa with 97% to 82% similarity. In total, 349 distinct metabolic pathways were identified. FAP-NOS and control groups did not significantly differ (P > 0.05; FDR-adjusted) in any predicted metabolic pathways. Significantly differentially abundant metabolic pathways were observed between different de novo enterotypes. Fecal dry weight, fecal pH, and bacterial load significantly correlated with different metabolic pathways [Supplementary Figure 15]. Pain severity and duration of symptoms did not correlate with abundance of any pathway (P > 0.05; FDR-adjusted).
DISCUSSION
Of all FAPD subgroups, FAP-NOS is the least characterized, and associated biomarkers have never been reported before. In this pilot-case-control study, for the first time, the microbial community and associated metabolites in pediatric FAP-NOS patients are addressed and the occurrence of early life events - defined as early life traumatic events and antibiotics treatment - is evaluated.
Early life stress has previously been suggested as a risk factor for the development of FAPDs, as the first years of life pose a critical developmental window. Early life events may thus have lasting consequences and may alter pain processing later in life[2,9,42]. On the contrary, here we showed no differences in experienced traumatic early life events and early life antibiotics treatment in FAP-NOS compared to controls. In addition, no significant associations of traumatic early life events and/or early life antibiotics treatment with fecal microbial diversity, composition, and structure, including associated metabolites, were observed. However, we repeatedly show a significant effect of country, i.e., Switzerland vs. Croatia, and fecal characteristics, i.e., fecal pH, fecal dry weight, bacterial load, and de novo enterotype, on the fecal bacterial community. Contrary to previously observed three enterotypes in school-aged children, dominated by Bacteroides, Prevotella or Bifidobacterium[43], and enterotypes identified in adults i.e., Bacteroides, Prevotella or Ruminococcus dominated[44], here we identified only two de novo enterotypes enriched in 12 or 20 distinct genera with concurring difference in metabolic profiles and predicted metabolic pathways. More specifically, enterotype 2 was enriched in Bacteroides and Alistipes species, which have previously been associated with amino acid degradation[45,46]. In line, enterotype 2 was depleted in amino acids (among others, valine, isoleucine, proline, and alanine) compared with enterotype 1, and depleted in predicted metabolic pathways for valine and isoleucine biosynthesis. Concurringly, for enterotype 2, the genus Bacteroides significantly negatively correlated with alanine and proline, while the genus Alistipes significantly negatively correlated with proline concentrations.
We showed a significant increase in microbiota richness in FAP-NOS compared to controls while controlling for demographic and clinical data, and fecal characteristics. Previous research has illustrated the increase in microbial richness with increasing luminal pH[39], which we confirm here. However, precise mechanisms of pH on microbial features are not yet known. In the adult population, microbiome richness is associated with health due to improved community resilience promoted by increased efficiency and redundancy[47], while decreased richness is associated with various diseases (e.g., diabetes, inflammatory bowel diseases, obesity)[48,49]. In the rarely available studies referring to pediatric FAPDs patients, inconsistent results were reported, with microbiota alpha diversity metrics in patients being increased, decreased or similar compared to the controls[13,14,50]. These inconsistent results might be explained by different FAPD subgroups, distinct dietary preferences, e.g., high fiber intake[51], or by potential underlying microbiota confounders. Indeed, a strong association linking increased richness with low Bacteroidota and high Bacillota abundance was previously shown[52]. Nevertheless, in our study, the Bacillota/Bacteroidota ratio showed no significant difference between FAP-NOS and controls, thereby further strengthening the differences in alpha diversity observed.
Multi-omics studies in pediatric and adult IBS patients alike suggest a correlation between microbial features and symptom severity[15,53]. However, no correlation between pain intensity and duration of symptoms with fecal microbial community and associated metabolites was observed in the present study. We showed a significant difference in fecal bacterial composition (unweighted UniFrac) and no difference in fecal bacterial structure (weighted UniFrac) between FAP-NOS and healthy controls. However, the degree of variation explained in bacterial composition was low and similar to that of the country, de novo enterotype, fecal dry weight, and fecal pH, thus questioning the clinical relevance of those differences as biomarkers. The Faecalibacterium/Bacteroides ratio has previously been suggested as a biomarker for FAPDs, with lower ratios observed in FAPDs patients[13]. However, in the present study, no difference in this taxa ratio was observed between FAP-NOS and controls. Instead, we showed enrichment of Sarcina ventriculi and B. bifidum in pediatric FAP-NOS compared to controls, with no observed changes at higher taxonomic levels. Sarcina ventriculi is a gut commensal found in fecal samples of healthy adults mainly following a vegetarian diet[54]. However, the extent of pathogenicity of Sarcina ventriculi is still discussed. In rare cases, Sarcina ventriculi infections have been reported, with the main isolation location being the gastroesophageal tract. Interestingly, abdominal pain was one of the major comorbidities observed[55]. In general, high counts of Bifidobacterium species are associated with healthy microbiota and probiotic formulations containing specific strains of this group have also been suggested as a treatment for pediatric IBS, alleviating abdominal pain[56,57]. In adult IBS patients, treatment with a specific B. bifidum strain resulted in a significant decrease in pain symptoms compared to placebo group[58]. However, the sequence similarity of the 16S rRNA genes of some Bifidobacterium groups has been described as being exceptionally high (99% similarity)[59,60]. Together with the notion that the V4 region of the 16S rRNA gene may not always be able to differentiate between species[61], caution is required when interpreting these results. We show an association between probiotic intake in the last three months and an increase in B. animalis in overall fecal microbiota. Given the high sequence similarities of the 16S rRNA genes and the higher, though non-significant, probiotic intake in the FAP-NOS group, an influence of probiotics on the observed results of Bifidobacterium abundances cannot be excluded. High-resolution techniques, such as shotgun metagenomic sequencing, are thus warranted to further shed light on specific Bifidobacterium species changes between FAP-NOS and healthy controls. Given the lack of differences in bacterial abundance at higher taxonomic levels (i.e., genus and family level) and the relatively small amount (2%) of variation explained by health status (i.e., FAP-NOS vs. control), our data suggest mild alterations in FAP-NOS microbiota compared to controls.
Here, we showed that fecal organic acid and amino acid composition, including SCFAs and neuroactive compounds, did not significantly differ between FAP-NOS patients and healthy children. In addition, no differences were observed in predicted bacterial metabolic pathways. Similarly, a study comparing volatile organic acids in fecal samples from pediatric IBS, FAP-NOS and controls did not show differences between groups[62]. Nevertheless, a multi-omics study in pediatric IBS patients revealed a trend toward a decrease or increase in predicted metabolic pathways and enzymes involved in microbial carbohydrate degradation or amino acid metabolism, respectively[15]. In addition, formate, lactate, tyrosine, lysine, and leucine have been shown to be enriched in pediatric IBS patients compared to healthy controls[63]. Given that before-mentioned studies focus on diarrhea or constipation-predominant IBS with associated differences in colon transit time and thus fermentation time, it seems plausible that no effects are seen in FAP-NOS where altered colon transit time is not present[3]. The metabolic pathway prediction with PICRUSt2 has limitations, such as bias resulting from a strong dependency on reference genomes and the inability to address strain-specific functionality[36]. Thus, the present PICRUSt2 analysis is a preliminarily explorative prediction and would need to be verified with shotgun metagenomics, metabolomics, and/or RNA sequencing. Specific alterations of gut microbial functionality in FAP-NOS patients may be revealed only when high-resolution techniques are applied.
It must be noted that the present study included children from a relatively wide age range of 4 to 12 years. Based on fecal microbial richness, previous research has suggested that the maturation of the gut microbiota extends beyond the fifth year of life[64], suggesting potential differences between different ages in the present study. Even though we show a significant difference in terms of age between children recruited for the control and FAP-NOS groups, in subsequent linear regression models, there was no association between age and different microbial features (i.e., alpha and beta diversity, taxa abundances, metabolites, and predicted metabolic pathways). In addition, no age-related variations in FAP-NOS patients were observed.
The present study has several limitations. Firstly, although fecal samples have their advantages as a non-invasive form of investigation of the gut microbiota, they serve only as a proxy of the gut microbial community present in different regions of the colon and its metabolic potential[65]. In association with nutrient availability (carbohydrates vs. proteins) and pH gradient, the gut microbial community and functionality differ across the lateral (mucosa to lumen) and longitudinal (proximal to distal) axis of the colon[66]. Observed changes in fecal microbial community or lack thereof may thus not be directly extrapolated to the entire gut microbial community. Thus, the biological relevance of observed changes needs to be addressed in future studies. Secondly, the present study is a pilot study with a relatively small sample size. Only a large-scale study would allow for further stratification of the heterogenous FAP-NOS group. Even though the present study addressed various potential confounders, Falony et. al have shown that up to 69 clinical and questionnaire-based covariates could be associated with the gut microbial composition of healthy individuals[67]. Likewise, the fecal metabolome is affected by various environmental and medical factors such as physical fitness and specific diets[68,69]. Future studies should thus address additional confounders such as detailed food habits. In the present study, early life traumatic events were evaluated using validated PTSD caregiver reports. However, there are less obvious experiences, such as unstable families or poverty, that pose a substantial burden on children. In general, there is still considerable ambiguity concerning the definition of early life stress itself and aspects including parental care or dysfunctional relationship between parents and children may not be captured by caregiver reports[70].
Conclusively, no differences in the occurrence of early life stress and fecal metabolic profiles between
DECLARATIONS
Acknowledgments
We thank Alfonso Die and Adam Krzystek for assistance with liquid chromatography analysis. We thank Rebekka Koller, Vanessa Rüger, Sara Sila, and Ivana Trivic for participant recruitment and sample collection. We thank Helene Werner for sharing her expertise on questionnaire selection. Illumina sequencing data were generated in collaboration with the Genetic Diversity Centre (GDC), ETH Zürich. We thank Julia Isenring and Florentin Constancias for their discussions on data analysis.
Authors’ contributions
Designed the study: Braegger C, Lacroix C, Otaru N, Pugin B
Supervised data collection in corresponding centers: Braegger C, Hojsak I
Performed the experiments: Otaru N, Plüss S
Performed the data analysis: Otaru N
Drafted the manuscript: Lacroix C, Otaru N, Pugin B
All authors critically reviewed the manuscript and approved the submitted version.
Availability of data and materials
Raw sequences generated and analyzed during the current study are available in the European Nucleotide Archive repository under accession number PRJEB57328 (https://www.ebi.ac.uk/ena/browser/view/PRJEB57328).
Financial support and sponsorship
This work was supported by its own resources (ETH Zürich and University Children’s Hospital Zürich).
Conflicts of interest
Christophe Lacroix is an Editorial Board member of the journal Microbiome Research Reports, while the other authors have declared that they have no conflicts of interest.
Ethical approval and consent to participate
Ethical approval was given by the committees in Zurich (2018-00523) and Zagreb (02-23/31-2-18) for the purpose of processing biological samples and health-related data as performed in this study. Written informed consent was obtained from the caregivers for the purpose of processing biological samples and health-related data as performed in this study.
Consent for publication
Not applicable.
Copyright
© The Author(s) 2024.
Supplementary Materials
REFERENCES
1. Korterink JJ, Diederen K, Benninga MA, Tabbers MM. Epidemiology of pediatric functional abdominal pain disorders: a meta-analysis. PLoS One 2015;10:e0126982.
2. Thapar N, Benninga MA, Crowell MD, et al. Paediatric functional abdominal pain disorders. Nat Rev Dis Primers 2020;6:89.
3. Hyams JS, Di Lorenzo C, Saps M, Shulman RJ, Staiano A, van Tilburg M. Functional disorders: children/adolescents. Gastroenterology 2016;150:1456-68.e2.
4. Drossman DA, Hasler WL. Rome IV-functional GI disorders: disorders of gut-brain interaction. Gastroenterology 2016;150:1257-61.
5. Willits AB, Grossi V, Glidden NC, Hyams JS, Young EE. Identification of a pain-specific gene expression profile for pediatric recurrent abdominal pain. Front Pain Res 2021;2:759634.
7. Levy RL, Jones KR, Whitehead WE, Feld SI, Talley NJ, Corey LA. Irritable bowel syndrome in twins: heredity and social learning both contribute to etiology. Gastroenterology 2001;121:799-804.
8. Zeevenhooven J, Rutten JMTM, van Dijk M, Peeters B, Benninga MA. Parental factors in pediatric functional abdominal pain disorders: a cross-sectional cohort study. J Pediatr Gastroenterol Nutr 2019;68:e20-6.
9. Jena A, Montoya CA, Mullaney JA, et al. Gut-brain axis in the early postnatal years of life: a developmental perspective. Front Integr Neurosci 2020;14:44.
10. Bradford K, Shih W, Videlock EJ, et al. Association between early adverse life events and irritable bowel syndrome. Clin Gastroenterol Hepatol 2012;10:385-90.e1.
11. Uusijärvi A, Bergström A, Simrén M, et al. Use of antibiotics in infancy and childhood and risk of recurrent abdominal pain--a Swedish birth cohort study. Neurogastroenterol Motil 2014;26:841-50.
12. Duan R, Zhu S, Wang B, Duan L. Alterations of gut microbiota in patients with irritable bowel syndrome based on 16S rRNA-targeted sequencing: a systematic review. Clin Transl Gastroenterol 2019;10:e00012.
13. Abomoelak B, Pemberton V, Deb C, et al. The gut microbiome alterations in pediatric patients with functional abdominal pain disorders. Microorganisms 2021;9:2354.
14. Saulnier DM, Riehle K, Mistretta TA, et al. Gastrointestinal microbiome signatures of pediatric patients with irritable bowel syndrome. Gastroenterology 2011;141:1782-91.
15. Hollister EB, Oezguen N, Chumpitazi BP, et al. Leveraging human microbiome features to diagnose and stratify children with irritable bowel syndrome. J Mol Diagn 2019;21:449-61.
16. Rutten JM, Benninga MA, Vlieger AM. IBS and FAPS in children: a comparison of psychological and clinical characteristics. J Pediatr Gastroenterol Nutr 2014;59:493-9.
17. Schmidt SJ, Barblan LP, Lory I, Landolt MA. Age-related effects of the COVID-19 pandemic on mental health of children and adolescents. Eur J Psychotraumatol 2021;12:1901407.
18. Scheeringa M. Young child PTSD checklist. 2013. Available from: https://www.michaelscheeringa.com/uploads/1/2/0/2/120202234/ycpc_2014_5_23.pdf. [Last accessed on 4 Jun 2024].
19. Landolt MA, Schnyder U, Maier T, Schoenbucher V, Mohler-Kuo M. Trauma exposure and posttraumatic stress disorder in adolescents: a national survey in Switzerland. J Trauma Stress 2013;26:209-16.
20. Steinberg AM, Brymer MJ, Kim S, et al. Psychometric properties of the UCLA PTSD reaction index: part I. J Trauma Stress 2013;26:1-9.
21. Paganini D, Uyoga MA, Kortman GAM, et al. Prebiotic galacto-oligosaccharides mitigate the adverse effects of iron fortification on the gut microbiome: a randomised controlled study in Kenyan infants. Gut 2017;66:1956-67.
22. Otaru N, Ye K, Mujezinovic D, et al. GABA production by human intestinal Bacteroides spp.: prevalence, regulation, and role in acid stress tolerance. Front Microbiol 2021;12:656895.
24. Didion JP, Martin M, Collins FS. Atropos: specific, sensitive, and speedy trimming of sequencing reads. PeerJ 2017;5:e3720.
25. Callahan BJ, McMurdie PJ, Rosen MJ, Han AW, Johnson AJ, Holmes SP. DADA2: High-resolution sample inference from Illumina amplicon data. Nat Methods 2016;13:581-3.
26. Microbiome Project Consortium. Structure, function and diversity of the healthy human microbiome. Nature 2012;486:207-14.
27. Le Chatelier E, Nielsen T, Qin J, et al; MetaHIT consortium. Richness of human gut microbiome correlates with metabolic markers. Nature 2013;500:541-6.
28. Arumugam M, Raes J, Pelletier E, et al; MetaHIT Consortium. Enterotypes of the human gut microbiome. Nature 2011;473:174-80.
29. The R Project for statistical computing. Available from: https://www.r-project.org/. [Last accessed on 27 May 2024].
30. McLaren M. speedyseq: faster implementations of phyloseq functions. Available from: https://github.com/mikemc/speedyseq. [Last accessed on 27 May 2024].
31. Oksanen J, Simpson GL, Blanchet FG et al. vegan: Community Ecology Package. Available from: https://cran.r-project.org/web/packages/vegan/index.html. [Last accessed on 27 May 2024].
32. Paradis E, Schliep K. ape 5.0: an environment for modern phylogenetics and evolutionary analyses in R. Bioinformatics 2019;35:526-8.
33. Andersen KS, Kirkegaard RH, Karst SM, Albertsen M. ampvis2: an R package to analyse and visualise 16S rRNA amplicon data. bioRXiv. [Preprint.] Apr 11, 2018 [accessed 2024 May 27]. Available from: https://www.biorxiv.org/content/10.1101/299537v1.
34. Constancias F, Sundar S. fconstancias/DivComAnalyses: v0.9. Zenodo. 2022. Available from: https://zenodo.org/records/6473394. [Last accessed on 27 May 2024].
35. Mallick H, Rahnavard A, McIver LJ, et al. Multivariable association discovery in population-scale meta-omics studies. PLoS Comput Biol 2021;17:e1009442.
36. Douglas GM, Maffei VJ, Zaneveld JR, et al. PICRUSt2 for prediction of metagenome functions. Nat Biotechnol 2020;38:685-8.
37. Caspi R, Billington R, Ferrer L, et al. The MetaCyc database of metabolic pathways and enzymes and the BioCyc collection of pathway/genome databases. Nucleic Acids Res 2016;44:D471-80.
38. Vandeputte D, Falony G, Vieira-Silva S, Tito RY, Joossens M, Raes J. Stool consistency is strongly associated with gut microbiota richness and composition, enterotypes and bacterial growth rates. Gut 2016;65:57-62.
39. Firrman J, Liu L, Mahalak K, et al. The impact of environmental pH on the gut microbiota community structure and short chain fatty acid production. FEMS Microbiol Ecol 2022;98:fiac038.
40. Wickham H, Navarro D, Pedersen TL. ggplot2: elegant graphics for data analysis (3e). New York: Springer. 2016. Available from: https://ggplot2-book.org/. [Last accessed on 27 May 2024].
41. Gu Z, Eils R, Schlesner M. Complex heatmaps reveal patterns and correlations in multidimensional genomic data. Bioinformatics 2016;32:2847-9.
42. Melchior M, Kuhn P, Poisbeau P. The burden of early life stress on the nociceptive system development and pain responses. Eur J Neurosci 2022;55:2216-41.
43. Zhong H, Penders J, Shi Z, et al. Impact of early events and lifestyle on the gut microbiota and metabolic phenotypes in young school-age children. Microbiome 2019;7:2.
44. Costea PI, Hildebrand F, Arumugam M, et al. Enterotypes in the landscape of gut microbial community composition. Nat Microbiol 2018;3:8-16.
45. Smith EA, Macfarlane GT. Enumeration of amino acid fermenting bacteria in the human large intestine: effects of pH and starch on peptide metabolism and dissimilation of amino acids. FEMS Microbiol Ecol 1998;25:355-68.
46. David LA, Maurice CF, Carmody RN, et al. Diet rapidly and reproducibly alters the human gut microbiome. Nature 2014;505:559-63.
47. Larsen OFA, Claassen E. The mechanistic link between health and gut microbiota diversity. Sci Rep 2018;8:2183.
48. Vijay A, Valdes AM. Role of the gut microbiome in chronic diseases: a narrative review. Eur J Clin Nutr 2022;76:489-501.
49. Kim MH, Yun KE, Kim J, et al. Gut microbiota and metabolic health among overweight and obese individuals. Sci Rep 2020;10:19417.
50. Rigsbee L, Agans R, Shankar V, et al. Quantitative profiling of gut microbiota of children with diarrhea-predominant irritable bowel syndrome. Am J Gastroenterol 2012;107:1740-51.
51. Barandouzi ZA, Lee J, Maas K, Starkweather AR, Cong XS. Altered gut microbiota in irritable bowel syndrome and its association with food components. J Pers Med 2021;11:35.
52. Manor O, Dai CL, Kornilov SA, et al. Health and disease markers correlate with gut microbiome composition across thousands of people. Nat Commun 2020;11:5206.
53. Mars RAT, Yang Y, Ward T, et al. Longitudinal multi-omics reveals subset-specific mechanisms underlying irritable bowel syndrome. Cell 2020;182:1460-73.e17.
55. Tartaglia D, Coccolini F, Mazzoni A, et al. Sarcina ventriculi infection: a rare but fearsome event. a systematic review of the literature. Int J Infect Dis 2022;115:48-61.
56. Guandalini S, Magazzù G, Chiaro A, et al. VSL#3 improves symptoms in children with irritable bowel syndrome: a multicenter, randomized, placebo-controlled, double-blind, crossover study. J Pediatr Gastroenterol Nutr 2010;51:24-30.
57. Giannetti E, Maglione M, Alessandrella A, et al. A mixture of 3 bifidobacteria decreases abdominal pain and improves the quality of life in children with irritable bowel syndrome: a multicenter, randomized, double-blind, placebo-controlled, crossover trial. J Clin Gastroenterol 2017;51:e5-10.
58. Guglielmetti S, Mora D, Gschwender M, Popp K. Randomised clinical trial: Bifidobacterium bifidum MIMBb75 significantly alleviates irritable bowel syndrome and improves quality of life--a double-blind, placebo-controlled study. Aliment Pharmacol Ther 2011;33:1123-32.
59. Ventura M, Canchaya C, Casale AD, et al. Analysis of bifidobacterial evolution using a multilocus approach. Int J Syst Evol Microbiol 2006;56:2783-92.
60. Alessandri G, van Sinderen D, Ventura M. The genus bifidobacterium: from genomics to functionality of an important component of the mammalian gut microbiota running title: Bifidobacterial adaptation to and interaction with the host. Comput Struct Biotechnol J 2021;19:1472-87.
61. Johnson JS, Spakowicz DJ, Hong BY, et al. Evaluation of 16S rRNA gene sequencing for species and strain-level microbiome analysis. Nat Commun 2019;10:5029.
62. Bosch S, van Gaal N, Zuurbier RP, et al. Differentiation between pediatric irritable bowel syndrome and inflammatory bowel disease based on fecal scent: proof of principle study. Inflamm Bowel Dis 2018;24:2468-75.
63. Shankar V, Homer D, Rigsbee L, et al. The networks of human gut microbe-metabolite associations are different between health and irritable bowel syndrome. ISME J 2015;9:1899-903.
64. Roswall J, Olsson LM, Kovatcheva-Datchary P, et al. Developmental trajectory of the healthy human gut microbiota during the first 5 years of life. Cell Host Microbe 2021;29:765-76.e3.
65. Tang Q, Jin G, Wang G, et al. Current sampling methods for gut microbiota: a call for more precise devices. Front Cell Infect Microbiol 2020;10:151.
67. Falony G, Joossens M, Vieira-Silva S, et al. Population-level analysis of gut microbiome variation. Science 2016;352:560-4.
68. Erben V, Poschet G, Schrotz-King P, Brenner H. Evaluation of different stool extraction methods for metabolomics measurements in human faecal samples. BMJ Nutr Prev Health 2021;4:374-84.
69. Cui M, Trimigno A, Castro-Mejía JL, et al. Human fecal metabolome reflects differences in body mass index, physical fitness, and blood lipoproteins in healthy older adults. Metabolites 2021;11:717.
Cite This Article
Export citation file: BibTeX | EndNote | RIS
OAE Style
Otaru N, Pugin B, Plüss S, Hojsak I, Braegger C, Lacroix C. A pilot case-control study on the fecal microbiota of pediatric functional abdominal pain-not otherwise specified and the role of early life stress. Microbiome Res Rep 2024;3:32. http://dx.doi.org/10.20517/mrr.2023.75
AMA Style
Otaru N, Pugin B, Plüss S, Hojsak I, Braegger C, Lacroix C. A pilot case-control study on the fecal microbiota of pediatric functional abdominal pain-not otherwise specified and the role of early life stress. Microbiome Research Reports. 2024; 3(3): 32. http://dx.doi.org/10.20517/mrr.2023.75
Chicago/Turabian Style
Nize Otaru, Benoît Pugin, Serafina Plüss, Iva Hojsak, Christian Braegger, Christophe Lacroix. 2024. "A pilot case-control study on the fecal microbiota of pediatric functional abdominal pain-not otherwise specified and the role of early life stress" Microbiome Research Reports. 3, no.3: 32. http://dx.doi.org/10.20517/mrr.2023.75
ACS Style
Otaru, N.; Pugin B.; Plüss S.; Hojsak I.; Braegger C.; Lacroix C. A pilot case-control study on the fecal microbiota of pediatric functional abdominal pain-not otherwise specified and the role of early life stress. Microbiome. Res. Rep. 2024, 3, 32. http://dx.doi.org/10.20517/mrr.2023.75
About This Article
Copyright
Data & Comments
Data
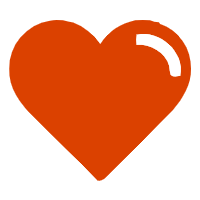
Comments
Comments must be written in English. Spam, offensive content, impersonation, and private information will not be permitted. If any comment is reported and identified as inappropriate content by OAE staff, the comment will be removed without notice. If you have any queries or need any help, please contact us at support@oaepublish.com.