Reversible phase transitions and enhanced electrostrain in BNST-xFN ceramics under electric and thermal stimuli
Abstract
Ferroelectric materials based on (Bi0.5Na0.5)TiO3 are well-known for their outstanding chemical stability and exceptional electrical properties, particularly their large electrostrain response under applied electric fields, positioning them as promising candidates for precision actuator applications. In this study, we investigate the electrical and structural responses of lead-free (Bi0.38Na0.38Sr0.24)Ti1-x(Fe0.5Nb0.5)xO3 (BNST-xFN) ferroelectric ceramics under the combined effects of temperature and electric field. Using in-situ electric field and variable-temperature Raman spectroscopy, piezoelectric force microscopy, and comprehensive dielectric and ferroelectric property evaluations, we explore the evolution of structural transformations, polarization behavior, and macroscopic property changes in ceramics with different initial phase structures under thermal and electrical stimuli. Notably, the BNST-0.01FN composition, located near the boundary between the non-ergodic relaxor and ergodic relaxor phases, exhibits a remarkable room-temperature electrostrain of 0.37%, driven by a reversible electric field-induced nonpolar-to-polar phase transition. Upon heating, as the BNST ceramic approaches the phase boundary, a prominent electrostrain (~0.38%) is observed near the temperature of the ferroelectric-to-relaxor phase transition (TFR, ~60 °C) under the electric field. This study combines in-situ microstructural analysis with macroscopic ferroelectric characterization, providing a deeper understanding of the dynamic coupling between microscopic fields and macroscopic electrical properties, and offering valuable insights for the design of high-performance lead-free ferroelectric ceramics.
Keywords
INTRODUCTION
Ferroelectric materials have long been at the forefront of materials science due to their unique electromechanical coupling properties, which enable a wide range of applications in sensors, actuators, and storage devices[1-5]. Among these, multilayer ceramic actuators (MLCAs) based on ferroelectric ceramics exhibit electric-field-induced strain (electrostrain) with numerous advantages, including high precision, rapid response, and low power consumption, making them ideal for ultra-precise positioning, as well as micro- and nano-scale actuation and control[6-8]. These attributes underscore their significant potential in advanced technological applications. Currently, commercially available actuators, such as fuel injection valves and micro-positioning systems, are typically based on lead-based ferroelectric materials such as lead zirconate titanate [Pb(Zr,Ti)O3 (PZT)] and its solid solutions[9-11]. However, the toxic lead oxides used in these materials pose serious health risks, driving the urgent need for effective lead-free alternatives[12-14].
The discovery of large electrostrain properties in (Bi0.5Na0.5)TiO3-BaTiO3-(K0.5Na0.5)NbO3 (BNT-BT-KNN) materials by Zhang et al. in 2007[15] marked a significant breakthrough, attracting considerable attention to BNT and its solid solutions, which exhibit recoverable electrostrain that comparable, and even surpass, those of lead-based materials[7,16,17]. This breakthrough opened new avenues for the development of lead-free ferroelectric actuators, with the potential to replace lead-based materials in commercial applications, spurring extensive research in this area[18,19]. For instance, BNT-BT-K0.47Na0.47Li0.06Nb0.99Sb0.01O2.99 (BNBT-KNLNS) ceramics, designed by Li et al., exhibited large electrostrain values (0.32%-0.51%) and low strain hysteresis (~11.1%) across a wide temperature range (25-125 °C)[20]. This exceptional performance is attributed to the incorporation of a small fraction of ferroelectric states within the morphotropic phase boundary (MPB) matrix, which consists of tetragonal (T) and rhombohedral (R) phases, enabling a reversible transition from the relaxor to the ferroelectric phase[21].
These findings underscore the significant impact of phase transitions on the electrostrain properties of BNT-based materials, highlighting the close relationship between electrostrain behavior and phase boundary characteristics. In these materials, the relaxor phases can be classified as the non-ergodic relaxor (NER) and ER phases, with the key distinction being whether the ferroelectric phase can be stabilized after the electric field is removed[22]. The phase boundaries in BNT-based materials are often optimized through the formation of solid solutions or the introduction of dopant components, which further enhance their electrostrain performance[17].
BNT-based actuator materials, driven by phase-transition electrostrain properties, are often plagued by significant hysteresis and high driving electric fields, which remain major obstacles to their practical application[7]. However, doping with ferroelectric materials such as BaTiO3 (BT) and paraelectric materials such as SrTiO3 (ST) with lower coercive fields has proven effective in reducing both the driving electric field and strain hysteresis, facilitating high electrostrain responses[23,24]. For instance, Bai et al. demonstrated that the incorporation of ST into BNT-0.17(Bi0.5K0.5)TiO3 (BNT-0.17BKT) ceramics enhanced electrostrain while simultaneously decreasing ferroelectricity. At a relatively low driving electric field of 60 kV/cm, these ceramics exhibited a substantial electrostrain response (S = 0.38%,
In addition to the incorporation of new components and BNT-based solid solution strategies, ceramics can also be modified through ion doping, where ions of varying valences, radii, and concentrations are introduced into the ceramic matrix to induce lattice distortion, oxygen vacancies, and localized random fields, thereby enhancing the electromechanical properties[1-7]. Ion doping can be categorized based on the doping site: A-site[28], B-site[29,30], and A/B-site co-doping[31-34]. Multiple-ion doping can optimize the electric domain structure and phase transition behavior, reducing hysteresis and driving electric fields while improving electrostrain responses. For example, Rahman et al. achieved an electrostrain of 0.38% at
The electrostrain response of BNT-based ceramics is intrinsically linked to their complex phase transitions, which are highly sensitive to both thermal and electrical stimuli. However, the interplay between temperature and electric field, along with its influence on the electrostrain response, remains not fully understood. Identifying the relationship between microstructure, polarization behavior, and macroscopic electrostrain is crucial for elucidating the underlying mechanisms. In-situ Raman spectroscopy, a powerful tool for probing local structural changes in ferroelectric materials under varying temperature and electric field conditions, offers real-time insights into the material's response to external stimuli. This provides valuable information on the atomic-scale mechanisms that govern the macroscopic properties of these materials[40]. Additionally, piezoelectric force microscopy (PFM) is an essential technique for direct observation of domain structures and polarization responses in ferroelectric ceramics[41]. Combining these techniques with measurements of the temperature-dependent properties of ceramics, such as dielectric behavior and polarization-electric field (P-E) hysteresis loops- represents a reliable method for characterizing the electromechanical properties of BNT-based materials[42]. This integrated approach enables a comprehensive understanding of the electrostrain response in BNT-based ceramics, which is critical for their application in high-precision positioning systems and sensors.
This study investigates the electrostrain response of BNT-based ceramics under dual stimulation by temperature and electric field, with a focus on structural distortions, local polarization behavior, and their effects on electrostrain. The work was conducted on (Fe0.5Nb0.5)4+-doped BNT-0.24ST systems, featuring different initial phase structures with different doping levels. An electrostrain of 0.37% was achieved in ceramics with 0.01 mol.% (Fe0.5Nb0.5)4+ doping. Notably, around 60 °C, BNT-0.24ST ceramics undergo a NER to ER phase transition, leading to a significant electrostrain (~0.38%) via a reversible nonpolar-to-polar phase transition induced by the electric field. The intrinsic mechanism behind this electrostrain enhancement is explored through a comprehensive analysis of in-situ electric field Raman spectroscopy, PFM, and ferroelectric properties (P-E loops, S-E curves and J-E curves) across varying electric fields and temperatures. Our findings not only illuminate the complex phase transitions in BNT-based ceramics but also offer valuable insights for the design of high-performance, lead-free ferroelectric ceramics for actuator applications.
MATERIALS AND METHODS
Ceramic samples of the (Bi0.38Na0.38Sr0.24)Ti1-x(Fe0.5Nb0.5)xO3 (BNST-xFN, x = 0, 0.01, 0.02, 0.03) system were synthesized using a traditional solid-state reaction method. The raw materials, including Bi2O3 (99%),
For X-ray diffraction (XRD) measurements, ceramic samples were milled to fine powder using an onyx mortar and annealed at 500 °C for several hours to remove internal stresses. XRD was performed using a SmartLab X-ray diffractometer (Rigaku, Japan) equipped with a temperature control system. Scans were conducted over a range of 10° to 90° at room temperature (RT), with a step size of 0.01° and a scan rate of 1°/min. Data refinement was performed using the FULLPROF software (version 2000) to accurately determine the lattice parameters. High-temperature XRD tests were conducted on both poled and unpoled bulk ceramics. The samples were polished, annealed, and then covered with room-temperature silver paste, followed by polarization for over 20 min at an electric field higher than the coercive field. The silver paste was subsequently removed with alcohol. Test data is collected at 10 °C intervals, with the temperature rising at a rate of 10 °C/min, with testing commencing after stabilization at the target temperature for 1 min, using a scanning step of 0.01° and a rate of 1°/min. Raman spectra were collected using a Raman spectrometer (LabRam HR Evolution, HORIBA JOBIN YVON, France) equipped with a heating stage and excited by a 532 nm laser. In-situ Raman measurements were performed under an applied electric field, with silver paste electrodes on both the upper and lower surfaces of the side-polished ceramic samples. The temperature was ramped at 10 °C/min, with measurements taken after stabilization at the target temperature for 1 min. Micro-morphological images were obtained by thermally etching the polished samples for 20 min at approximately 100 °C below the sintering temperature, followed by scanning electron microscopy (SEM, Quanta FEG 250, FEI, USA). Dielectric properties were measured on silver-electrode-coated samples using a multi-frequency LCR meter (E4980A, Agilent, USA). Additionally, the temperature rises from RT to
RESULTS AND DISCUSSION
In Figure 1A, the XRD patterns of BNST-xFN ceramics reveal that both BNST and BNST-0.01FN exhibit a pure perovskite structure within the resolution range of the instrument. And it can be seen from Figure 1B that the (110) diffraction peak shifts to higher angles with increasing doping content, a result of the substitution of Ti4+ by the (Fe0.5Nb0.5)4+ composite ion at the B-site. In ABO3-type perovskites, the B-site coordination number (CN) is 6, with an ion radius of 0.605 Å[43] for Ti4+. For Fe3+ and Nb5+, which have the same CN, the ion radii are 0.55 and 0.645 Å, respectively. The calculated ion radius of (Fe0.5Nb0.5)4+ is
Figure 1. Microstructure and surface morphology of BNST-xFN ceramic samples after thermal etching. (A) X-ray diffraction (XRD) patterns of BNST-xFN ceramics, with red pentagrams indicating the peaks corresponding to the impurity phase. (B) Enlarged view of the (111) diffraction peak. (C) Magnified image of the (200) diffraction peak. (D) Raman spectra of BNST-xFN ceramic samples, along with the results of fitting thirteen Gaussian-Lorentzian peaks. (E) Variation of the Gaussian-Lorentzian peak positions across the thirteen peaks. (F1-F4) Surface morphology maps of BNST-xFN ceramics after thermal etching: (F1) x = 0, (F2) x = 0.01,
Raman spectroscopy, a powerful vibrational technique, provides valuable insights into the molecular vibrational modes and structural evolution of materials[40,44-46]. We measured the Raman spectra of BNST-xFN ceramics in the wavenumber range of 20-1,000 cm-1, with the results shown in Figure 1D. The Raman spectra can be divided into four distinct regions[40,44], each corresponding to different lattice vibrational modes. Region I (wavenumbers below ~200 cm-1) is primarily associated with vibrations of the A-site cations, such as Na+, Bi3+, and Sr2+, in this study. Region II, spanning roughly 200-425 cm-1, is linked to the vibrational modes of the Ti-O bond. The region from 425 to 680 cm-1 corresponds to vibrations of the TiO6 octahedron, including stretching and bending modes of the oxygen octahedra. In Region IV (above
However, due to the relative weakness of some modes[48], we used 13 Gaussian-Lorentzian peak functions to deconvolve the Raman spectra of BNST, BNST-0.01FN, BNST-0.02FN, and BNST-0.03FN, employing the best fitting algorithm, as shown in Figure 1D. The fitted peak positions are summarized in Figure 1E. Comparing the four Raman spectra in Figure 1D, it is evident that BNST and BNST-0.01FN exhibit similar peak profiles. However, in regions II and III, the peaks of BNST-0.01FN are noticeably broader than those of BNST. This broadening is attributed to the doping of (Fe0.5Nb0.5)4+ ions, which increases ion disorder and facilitates the transition to the ER phase[40]. In contrast, BNST-0.02FN and BNST-0.03FN show distinctly different spectra, likely due to the formation of the impurity phase and Enhanced relaxation. The presence of impurity phases notably affects both the microstructure and morphology of the ceramics. As shown in Figure 1F1-F4, the surface morphology of the ceramics is visibly altered after thermal etching. Doping with
The structure and grain evolution of ceramics directly influence their electrical properties. Figure 2 presents the dielectric and ferroelectric characteristics of the BNST-xFN ceramic samples. Specifically, Figure 2A-D shows the temperature-dependent variations of the dielectric constant (εr) and dielectric loss (tanδ) of the poled BNST-xFN ceramics, measured from RT to 450 °C at different frequencies. Prior to reaching the maximum dielectric constant (εm), εr exhibits frequency dispersion as a function of frequency. This behavior is attributed to the relaxation characteristics of the polarization response within the dielectric material. At a constant temperature, an increase in frequency typically results in a slight decrease in εr. This trend is due to the fact that at lower frequencies, the polarization mechanisms within the material have sufficient time to fully flourish, thereby enhancing the dielectric response. This phenomenon is not unique to BNT-based materials; it is a common feature observed in many relaxor ferroelectrics[49,50]. For the BNST and BNST-0.01FN samples, the εr begins to decline above the characteristic temperature (Tm), which corresponds to the εm. This decrease is attributed to the weakening of the polarization response associated with the paraelectric phase. Concurrently, the frequency dispersion is significantly reduced, indicating that the relaxation processes are suppressed at elevated temperatures[51].
Figure 2. Dielectric and ferroelectric properties of BNST-xFN ceramic samples. (A-D) Dielectric constant (εr) and dielectric loss (tanδ) of BNST-xFN ceramics measured at 1, 10, and 100 kHz, 1 MHz as a function of temperature from room temperature (RT) to
In contrast, BNST-0.02FN and BNST-0.03FN display significant frequency dispersion throughout the entire temperature range[21]. This behavior is likely associated with the complex nature of the polarization mechanisms in these materials. Specifically, the presence of polar nanoregions (PNRs) and the formation of impurity phase, as illustrated in Figure 1F3 and F4, significantly influence the dielectric response. These structural features introduce local inhomogeneities that enhance the relaxor behavior, leading to the observed frequency dispersion[52]. Notably, a sharp increase in both εr and tanδ, especially at low frequencies
The introduction of the paraelectric phase, ST, leads to a marked decrease in the Curie temperature (TC) of the ceramics, compared to the 320 °C typically observed for BNT[53]. However, doping with (Fe0.5Nb0.5)4+ has a minimal effect on Tm, with only slight fluctuations in the temperatures across all four samples. This indicates that the (Fe0.5Nb0.5)4+ dopants primarily influence the relaxation behavior rather than the ferroelectric phase transition temperature. In addition to the primary dielectric anomaly peak corresponding to the εm, a second anomaly peak appears in BNST, more prominently visible in the tanδ curve (inset of Figure 2A). This anomaly is characteristic of the poled NER-phase BNT-based ceramics and marks the transition from the ferroelectric phase to the relaxor phase, which is defined as the TFR. Upon doping with (Fe0.5Nb0.5)4+, the TFR for the other three samples shifts below RT, indicating that the ceramic samples transition from the NER phase to the ER phase.
To visualize the impact of (Fe0.5Nb0.5)4+ doping on the dielectric properties of the ceramics, we have analyzed the temperature-dependent variation of the εr at a frequency of 1 MHz, as shown in Figure 2E. The characteristic temperatures and εm extracted from these dielectric properties are summarized in Figure 2F. The results indicate that the εr curves flatten as the doping level of (Fe0.5Nb0.5)4+ increases, suggesting that (Fe0.5Nb0.5)4+ doping significantly enhances the dielectric relaxation in the ceramics. Specifically, the εm value remains relatively stable for BNST-0.01FN but decreases significantly with further doping (in BNST-0.02FN and BNST-0.03FN). This behavior is attributed to two primary factors. First, higher concentrations of
The ferroelectric properties also exhibit significant changes with increasing doping. As shown in Figure 2G, BNST displays a typical ferroelectric hysteresis loop at 100 kV/cm, while the P-E loop of
The significant differences in dielectric and ferroelectric properties suggest that ceramic samples with distinct phase structures exhibit fundamentally different responses to electric field stimuli. As shown in Figure 3A, the J-E curves of BNST under an applied electric field exhibit four centrosymmetric peaks, with all positive current density peaks located in the first quadrant. Peak P1 corresponds to domain switching in a typical ferroelectric, while peak P2, appearing at higher electric fields, is associated with the presence of PNRs, which exhibit relatively weak current peaks[54].
Figure 3. Polarization response and microstructure under electric field of BNST ceramic. (A) J-E curves of BNST ceramic samples at different applied electric fields. (B) Amplitude image and (C) phase image of domain structures in BNST ceramic. (D) Local switching spectra obtained via PFM, showing phase and amplitude response as a function of electric field. (E) Raman spectra and the fitting results of thirteen Gaussian-Lorentzian peaks for BNST ceramic at varying electric fields. (F) Variation of Gaussian-Lorentzian peak positions in regions I-III as a function of electric field. (G) Comparison of Raman spectra for poled and unpoled BNST samples.
The simultaneous presence of these two peaks indicates that the response to the electric field in BNST is primarily driven by domain switching, superimposed by the effects of PNRs. To further investigate the polarization response of the BNST sample, we performed PFM tests, and the results are shown in
In-situ analysis techniques offer an intuitive means to explore the evolution of materials under the influence of electric fields[40]. As shown in Figure 3E and F, we present the results of in-situ Raman spectra under applied electric fields. Figure 3E reveals significant changes in the vibrational modes of the A-site, B-O bond, and BO6 octahedron as the electric field is progressively enhanced. In Region I, the peak corresponding to W1 evolves from being flush with the left peak to significantly surpassing it, while peak W2 gradually emerges as the electric field reaches 40 kV/cm. These observations indicate a distinct phase transition in the ceramic samples under the electric field, leading to changes at the atomic and bond scales. In Region II, the peak corresponding to the B-O bond sharpens and becomes more concentrated, suggesting that the applied electric field induces a transition from disorder to order within the lattice[40,44], a phenomenon also observed in other BNT-based materials, implying enhanced polarity of the unit cell[40,44]. The most prominent change occurs in the vibrational modes of the BO6 octahedron, where the W4 peak persists up to 35 kV/cm, while the W5 peak, present in the absence of the electric field, gradually diminishes as the field increases. This shift causes the BO6 octahedron vibrational mode to transition from broadening to concentration, mirroring the trend in Region II, and indicates that the nanodomains generated by bond weakening are aligned under the applied electric field, promoting polarity through a transition from disorder to order[40,44]. Notably, the electric field intensities corresponding to the P1 and P2 peaks in the J-E curve depicted in Figure 3A are 7.3 and 25.4 kV/cm, respectively. These peaks are indicative of distinct modes in the polarization response of the ceramics under an applied electric field. The P1 peak, occurring at a lower electric field, is associated with domain switching, while the P2 peak at higher electric fields is dominated by the arrangement of PNRs. As the applied electric field increases in Figure 3E, the W5 peak existing in the initial state disappears between 20 and 25 kV/cm. This disappearance coincides precisely with the electric field range between the P1 and P2 peaks in the J-E curve [Figure 3A]. This observation suggests that domain switching is complete and an ordered evolution of the polarization response has occurred.
As the electric field reaches 40 kV/cm, peak W4 is replaced by W6, indicating a significant change in the polarization response. At this stage, the domain flip has already been completed, and the response from the PNRs becomes more diffuse and less pronounced above the P1 peak. This indicates that these regions are contributing to the overall polarization but are not as sharply defined as the primary domain-switching peaks. This behavior is consistent with the relaxor nature of BNST ceramics, where the presence of PNRs leads to a broad and diffuse polarization response rather than sharp transitions[21].
The peak position change curves for different peaks in each region, as shown in Figure 3F, further confirm that the inflection points of the peak shifts in the in-situ Raman spectra often correspond to the polarization response modes of BNST under different electric field strengths, as observed in Figure 3A. This correspondence underscores the close relationship between microstructural changes and the polarization response in BNST ceramic. The initial sharp peaks (W4 and W5) are associated with domain switching, while the later peaks (W6) and the diffuse response from the PNRs indicate a more complex interplay between domain alignment and PNR reorientation. This finding highlights the importance of understanding the microstructural changes that occur under an applied electric field, as these changes significantly influence the macroscopic electrical properties of the material[55]. Finally, a comparison of the Raman spectra of poled and unpoled ceramics in Figure 3G provides a more intuitive understanding of the narrowing of B-O bonding and BO6 octahedron vibrational peaks, along with changes in peak shapes and intensities in Region I[51]. These results clearly demonstrate that the electric field induces a phase structure change in the ceramic samples, which in turn influences the arrangement of A-site ions in the lattice.
For BNST-0.01FN, Figure 4A shows that the peak P1 has significantly reduced in intensity and shifted to the second quadrant, compared to BNST. This suggests that the ceramic material tends to revert to a nonpolar state upon removal of the electric field. However, the persistence of a significant intensity for peak P1, along with its broadening, continues until the electric field is reduced to zero, indicating that some domain switching remains within the ceramic. This observation is further supported by the PFM results presented in Figure 4B-D. The amplitude and phase images in Figure 4B and C reveal that BNST-0.01FN consists of nanodomains with noticeably reduced sizes, as well as regions that exhibit no domain response, likely corresponding to the PNRs. Additionally, the amplitude image in Figure 4D aligns with the S-E curve of BNST-0.01FN, showing no negative electrostrain, which further corroborates the absence of a negative electrostrain response in the material.
Figure 4. Polarization response and microstructure under electric field of BNST-0.01FN ceramic. (A) J-E curves of BNST-0.01FN ceramic samples at different applied electric fields. (B) Amplitude image and (C) phase image of domain structures in BNST-0.01FN ceramic. (D) Local switching spectra obtained via PFM, showing phase and amplitude response as a function of electric field. (E) Raman spectra and the fitting results of thirteen Gaussian-Lorentzian peaks for BNST-0.01FN ceramic at various electric fields. (F) Variation of Gaussian-Lorentzian peak positions in regions I-III as a function of electric field. (G) Comparison of Raman spectra for poled and unpoled BNST-0.01FN samples.
In comparison with the Raman spectra of BNST under an in-situ electric field, the results for BNST-0.01FN, presented in Figure 4E-G, show notable differences. The Raman spectra under varying applied electric fields, as shown in Figure 4E, reveal that the A-site vibrational modes remain largely unaffected, while the peaks corresponding to the B-O bond and BO6 octahedra undergo significant evolution. This suggests that the applied electric field induces a transition from disorder to order within the lattice, enhancing the polarity of the unit cell[40,44]. As indicated by the peak shifts in Figure 4F, the primary fluctuation occurs in region II, which corresponds to the B-O bond vibrational mode, with the A-site ions near the B-O bond (around 200 cm-1) being the most affected by this fluctuation. This change is most pronounced in the comparative curves shown in Figure 4G. The doping of (Fe0.5Nb0.5)4+ leads to a more pronounced response from the PNRs within the ceramic, which align under the applied electric field. However, this response has little impact on the lattice structure itself, resulting in the narrowing of the vibrational mode peaks for the B-O bond and BO6 octahedra, while the A-site vibrational modes remain almost unchanged.
As doping increases to BNST-0.03FN, no distinct current density peaks are observed in the J-E curves, as shown in Figure 5A, and the domain structure becomes increasingly undefined, as seen in Figure 5B and C. In BNST-0.03FN, only the tiny PNR response contributes to polarization, resulting in weak signal feedback in the phase and amplitude images at high applied bias, as shown in Figure 5D. This behavior corresponds to a significant reduction in both Pmax and Smax in the P-E loop and S-E curve. The in-situ Raman spectra, presented in Figure 5E and F, also show minimal changes, with only slight fluctuations in peak positions at high electric fields. Notably, the Raman spectra of the poled and unpoled samples almost entirely overlap, as shown in Figure 5G, indicating that the vibrational modes and lattice structures remain stable. These results suggest that as (Fe0.5Nb0.5)4+ doping increases, the ferroelectric characteristics of the ceramic gradually diminish, and its response to the applied electric field becomes increasingly less sensitive.
Figure 5. Polarization response and microstructure under electric field of BNST-0.03FN ceramic. (A) J-E curves of BNST-0.03FN ceramic samples at different applied electric fields. (B) Amplitude image and (C) phase image of domain structures in BNST-0.03FN ceramic. (D) Local switching spectra obtained via PFM, showing phase and amplitude response as a function of electric field. (E) Raman spectra and the fitting results of thirteen Gaussian-Lorentzian peaks for BNST-0.03FN ceramic at various electric fields. (F) Variation of Gaussian-Lorentzian peak positions in regions I-III as a function of electric field. (G) Comparison of Raman spectra for poled and unpoled BNST-0.03FN samples.
The analysis of Figures 2-5 reveals that ion doping can effectively modify the characteristic temperature of BNT-based ceramics and influence their phase structure, leading to optimized and enhanced material properties. Further investigation into the evolution of phase structures in BNT-based materials with varying doping levels, along with the corresponding microscopic polarization response and macroscopic property changes, is an important avenue for future research. To this end, we conducted detailed studies on the structural, polarization, and ferroelectric property variations of three different ceramic samples during the heating process. The results of these studies are presented in Figures 6-8.
Figure 6. Ferroelectric properties, polarization response, and microstructure during the heating process of BNST ceramic. (A) P-E loops and (B) S-E curves of BNST ceramic at different temperatures. (C) Variation of Pmax, Pr, Smax and Sneg as a function of temperature. (D)
Figure 7. Ferroelectric properties, polarization response, and microstructure during the heating process of BNST-0.01FN ceramic. (A)
Figure 8. Ferroelectric properties, polarization response, and microstructure during the heating process of BNST-0.03FN ceramic. (A) P-E loops and (B) S-E curves of BNST-0.03FN ceramic at different temperatures. (C) Variation of Pmax, Pr, Smax and Sneg as a function of temperature. (D) J-E curves of BNST-0.03FN ceramic at different temperatures. (E) Two-dimensional evolution of the J-E curves with temperature in the first and second quadrants. (F) XRD pattern showing the evolution of the (111) peak and (200) peak with temperature of BNST-0.03FN ceramic. (G) Raman spectra and the fitting results of thirteen Gaussian-Lorentzian peaks for BNST-0.03FN ceramic at different temperatures. (H) Variation of Gaussian-Lorentzian peak positions in regions I-III as a function of temperature.
The evolution of the P-E loops and S-E curves with increasing temperature for BNST, along with the corresponding ferroelectric parameters, is shown in Figure 6A-C. As the temperature increases, the P-E loops of BNST gradually exhibit a pinched shape, accompanied by a significant decrease in Pmax at a certain temperature. Notably, the Sneg gradually disappears, while the Smax increases, reaching a peak of 0.38% near the TFR. Interestingly, as the temperature rises, the P-E loops and S-E curves of BNST evolve toward the features observed in BNST-0.01FN. To gain deeper insight into the polarization response, J-E curves were measured at various temperatures, and the two-dimensional evolution of the first and second quadrant J-E curves with temperature is depicted in Figure 6D and E. The results show that peak P1 shifts toward lower electric fields as the temperature increases, eventually moving to the second quadrant, such as BNST-0.01FN. In contrast, peak P2 moves in the opposite direction, and the intensities of both peaks gradually decrease, ultimately merging into a broad peak without distinct current maxima at high temperatures. This indicates a transition from the NER to the ER phase during heating, with the contribution of domain switching decreasing. Consequently, the P-E loop girdles, and Sneg decreases. As the ceramic reaches the boundary between the NER and ER phases, the potential barrier between these phases diminishes due to the proximity of the free energies of the electric field-induced ferroelectric and ER phases, leading to a significant enhancement of electrostrain performance[56]. Upon further heating, as BNST fully transitions to the ER phase, the size and number of PNRs decrease, weakening the polarization contribution. This results in a reduction of both Pmax and Smax due to the increased potential barrier in the ER phase and a decrease in the free energy of the ER phase.
XRD and Raman spectroscopy were employed to investigate the structural evolution of the ceramics with temperature, as shown in Figure 6F-H. Figure 6F presents the evolution of the (111) and (200) peaks of the poled sample during the heating process. Notably, the shoulder of the (111) peak, highlighted by the orange circle at RT, evolves into a distinct peak as the temperature increases, completing this transition near the
For BNST-0.01FN, which is already in the ER phase, the P-E loops shown in Figure 7A gradually evolve from a pinched to an inclined shape with increasing temperature. This behavior is consistent with the S-E curves in Figure 7B, where both Pmax and Smax decrease progressively. As shown in Figure 7C, the temperature dependence of Pmax, Smax, and Pr indicates a gradual reduction in all parameters without any abrupt transitions, suggesting that ceramics in the ER phase exhibit enhanced relaxation behavior under elevated temperatures. This observation is further confirmed in Figure 7D and E, where the two current density peaks significantly diminish in intensity and eventually disappear with increasing temperature. The J-E curve forms a broad bulge, signaling a decrease in the size and number of PNRs, and a weakening of the polarization contribution. As a result, the ability of the ceramic to induce a strong polar state under an applied electric field is diminished.
XRD results in Figure 7F show no changes in phase structure, with the (111) and (200) peaks merely shifting to lower angles due to temperature-induced lattice expansion. Similarly, the Raman spectra in
A similar phenomenon was observed in the BNST-0.03FN ceramic sample, with even more stable P-E loops and S-E curves, as well as corresponding Pmax, Smax and Pr parameters shown in Figure 8A-C. However, the
The above analyses reveal that the phase structure of ceramics can be modulated through doping and temperature control, which in turn affects their response behavior and ferroelectric properties under an applied electric field. To better illustrate these findings, we constructed the schematic shown in Figure 9. Figure 9A illustrates that BNST ceramics below the TFR are in the NER phase. As indicated by the clear relaxation behavior observed in the dielectric constant profile [Figure 2A] and the distinct distribution of domains revealed by PFM results [Figure 3C], the initial microstructure of BNST consists of isotropic domains interspersed with a small number of PNRs, as depicted in Figure 9B. When an electric field is applied, both domain switching and the alignment of the PNRs contribute to the polarization response. This dual mechanism results in four centrosymmetric current density peaks in the first and fourth quadrants of the J-E curve, as shown in Figure 9C. At low electric fields, the current density peaks primarily arise from domain switching, which is accompanied by a sharp increase in polarization in the P-E loop and the appearance of Sneg in the S-E curve. This behavior is indicative of the significant contribution of domain reorientation to the polarization response, as commonly observed in ferroelectric materials. Conversely, the current peaks at higher electric fields are associated with the alignment of the PNRs. At this point, the increase in polarization in the P-E loop begins to slow down, suggesting that the PNRs are gradually contributing to the overall polarization response. Importantly, the transition from the NER phase to the ferroelectric phase is irreversible. Consequently, the microstructure cannot be restored to its original state upon removal of the electric field, as illustrated in Figure 9B. This irreversibility leads to a significant Pr in the P-E loop.
Figure 9. Polarization response and macroscopic properties of ceramics with different phase structures. (A) Schematic showing the dielectric constant as a function of temperature for BNST-xFN ceramics, with BNST, BNST-0.01FN, and BNST-0.03FN represented at distinct positions along the curve. (B) Initial domain structure, polarization response under an applied electric field, and after field removal for BNST ceramic. (C) P-E loops, S-E curve and J-E curve of BNST ceramic. (D) Initial domain structure, polarization response under an applied electric field, and after field removal for BNST-0.01FN ceramic. (E) P-E loops, S-E curve and J-E curve of BNST-0.01FN ceramic. (F) Initial domain structure, polarization response under an applied electric field, and after field removal for BNST-0.03FN ceramic. (G) P-E loops, S-E curve and J-E curve of BNST-0.03FN ceramic.
When 1 mol.% of (Fe0.5Nb0.5)4+ is doped into the ceramics, the dielectric profile shifts above the TFR, as shown in Figure 9A. According to the PFM results in Figure 4B and C, BNST-0.01FN is situated at the phase boundary between the NER and ER phases. Its microstructure is dominated by PNRs, with a small number of significantly reduced-size nanodomains [Figure 9D]. Since the free energy at the phase boundary between the NER and ER phases is close to that of the ferroelectric phase, with a low potential barrier, the applied electric field can more easily induce a transition to the ferroelectric phase. This transition is reversible. Upon removal of the electric field, the PNR regions can recover, leading to the pinched P-E loop shown in Figure 9E (with a sharp decrease in Pr compared to BNST) and the near disappearance of Sneg in the S-E curve. Reversible nonpolar-to-polar phase transitions usually result in a significantly higher Smax.
With further doping, the dielectric curve shifts further away from the TFR, and the relaxation of BNST-0.03FN is enhanced. In this case, the polarization response is primarily contributed by the smaller-sized PNRs, as shown in Figure 9F. Since the free energy is now significantly deviated from that of the ferroelectric phase, the electric field can no longer induce long-range ordered polarization, leading to a significant reduction in polarization, electrostrain, and current density responses, as shown in Figure 9G.
By comprehensively analyzing the structure, polarization response, and property effects of different phase structures of BNT-based ceramics under in situ electric field stimulation, combined with the temperature variation effects, we gain a deeper understanding of the evolution under the influence of chemical modification, electric field, and temperature. This understanding can help in the design of materials with enhanced electrostrain properties for lead-free actuator applications.
CONCLUSIONS
This study systematically investigates the phase structure evolution, polarization behavior, and electrical properties of BNST-xFN lead-free ferroelectric ceramics under the combined influences of electric field and temperature. Through a detailed analysis of the electrostrain behavior across ceramics with varying initial phase structures, we identify the mechanisms responsible for their distinct responses. Notably, BNST-0.01FN ceramics, located at the phase boundary between the NER and ER phases, undergo a reversible nonpolar-to-polar phase transition when subjected to an electric field, resulting in an electrostrain of 0.37%. Furthermore, BNST ceramics initially in the NER phase exhibit a gradual transition to the ER phase as the temperature increases, yielding a significant electrostrain of 0.38% under the synergistic effects of electric field and thermal stimuli. These findings underscore the dynamic interplay between microstructural evolution and macroscopic electrical properties, providing critical insights for the design of advanced lead-free actuator materials with enhanced electrostrain performance.
DECLARATIONS
Authors’ contributions
Conceptualization, supervision, investigation, formal analysis, funding acquisition, writing - original draft: Jing, R.
Investigation, formal analysis: Man, W.
Data curation: Nie, X.
Formal analysis: Zhang, L.
Conceptualization, supervision, methodology, resources, project administration, funding acquisition, writing - review & editing: Jin, L.
Availability of data and materials
The rata data supporting the findings of this study are available within this Article and its
Financial support and sponsorship
This work was finically supported by the National Natural Science Foundation of China (Grant Nos. 52302153 and 52261135548), the China Postdoctoral Science Foundation (Grant Nos. GZC20232075 and 2023M742767) and Shaanxi Province postdoctoral research project (Project No. 2023BSHTBZZ14). The SEM work was done at the International Center for Dielectric Research (ICDR), Xi’an Jiaotong University, Xi’an, China.
Conflicts of interest
All authors declared that there are no conflicts of interest.
Ethical approval and consent to participate
Not applicable.
Consent for publication
Not applicable.
Copyright
© The Author(s) 2025.
Supplementary Materials
REFERENCES
1. Jo, W.; Dittmer, R.; Acosta, M.; et al. Giant electric-field-induced strains in lead-free ceramics for actuator applications - status and perspective. J. Electroceram. 2012, 29, 71-93.
2. Panda, P. K.; Sahoo, B. PZT to lead free piezo ceramics: a review. Ferroelectrics 2015, 474, 128-43.
3. Yang, L.; Kong, X.; Li, F.; et al. Perovskite lead-free dielectrics for energy storage applications. Prog. Mater. Sci. 2019, 102, 72-108.
4. Zhang, L.; Jing, R.; Huang, Y.; et al. Ultra-weak polarization-strain coupling effect boosts capacitive energy storage. Adv. Mater. 2024, 36, e2406219.
5. Zhang, S. High entropy design: a new pathway to promote the piezoelectricity and dielectric energy storage in perovskite oxides. Microstructures 2023, 3, 2023003.
6. Hao, J.; Li, W.; Zhai, J.; Chen, H. Progress in high-strain perovskite piezoelectric ceramics. Mater. Sci. Eng. R. Rep. 2019, 135, 1-57.
7. Fan, P.; Liu, K.; Ma, W.; et al. Progress and perspective of high strain NBT-based lead-free piezoceramics and multilayer actuators. J. Materiomics. 2021, 7, 508-44.
8. Wei, Y.; Deng, Y.; Dong, S.; et al. Enhancement of piezoelectric performance in (Bi1/2Na1/2)TiO3-based system through single-crystallization. Chem. Eng. J. 2024, 496, 153996.
9. Li, F.; Wang, L.; Jin, L.; et al. Piezoelectric activity in Perovskite ferroelectric crystals. IEEE. Trans. Ultrason. Ferroelectr. Freq. Control. 2015, 62, 18-32.
10. Zeng, J.; Zhao, K.; Shi, X.; Ruan, X.; Zheng, L.; Li, G. Large strain induced by the alignment of defect dipoles in (Bi3+,Fe3+) co-doped Pb(Zr,Ti)O3 ceramics. Scripta. Mater. 2018, 142, 20-2.
11. Bian, L.; Qi, X.; Li, K.; et al. High-performance [001]c-textured PNN-PZT relaxor ferroelectric ceramics for electromechanical coupling devices. Adv. Funct. Mater. 2020, 30, 2001846.
12. Panda, P. K. Review: environmental friendly lead-free piezoelectric materials. J. Mater. Sci. 2009, 44, 5049-62.
13. Zheng, T.; Wu, J.; Xiao, D.; Zhu, J. Recent development in lead-free perovskite piezoelectric bulk materials. Prog. Mater. Sci. 2018, 98, 552-624.
14. Wang, D.; Fan, Z.; Rao, G.; et al. Ultrahigh piezoelectricity in lead-free piezoceramics by synergistic design. Nano. Energy. 2020, 76, 104944.
15. Zhang, S.; Kounga, A. B.; Aulbach, E.; Ehrenberg, H.; Rödel, J. Giant strain in lead-free piezoceramics Bi0.5Na0.5TiO3-BaTiO3-
16. Viola, G.; Tian, Y.; Yu, C.; et al. Electric field-induced transformations in bismuth sodium titanate-based materials. Prog. Mater. Sci. 2021, 122, 100837.
17. Zhou, X.; Xue, G.; Luo, H.; Bowen, C. R.; Zhang, D. Phase structure and properties of sodium bismuth titanate lead-free piezoelectric ceramics. Prog. Mater. Sci. 2021, 122, 100836.
18. Wu, X.; Wu, C.; Yang, D.; Yin, J.; Wu, J. Strain regulation via composition and valence dependent substitution in BNT-based solid solutions. Adv. Powder. Mater. 2023, 2, 100079.
19. Lai, L.; Li, B.; Tian, S.; Zhao, Z.; Zhang, S.; Dai, Y. Giant electrostrain in lead-free textured piezoceramics by defect dipole design. Adv. Mater. 2023, 35, e2300519.
20. Li, T.; Liu, C.; Shi, P.; et al. High-performance strain of lead-free relaxor-ferroelectric piezoceramics by the morphotropic phase boundary modification. Adv. Funct. Mater. 2022, 32, 2202307.
21. Jing, R.; Zhang, L.; Hu, Q.; et al. Phase evolution and relaxor to ferroelectric phase transition boosting ultrahigh electrostrains in
22. Wang, Z.; Zhao, J.; Zhang, N.; et al. Optimizing strain response in lead-free (Bi0.5Na0.5)TiO3-BaTiO3-NaNbO3 solid solutions via ferroelectric/(non-)ergodic relaxor phase boundary engineering. J. Materiomics. 2023, 9, 244-55.
23. Malik, R. A.; Hussain, A.; Maqbool, A.; et al. Temperature-insensitive high strain in lead-free Bi0.5(Na0.84K0.16)0.5TiO3-0.04SrTiO3 ceramics for actuator applications. J. Am. Ceram. Soc. 2015, 98, 3842-8.
24. Zhang, X.; Jiang, G.; Liu, D.; Yang, B.; Cao, W. Enhanced electric field induced strain in (1-x)((Bi0.5Na0.5)TiO3-Ba(Ti,Zr)O3)-xSrTiO3 ceramics. Ceram. Int. 2018, 44, 12869-76.
25. Bai, W.; Li, L.; Li, W.; Shen, B.; Zhai, J.; Chen, H. Effect of SrTiO3 template on electric properties of textured BNT-BKT ceramics prepared by templated grain growth process. J. Alloys. Compd. 2014, 603, 149-57.
26. Wang, F.; Xu, M.; Tang, Y.; et al. Large strain response in the ternary Bi0.5Na0.5TiO3-BaTiO3-SrTiO3 solid solutions. J. Am. Ceram. Soc. 2012, 95, 1955-9.
27. Lalitha, K. V.; Koruza, J.; Rödel, J. Propensity for spontaneous relaxor-ferroelectric transition in quenched (Na1/2Bi1/2)TiO3-BaTiO3 compositions. Appl. Phys. Lett. 2018, 113, 252902.
28. Li, T.; Lou, X.; Ke, X.; et al. Giant strain with low hysteresis in A-site-deficient (Bi0.5Na0.5)TiO3-based lead-free piezoceramics. Acta. Mater. 2017, 128, 337-44.
29. Fan, P.; Zhang, Y.; Xie, B.; et al. Large electric-field-induced strain in B-site complex-ion (Fe0.5Nb0.5)4+-doped Bi1/2(Na0.82K0.12)1/2TiO3 lead-free piezoceramics. Ceram. Int. 2018, 44, 3211-7.
30. Wei, Q.; Zhu, M.; Zheng, M.; Hou, Y. Giant strain of 0.65% obtained in B-site complex cations (Zn1/3Nb2/3)4+-modified BNT-7BT ceramics. J. Alloys. Compd. 2019, 782, 611-8.
31. Ullah, A.; Ahn, C. W.; Hussain, A.; Lee, S. Y.; Lee, H. J.; Kim, I. W. Phase transitions and large electric field-induced strain in BiAlO3-modified Bi0.5(Na, K)0.5TiO3 lead-free piezoelectric ceramics. Curr. Appl. Phys. 2010, 10, 1174-81.
32. Janbua, J.; Niemchareon, S.; Muanghlua, R.; Vittayakorn, N. High strain response of the (1-x)(0.94Bi0.5Na0.5TiO3-0.06BaTiO3)-
33. Ge, R.; Zhao, Z.; Duan, S.; et al. Large electro-strain response of La3+ and Nb5+ co-doped ternary 0.85Bi0.5Na0.5TiO3-0.11Bi0.5K0.5TiO3-0.04BaTiO3 lead-free piezoelectric ceramics. J. Alloys. Compd. 2017, 724, 1000-6.
34. Wang, C.; Lou, X. High energy storage properties of 0.94Bi0.5Na0.5TiO3-0.06BaTiO3 ceramics by incorporating
35. Rahman, J. U.; Hussain, A.; Maqbool, A.; et al. Field induced strain response of lead-free BaZrO3-modified Bi0.5Na0.5TiO3-BaTiO3 ceramics. J. Alloys. Compd. 2014, 593, 97-102.
36. Cheng, R.; Xu, Z.; Chu, R.; Hao, J.; Du, J.; Li, G. Electric field-induced ultrahigh strain and large piezoelectric effect in Bi1/2Na1/2TiO3-based lead-free piezoceramics. J. Eur. Ceram. Soc. 2016, 36, 489-96.
37. Bafandeh, M. R.; Han, H.; Lee, J. Enhanced electric field induced strain in complex-ion Ga3+ and Ta5+-doped 0.93BNT-0.07BT piezoceramic. J. Electroceram. 2021, 47, 89-99.
38. Li, L.; Hao, J.; Xu, Z.; Li, W.; Chu, R.; Li, G. Large strain response in (Mn,Sb)-modified (Bi0.5Na0.5)0.935Ba0.065TiO3 lead-free piezoelectric ceramics. Ceram. Int. 2016, 42, 14886-93.
39. Hao, J.; Xu, Z.; Chu, R.; Li, W.; Fu, P.; Du, J. Field-induced large strain in lead-free (Bi0.5Na0.5)1-xBaxTi0.98(Fe0.5Ta0.5)0.02O3 piezoelectric ceramics. J. Alloys. Compd. 2016, 677, 96-104.
40. Schütz, D.; Deluca, M.; Krauss, W.; Feteira, A.; Jackson, T.; Reichmann, K. Lone-pair-induced covalency as the cause of temperature- and field-induced instabilities in bismuth sodium titanate. Adv. Funct. Mater. 2012, 22, 2285-94.
42. Jin, L.; Li, F.; Zhang, S.; Green, D. J. Decoding the fingerprint of ferroelectric loops: comprehension of the material properties and structures. J. Am. Ceram. Soc. 2014, 97, 1-27.
43. Shannon, R. D. Revised effective ionic radii and systematic studies of interatomic distances in halides and chalcogenides. Acta. Cryst. A. 1976, 32, 751-67.
44. Jing, R.; Chen, X.; Lian, H.; Qiao, X.; Shao, X.; Zhou, J. Comparative study on structure, dielectric, and piezoelectric properties of (Na0.47Bi0.47Ba0.06)0.95A0.05TiO3 (A = Ca2+/Sr2+) ceramics: effect of radii of A-site cations. J. Eur. Ceram. Soc. 2018, 38, 3111-7.
45. Pasha, U. M.; Zheng, H.; Thakur, O. P.; et al. In situ Raman spectroscopy of A-site doped barium titanate. Appl. Phys. Lett. 2007, 91, 062908.
46. Zhang, Y.; Zeng, K.; Fu, J.; Xie, A.; Fu, Z.; Zuo, R. Giant strains of 0.70% achieved via a field-induced multiple phase transition in BNT-based relaxor antiferroelectric ceramics. J. Eur. Ceram. Soc. 2023, 43, 4748-56.
47. Kreisel, J.; Glazer, A. M.; Bouvier, P.; Lucazeau, G. High-pressure Raman study of a relaxor ferroelectric: the Na0.5Bi0.5TiO3 perovskite. Phys. Rev. B. 2001, 63, 174106.
48. Kreisel, J.; Glazer, A. M.; Jones, G.; Thomas, P. A.; Abello, L.; Lucazeau, G. An X-ray diffraction and Raman spectroscopy investigation of A-site substituted perovskite compounds: the (Na1-xKx)0.5Bi0.5TiO3 (0 ≤x ≤1) solid solution. J. Phys. Condens. Matter. 2000, 12, 3267.
49. Bokov, A. A.; Ye, Z. Recent progress in relaxor ferroelectrics with perovskite structure. J. Mater. Sci. 2006, 41, 31-52.
50. Zhao, P.; Wang, H.; Wu, L.; et al. High-performance relaxor ferroelectric materials for energy storage applications. Adv. Energy. Mater. 2019, 9, 1803048.
51. Li, G.; Ge, G.; Lin, J.; et al. Eco-friendly cooling materials with synergistic behavior of electromechanical and electrocaloric effects based on constructing B-site defect field. Appl. Mater. Today. 2022, 26, 101332.
52. Jia, X.; Zhang, J.; Xing, H.; Wang, J.; Zheng, P.; Wen, F. Large electrostrain response in binary Bi1/2Na1/2TiO3-Ba(Mg1/3Nb2/3)O3 solid solution ceramics. J. Alloys. Compd. 2018, 741, 7-13.
53. Smolenskii, G. A. New ferroelectrics of complex composition. IV. SciSpace 1961. Available from: https://scispace.com/papers/new-ferroelectrics-of-complex-composition-iv-2wd1zsevvv [Last accessed on 7 Apr 2025]
54. Shi, J.; Zhao, Y.; He, J.; et al. Deferred polarization saturation boosting superior energy-storage efficiency and density simultaneously under moderate electric field in relaxor ferroelectrics. ACS. Appl. Energy. Mater. 2022, 5, 3436-46.
55. Yin, J.; Zhao, C.; Zhang, Y.; Wu, J. Ultrahigh strain in site engineering-independent Bi0.5Na0.5TiO3-based relaxor-ferroelectrics. Acta. Mater. 2018, 147, 70-7.
Cite This Article
How to Cite
Download Citation
Export Citation File:
Type of Import
Tips on Downloading Citation
Citation Manager File Format
Type of Import
Direct Import: When the Direct Import option is selected (the default state), a dialogue box will give you the option to Save or Open the downloaded citation data. Choosing Open will either launch your citation manager or give you a choice of applications with which to use the metadata. The Save option saves the file locally for later use.
Indirect Import: When the Indirect Import option is selected, the metadata is displayed and may be copied and pasted as needed.
About This Article
Copyright
Data & Comments
Data
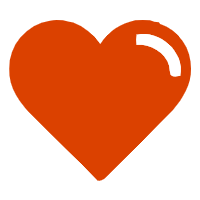
Comments
Comments must be written in English. Spam, offensive content, impersonation, and private information will not be permitted. If any comment is reported and identified as inappropriate content by OAE staff, the comment will be removed without notice. If you have any queries or need any help, please contact us at [email protected].