Stimuli-responsive biomedical polymeric films for tissue regeneration
Abstract
Tissue damage poses a significant burden on patients’ daily lives and has long driven the search for effective clinical treatments. Recent decades have witnessed the development of smart biomedical materials for satisfying specific requirements such as irregular shapes and dynamic microenvironments at defective sites. Stimuli-responsive polymeric films are well-positioned to play a considerable role in the exploitation of next-generation smart biomaterials for both soft and hard tissue regeneration. These polymeric films can be fabricated through diverse approaches and engineered with versatile structures and properties. Furthermore, responsive to stimuli such as temperature, water, and light, these films exhibit well-designed functions such as shape adaption, controlled drug release, and cell adhesion in vivo, effectively improving tissue regeneration. In this work, we review the recent advancements in stimuli-responsive biomedical polymeric films, beginning with the introduction of their fabrication methods. Subsequently, the stimuli-responsive mechanisms of the films are discussed and scrutinized in terms of structure and property variations. An overview of recent applications of stimuli-responsive films in tissue regeneration, including skin, cardiovascular, nerve, and bone regeneration, is provided. Finally, we further discuss the benefits and limitations of these smart films in practical applications, proposing our expectations and perspectives on future advancements of stimuli-responsive polymeric films.
Keywords
INTRODUCTION
Tissue damage, including skin injuries and bone defects resulting from diseases, surgeries, and traffic accidents, remains a significant global health concern[1-3]. For example, skin wounds like diabetic foot ulcers account for over 750,000 new cases annually among diabetic patients[4]. Tissue regeneration is a complex and comprehensive process that requires detailed consideration of the biomaterials’ effects on the shape, systemic response, and microenvironment of the damaged sites[5-7]. Over the past few decades, biomaterials used for replacing or repairing damaged tissues have undergone a progressive development from the laboratory to the clinic at an ever-increasing rate[8-10]. As science and technology advance, tissue regeneration increasingly requires biomaterials with more precise and personalized structures and properties to effectively match the repair and regeneration of the injured sites. Biomedical films, a class of planar biomaterials, are typically made from non-degradable polymers, biodegradable polymers, and synthetic or natural polymers[11-13]. Modern scientific advancements and technological innovations bring biomedical films with various effective preparation methods, including solvent casting[14], salt leaching[15], spin coating[12], electrospinning[16], and three-dimensional (3D) printing[17] to construct functionalized structures and properties of the films. Besides bioactivity, biocompatibility, and biodegradability, these biomedical polymeric films have been expansively developed with diverse stimuli-responsive functions such as shape adaption[18], actuation[19], adhesion[13,20], and drug release[21]. These features have significantly facilitated the exploration of new medical treatments for the regeneration of tissues including skin[22], cardiovascular tissue[23], nervous system[24], and bone[25].
Stimuli-responsive functions generally refer to the ability of polymers to alter their macro/microstructures and properties upon exposure to external stimuli such as heat[26,27], light[28,29], water[24,30], electricity[31], ultrasound[32], pH value[33], and mechanical compression[34]. These capabilities have garnered significant attention in biomedical applications[11,18,35]. As for mechanisms, the stimuli-responsive functionality of polymer materials stems from various internal changes, including the critical temperature transitions such as lower critical solution temperature (LCST) or upper critical solution temperature (UCST), reversible chemical reactions, non-covalent bonding such as hydrogen bonds, crystalline melting, electrostatic interactions, hydrophobic interactions, and the inclusion of small photo-responsive molecules like spiropyran (SP)[36]. These mechanisms can function independently or in concert, enabling the design of biomedical polymeric films with tunable stimuli-responsive behaviors. Research often reviews the stimuli-responsive biomaterials according to their external stimuli approaches[11]. However, the primary responses of polymer films to stimuli mainly involve alterations in their structures and properties. For example, exposure to water can induce movement in polymer chain segments, leading to transitions from a glassy state to a rubbery state and resulting in contraction, expansion, bending, and folding of the films[24,37]. In addition, stimuli such as high temperature can trigger phase transitions in polymer materials, such as liquid crystal phases or microphase separation, thereby altering the films’ mechanical properties[38]. In biomedical applications, stimuli-responsive polymeric films can also regulate drug release rates or cell adhesion based on changes in the biological environment, such as variations in pH value, enzyme concentration, or temperature[39].
In this work, we provide an introductory overview of stimuli-responsive biomedical polymeric films, as shown in Figure 1. Through the internal changes of molecular structures under external stimuli such as temperature, pH values, and light, the polymeric films have responsive structural and property variations, including contraction, expansion, bending, and folding, as well as stiffness change, controlled release, biodegradation, and cell adhesion. Nevertheless, the films can be further applied to various tissue regeneration and functionalized reconstruction - for example, regeneration of skin tissue, cardiovascular system, nerve, and bone. This review integrates the biological functions with structural and property changes in the films, which makes the design and controllability of the stimuli-responsive films more adaptable for specific tissue damages. Although many reviews have discussed stimuli-responsive mechanisms of smart materials, most focus on the various stimuli ways. In this review: (i) we discussed and compared advanced fabrication techniques for stimuli-responsive biomedical polymeric films developed in recent years; (ii) proposed a different classification framework based on structural and property changes to categorize response mechanisms comprehensively; (iii) based on this framework, we reviewed recent studies of stimuli-responsive biomedical polymeric films in diverse tissue regeneration scenarios, aligning more closely with their practical functional requirements in biomedical applications. Ultimately, we discussed and summarized the features of various soft and hard tissue damages, as well as proposing further development of the stimuli-responsive biomedical polymeric films for tissue regeneration, which offers new perspectives for the design and optimization of stimuli-responsive biomedical materials.
FABRICATION METHODS OF THE STIMULI-RESPONSIVE BIOMEDICAL POLYMERIC FILMS
Stimuli-responsive biomedical polymeric films are fabricated using various methods, including solvent casting, salt leaching, spin coating, electrospinning, and 3D printing technology. These methods allow for the precise design of film characteristics such as thickness, porosity, specific structures, flexibility, stiffness, adhesion, and swelling ability, tailored to the repair requirements of defective tissues. The characteristics and limitations of various fabrication methods are summarized in Table 1. Finally, the polymeric films repair or replace defective sites based on their stimuli-responsive structures and properties.
Characteristics and limitations of various fabrication methods
Fabrication methods | Characteristics | Limitations | Ref. |
Solvent casting | Simplicity, cost-effectiveness, good feasibility | Inability to control precise structures | [1,40-57] |
Salt leaching | Simplicity, cost-effectiveness, tunable pore architectures | Uncontrolled porous structural precision | [58-63] |
Spin-coating | Simplicity, cost-effectiveness, high controllability, and reproducibility | Material utilization inefficiency, non-planar substrate control | [4,12,64-73] |
Electrospinning technique | High surface area and distinctive microstructure, permeability | High-performance equipment | [16,74-78] |
3D printing technology | Personalized customization, precise controllability of macro/microstructures | Cost and equipment dependency | [17,24-25,79-89] |
Solvent casting represents a widely utilized methodology for polymeric film fabrication, which involves first dissolving the polymer in an appropriate solvent to form a homogeneous solution, followed by solvent evaporation within a mold to ultimately generate solid films [Figure 2A][40]. The designed properties such as mechanical strength and thermal stability of the films are determined by the selection of the polymer, e.g., poly(L-lactic acid) (PLLA)[41], polycaprolactone (PCL)[42], poly(vinyl alcohol) (PVA)[43], polyethylene glycol (PEG)[44], polyethylene oxide (PEO)[45], and solvent, e.g., N,N-dimethylformamide (DMF)[46], N,N-dimethylacetamide (DMAC)[47], tetrahydrofuran (THF)[41], dichloromethane (DCM)[48], chloroform[49], and toluene[50]. A critical step in the preparation process is ensuring the polymer dissolves completely in the solvent under uniform stirring[51]. Bioactive components or drug particles can also be incorporated at this stage[52-54]. After solvent evaporation, the film is carefully peeled off for visual inspection to assess its integrity, color, and opacity. By adjusting the concentration and evaporation temperature of the solution, the thickness and other properties of the films can be controlled. Additionally, by designing the molds, films of various shapes, such as rectangles and tubes, can be produced for application to different organs [1,42]. Di et al. constructed flexible composite films using this method, enhancing the films’ high-temperature stability and mechanical properties[55]. Sun et al. employed the solvent-casting self-assembly approach to fabricate a composite responsive film with enhanced mechanical properties[56]. Silva et al. prepared biopolymer nanocomposite films using the solvent casting method, which demonstrated good uniformity, semi-transparency, and thermal stability[57]. While solvent casting offers a simple and feasible method for producing robust films, its main limitation is the inability to control the casting of porous and other precise structures.
Figure 2. Fabrication methods of stimuli-responsive polymeric films. (A) Solvent casting to prepare photosensitive polymer films. Copyright 2023, MDPI[40]; (B) Preparation of porous films by salt leaching method. Copyright 2023, Elsevier[58]; (C) Main process for preparing thin films using the spin coating method. Copyright 2021, Royal Society of Chemistry[12]; (D) Electrospinning method to prepare superhydrophobic films. Copyright 2024, American Chemical Society[76]; (E) 3D printing technology to fabricate films with controlled thicknesses and structures. Copyright 2023, Elsevier[81].
Salt leaching is considered one of the oldest methods for fabricating porous films. This technique builds on the solvent casting method, with inorganic salts added to the solvents to dissolve biodegradable polymers
Spin-coating is a highly time-efficient and cost-effective technique for producing films with flat and smooth surfaces [Figure 2C][12,64]. This method involves pouring a uniformly dispersed polymer solution onto a rotating substrate, where centrifugal forces spread the solution evenly across the substrate, controlling the film thickness from tens of nanometers to several micrometers[65,66]. After solvent evaporation or other subsequent treatment steps, the polymeric films can be obtained. Research over the decades has clarified the relationship between fabrication variables and film thickness, including rotation speed, surface tension, and fluid viscosity[67]. In addition, the solvent evaporation rate and temperature can affect the crystallinity and surface morphology of the films[68]. By optimizing these parameters, the properties and structure of spin-coated films can be effectively controlled. Spin-coating is widely used to prepare monolayer and multilayer thin films, including freestanding (FS) nanosheets and membranes. In the biomedical field, spin-coated films are utilized for applications such as wound dressings[69], cell culture substrates[70], drug delivery devices[71] and others[72]. Theocharidis et al. developed a strain-programmable adhesive film with two layers using the spin-coating method for diabetic wound healing[4]. Despite its advantages such as low cost, simplicity, speed, high controllability, and reproducibility, the spin-coating method has limitations in material efficiency and control over non-planar substrates, which restrict its broader application in tissue regeneration[12,73].
The electrospinning technique has gained widespread interest in the biomedical field[74]. An electrospinning device generally comprises a spinneret with a syringe device, electronic equipment to control solution release, a collector to receive the electrospun materials, and a power supply to generate an electric field between the spinneret and the collector [Figure 2D][75,76]. The polymer solution is first placed into the syringe and then extruded as a liquid jet from the spinneret, where it forms polymer filaments under the electric field followed by being collected sequentially via the rotating (oriented nanofibers) or not rotating (nonoriented nanofibers) collectors[77]. The performance of electrospinning films is mainly influenced by external parameters such as humidity and temperature, as well as internal parameters including applied voltage, working distance, conductivity, and the viscosity of the polymer solution[78]. Electrospinning can produce ultra-fine polymer fibers with diameters ranging from micro to nano scales by applying a high electric field, resulting in films with a high surface area and distinctive microstructures. In particular, electrospinning films have been widely employed for biomedical applications due to their structural resemblance to the extracellular matrix (ECM), and their porous structure, which allows nutrient molecule permeability[16]. Furthermore, electrospinning technology can also be integrated with other manufacturing technologies, such as 3D printing and microfluidics, to fabricate multilayer composite films or hybrid materials with enhanced performance and functionality for tissue regeneration. Wang et al. designed a double-gradient biomedical film with a three-layer structure using electrospinning technology[75]. The multilayer nanofiber structure endowed the film with robust elasticity and unidirectional fluid transport capability, which effectively improved wound healing. However, the major limitations of this method include the need for high-voltage equipment and challenges associated with packaging, shipping, and handling[78].
3D printing technology has emerged as an effective tool for fabricating films tailored to personalized requirements, garnering significant interest in the biomedical field [Figure 2E][80,81]. In recent years, 3D printing incorporating computer-aid design has been developed for precisely controlling macro/microstructures, including the geometric shapes, thickness, and pores of the materials[25,82,83]. This method utilizes a polymeric solution for fused deposition modeling of films under specific conditions, e.g., high/low temperature, light or ultrasound, followed by removing the solvent (if present)[24,84]. During the printing process, parameters such as printing temperature, extrusion velocity, and nozzle movement speed directly affect the construction performance of the films[85,86]. The rational design of the film structure can yield films with varying functionalities and properties[87]. For instance, films can be designed with a porous structure to increase surface area, achieving improved cell adhesion and migration, thereby facilitating tissue regeneration[17]. Compared to other fabrication methods, the strengths of 3D printing include customized fabrication, rapid prototyping, and the ability to create complex structures. Liu et al. used fused deposition modeling (FDM) 3D printing technology to prepare a shape-memory film with good responsivity to temperature and water for minimally invasive treatment of various tissue defects[79]. Wang et al. employed 3D printing technology to fabricate a thin film with Janus structures, capable of transforming from a planar configuration to curled 3D structures for vascular tissue regeneration[88]. Huang et al. developed a 3D-printed wound dressing film embedded with calcium phosphate nanoparticles, which degrades in response to pH changes, enabling the controlled release of antimicrobial agents[89].
STIMULI-RESPONSIVE MECHANISMS
In this review, we categorize the mechanisms of stimuli-responsive biomedical polymeric films based on variations in their structures and properties, primarily triggered by stimuli such as temperature, light, electric fields, pH value, and ultrasound [Table 2]. The responsive mechanisms to these stimuli are crucial for developing and implementing the functionalized biomedical applications of these films.
Responsive structural and property variations of stimuli-responsive biomedical polymeric films
Responsive structural variations | Polymer substrates | Fabrication methods | Stimuli | Applications | Ref. |
Contraction | Poly(N-isopropylacrylamide) (PNIPAM) + Chitosan | Solvent casting | Temperature | Skin | [19] |
Polyvinyl butyral (PVB) | Solvent casting | Temperature | Skin | [22] | |
PCL + PEG | Solvent casting | Electricity | Skin | [44] | |
P(AAm-co-AAc) | Solvent casting | Elasticity | Skin | [90] | |
PEG + PEO | Solvent casting | Water | Skin | [45] | |
PEG + PEO | Solvent casting | Water | Nerve | [24,30] | |
Expansion | PVA + Chitosan | Solvent casting | Light | Skin | [91] |
PCL | Solvent casting | Temperature | Cardiovascular | [92] | |
PCL + PEG + Polyurethane (PU) | Solvent casting | Temperature and/or water | Cardiovascular | [79] | |
Poly(octamethylene maleate (anhydride) citrate) | Solvent casting | Force | Cardiovascular | [93] | |
PCL | Solvent casting | Temperature | Bone | [26-27] | |
Bending and folding | PCL + GelMA | Electrospinning | Temperature | Cardiovascular | [24,37] |
PCL + GelMA | 3D printing | Water | Cardiovascular | [88] | |
Polymethylmethacrylate (PMMA) | Spin coating | Temperature | Nerve | [24] | |
Poly(lactide-co-trimethylene carbonate) (PLATMC) | Electrospinning | Temperature | Nerve | [94] | |
PCL + PVA | Solvent casting | Water | Nerve | [95-96] | |
Chitosan + Acrylic acid-N-hydroxysuccinimide (AAc-NHS) | Solvent casting | Water | Nerve | [97] | |
Polypyrrole | Spin coating | Electricity | Nerve | [31] | |
PCL | 3D printing | Temperature | Bone | [25] | |
Poly (lactide-co-glycolide)-b-poly(ethylene glycol)-b-poly(lactide-co-glycolide) (PELGA) | Solvent casting | Temperature | Bone | [98] | |
isotactic polypropylene (iPP) + PNIPAm | Solvent casting | Temperature | hernia | [99] | |
Stiffness | PCL + PEG + PU | Solvent casting | Temperature and/or water | Cardiovascular | [79] |
Controlled release | Poly(vinylidene fluoride) (PVDF) + PEO | Electrospinning | Electricity | Skin | [21] |
PEG | Solvent casting | ROS | Skin | [100] | |
PVA | Solvent casting | NIR and/or pH value | Skin | [101] | |
PCL | Electrospinning | Enzyme | Cardiovascular | [102] | |
PCL + Gelatin | Electrospinning | Electricity | Cardiovascular | [103] | |
Biodegradation | Polyacrylic acid (PAA) + Polyallylamine hydrochloride (PAH) | Solvent casting | pH value | / | [36] |
Poly(lactic-co-glycolic acid) (PLGA) + PAH | Solvent casting | Ultrasound | Bone | [104] | |
Cell adhesion | PLLA | Solvent casting | Ultrasound | Skin | [41] |
PCL + PEG | Solvent casting | Electricity | Skin | [44] | |
PCL + GelMA | Electrospinning | Temperature | Cardiovascular | [24,37] | |
PCL + GelMA | 3D printing | Water | Cardiovascular | [88] | |
PLLA | Solvent casting | Temperature | Bone | [105] | |
PLGA | Salt leaching | Opto-acoustic | Bone | [106] | |
Poly(dimethylsiloxane) (PDMS) | Solvent casting | Electricity | Bone | [107] | |
PVDF | Solvent casting | Magnetoelectric | Skeletal muscle | [52-54] |
Responsive mechanisms of structural variations
Contraction
Contraction is a common structural change in stimuli-responsive films, enabling volume reduction under specific stimuli, which in turn facilitates interactions with tissues. Responsive contraction allows the films to adaptively wrap around or attach to target tissues in dynamic environments. Considering that most organs and tissues exist in a wet environment, water serves as a benign and bio-friendly stimulus, offering inherent advantages[108]. Yi et al. reported a water-responsive polymer film that remained stable under dry ambient conditions [Figure 3A][30]. When exposed to water, the film rapidly contracted, transforming into a soft and stretchable state as water molecules disrupted the polymer crystallites. This feature allowed the film to interactively wrap tissues, enhancing effective attachment and adaptability to moist biological environments for tissue repair. Temperature, a controllable and measurable stimulus, is widely used to induce contraction in thermally responsive films. Blacklow et al. reported an intelligent wound dressing comprising a thermally responsive adhesive hydrogel film containing PNIPAm [Figure 3B][19]. This film could adhere firmly to the skin and actively contract upon exposure to skin temperature, promoting wound healing through the mechanical force generated by contraction. Light as a stimulus offers several notable advantages, including immediacy and high precision. Notably, light enables a non-contact control mechanism to achieve the materials structure changes using small photo-responsive molecules[29]. For example, the protonated merocyanine (MCH+) groups in the film undergo isomerization to the spiropyran form after exposure to light of a specific wavelength. This transition dehydrates the polymer chains, causing a responsive contraction of the film [Figure 3C][109-112]. In addition, light, especially near-infrared (NIR) light, can induce stimuli-responsive performance via photothermal effects in thermally responsive materials. These materials can absorb light energy, raising temperature and subsequently activating temperature-based stimulus-responsive mechanisms[113-115]. However, it should be noted that light as a stimulus has limitations due to its shallow tissue penetration depth (less than 2 cm), which restricts its effective applications for deep tissue regeneration[116,117].
Figure 3. Mechanisms of responsive contraction and expansion of the films. (A) Water-responsive supercontractile polymer films due to crystalline transition. Copyright 2023, Springer Nature[30]; (B) Thermo-responsive contraction adhesive hydrogel films due to critical temperature transitions. Copyright 2019, American Association for the Advancement of Science[30]; (C) Light-induced contraction and expansion mechanism from a hydrogel film with photo-responsive spiropyran molecules. Copyright 2024, Springer Nature[112]; (D) Fabrication of light-responsive films with controlled expansion due to hydrophobic-hydrophilic transition. Copyright 2019, John Wiley and Sons[91].
Expansion
Expansion is commonly observed for stimuli-responsive solids, particularly for applications in supporting or filling defective tissues[35,118]. In the case of films, triggered expansion has been shown to remove the interfacial water between the film and tissue, alter the shape into the desired 3D structure, and control the water permeability and swelling ratio. Water and light are widely employed stimuli for inducing responsive expansion. Water-triggered mechanisms include the effects of hydrophilic functional groups, polymer network expansion, and osmotic pressure[95,96]. Light-induced expansion typically occurs by altering molecular characteristics, e.g., conformation, polarity, amphiphilicity, and charge[119]. Our group reported an adhesive biomedical film with water-triggered expansion[13]. The introduced black phosphorus nanosheets in the film could degrade ions to bond with the functional groups of polymer chains and then significantly improve the water absorption of the film. Upon being adhered to the defective tissue, the films had water-triggered expansion to remove the interfacial barrier and achieve robust adhesion for various biomedical functions. Liu et al. developed a high-expansion amphiphilic dynamic thermoset polyurethane (DTPU) that expanded when water permeated into the DTPU network[79]. The expansion mismatch drove the 2D film to deform into the desired 3D structure during minimally invasive implantation. Ryplida et al. reported a light-responsive thin film comprised of TiO2 polydopamine, perfluorosilicon carbon dot, chitosan, PVA, and tannic acid for skin tissue replacement [Figure 3D][91]. This film can be stimulated using Ultraviolet–visible spectroscopy (UV-vis) light irradiation to control hydrophobic-hydrophilic transition, significantly enhancing both water permeability and expansion ratio.
Bending and folding
Responsive bending and folding of the films are usually employed for biological tissues with curved or irregular surfaces. Currently, there are two primary methods to achieve directed bending or folding changes in polymeric films, including: (1) the design of heterogeneous polymer networks with layered, oriented, gradient, and anisotropic structures; and (2) the complex control and manipulation of non-uniform external stimuli to achieve pre-heterogenization of components[112]. By engineering internal structures or modulating component interactions with external stimuli such as temperature, light, or pH, the films undergo controlled bending/folding motions, enabling them to conform to damaged tissue surfaces. Lanzalaco et al. reported a humidity- and temperature-responsive film for hernia repair surgeries, using iPP as the substrate material and a thermo-responsive poly(N-isopropylacrylamide-co-N, N’-methylenebisacrylamide) (PNIPAAm-co-MBA) hydrogel as the coating layer [Figure 4A][99]. The reversible folding/unfolding behavior of the film was driven by the creation of hydrogen bonds among amide groups inside PNIPAAm and the destruction of hydrogen bonds with water molecules, enabling the structural changes in response to environmental stimuli. Additionally, Zhang et al. proposed a flexibly bending PMMA film with shape memory capability as a smart wound electrode [Figure 4B][24]. Triggered by the melting transition of the shape-memory polymer substrate, the film undulates toward damaged nerves when immersed in physiological saline solution at 37 °C, achieving targeted conformal contact. The stimuli-responsive polymeric film can also deform with the nerve, minimizing constraints and further achieving biofunctions such as sensing and repairing. Ultrasound, as a non-contact stimulus, exhibits superior tissue penetration (greater than 5 cm) compared to light-based triggers[120]. Fundamentally, cavitation and acoustic radiation force are the primary mechanisms that can generate heat or molecular switches to induce structural changes in the films[32,33]. High-Intensity Focused Ultrasound (HIFU) is an advanced technique that combines powerful thermal focusing and efficient cavitation effects to concentrate ultrasound energy precisely on a targeted area. HIFU reduces energy loss in surrounding tissues and enhances the control of localized variations of responsive films for applications such as cancer treatment and tissue ablation[32,121,122]. Zhu et al. used HIFU to trigger shape recovery in polyurethane urea-based implant devices through a crystallization transition at high temperatures [Figure 4C][123]. This study highlighted the deep energy penetration, safety, and rapid change of the HIFU-responsive film for medical applications.
Figure 4. Mechanisms of responsive bending and folding of the films. (A) Humidity and temperature-responsive films with controlled bending and folding due to the creation and destruction of hydrogen bonds. Copyright 2020, John Wiley and Sons[99]; (B) A 3D twining film based on the melt transition of the shape memory substrate. Copyright 2019, American Association for the Advancement of Science[24]; (C) Responsive folding implantation through crystallization transition at high temperature triggered via HIFU. Copyright 2024, Springer Nature[123]. HIFU: High-Intensity Focused Ultrasound.
Responsive mechanisms of property variations
Besides structural changes, variations in the properties of stimuli-responsive polymeric films are also crucial for adapting to physiological environments and regenerating defective tissues. We categorize these changes in terms of stiffness, controlled release, biodegradation, and cell adhesion. These variations in properties are instrumental for tissue regeneration and can be triggered by stimuli such as temperature, light, electricity, mechanical forces, and ultrasound.
Stiffness
Temperature is a well-known factor that can significantly alter the modulus of a polymer system. For example, when the temperature exceeds the LCST, the PNIPAAm polymer undergoes a volumetric reduction but an increase in modulus due to the formation of intramolecular hydrogen bonds between C = O and N-H groups within the PNIPAAm chains, leading to a compact, hydrophobic collapse of the polymer network[124]. This process results in a contractive and fixing effect that is highly beneficial for dynamic tissue wound healing. In addition, the water-triggered expansion of polymer networks can induce a stiffness transition from soft to tough. Liu et al. developed a shape memory polyurethane (SMPU) that enables a transition from a soft to a rigid state during shape recovery [Figure 5A][125]. Upon hydration, this SMPU underwent chain rearrangement, enhancing microphase separation and promoting stress transfer. Liu et al. developed a 3D-printed composite film with swelling-stiffening properties and programmable deformation [Figure 5B][79]. The water-triggered stiffness transition originated from microphase separation formed between hydrophilic and hydrophobic chain segments upon hydration. Furthermore, the hydrated pattern in the film morphed into the desired 3D structure because of swelling mismatch in water, which has the potential for minimally invasive implantation. Yang et al. reported a hybrid film impregnated with a supercooled salt solution[38]. Upon instantaneous nucleation stimulus, the film switched from a transparent and soft state to a white and rigid state, with its hardness increasing from 15 kPa to 385 MPa. The mechanism exploited the phase transition and robust physical metastability of the liquid, where the components interacted at the molecular level, enhancing the film’s functionality for specific applications.
Figure 5. Mechanisms of responsive variation in stiffness of the films. (A) Water-triggered stiffening of shape-memory polyurethane due to chain rearrangement and enhanced microphase separation. Copyright 2022, John Wiley and Sons[125]; (B) 3D printing porous films in response to temperature and water caused by microphase separation. Copyright 2024, Springer Nature[79].
Controlled release
Controlled release is a crucial function of biomedical films, adapting to dynamic microenvironments, such as inflammation or reactive oxygen species (ROS), and providing targeted delivery of nutrients or drugs[126,127]. Cheah et al. published an electrically active composite film to control the release of proteins
Figure 6. Mechanisms of responsive variation in controlled release, degradation and cell adhesion of the films. (A) Controlled protein release from PEDOT-based composite film under electrical stimulation. Copyright 2023, Elsevier[128]; (B) Responsive drug-release systems through ultrasound-induced cleavage of covalent and non-covalent bonds. Copyright 2021, Springer Nature[132]; (C) Ultrasound-responsive biodegradation and drug release due to the deconstruction of the Poly(lactic-co-glycolic acid) (PLGA) layer. Copyright 2024, Elsevier[104]; (D) Force-stimulated microstructure growth on the surface of a double-network hydrogel film for directional cell growth on demand. Copyright 2022, Springer Nature[133]; (E) NIR-triggered polymeric films modulate cell behaviors through transformable topographies. Copyright 2020, Oxford University Press[134]. PEDOT: poly(3,4-ethylenedioxythiophene); NIR: near-infrared.
Biodegradation
Controlled biodegradation of biomaterials is a key objective pursued by researchers in the biomedical field. Ultrasound has emerged as an effective tool that utilizes radiation force to deconstruct the macroscopic structures of films, aiding in the controlled release and biodegradation of polymeric systems. Song et al. demonstrated that ultrasound could specifically break down a PLGA layer, allowing for the controlled release of active components and enhancing the biodegradation of the polymer [Figure 6C][104]. In addition, pH values in the dynamic microenvironment can also alter the biodegradation of specific polymers[33]. For example, polyelectrolyte multilayer films (PEMs) made from PAA and PAH exhibit different properties at various pH values due to the dependence of charge density on the pH values of the solution. The biodegradation of the PEMs’ pores could be controlled by adjusting the pH values of the processing solution[36]. PAA can also be protonated at low pH values of the microenvironment, contributing to the cleavage of polymer chains[135]. These controllable behaviors underscore the versatility and adaptability of polymeric films in responding to environmental conditions within the body, aligning with the goals of effective tissue regeneration.
Cell adhesion
Cell adhesion is an integral initial phase in tissue regeneration, which can be meticulously manipulated via stimuli-responsive polymeric films. Studies have shown that cells exhibit strong adhesion to relatively hydrophobic surfaces[136]. Mu et al. reported a force-stimulated microstructure growth on the surface of hydrogel films [Figure 6D][133]. The mechanism is related to the stress/strain-induced bond cleavage followed by the rapid free radical polymerization of multiple monomers in a double-network (DN) hydrogel. This work showed that the surface of the hydrogel films can be engineered to improve cell adhesion and direct cell growth on demand. Zhao et al. proposed a NIR-triggered polymeric film to modulate the geometries and functions of endothelial cells by transformable topographies [Figure 6E][134]. Cell adhesion and migration could be promoted by the temporary anisotropic and permanent isotropic topographies on the film surface. This approach provides a powerful tool for precisely modulating cell behaviors and interactions for tissue regeneration.
APPLICATIONS FOR TISSUE REGENERATION
The variations in both the structure and properties of stimuli-responsive polymeric films are orchestrated through an array of mechanisms such as crystalline melting, reversible chemical reactions, non-covalent bonding, electrostatic interactions, hydrophobic interactions, and so on. These sophisticated mechanisms engender distinct functional adaptations, including contraction and controlled release, which are pivotal in the films’ applications. Leveraging these tailored responses, stimuli-responsive films have been meticulously developed to address defects in critical biological systems such as the skin, cardiovascular, nerve, and bone, facilitating tissue regeneration. This advanced engineering not only underscores the films' versatility but also their profound applicability in pioneering regenerative medicine strategies to restore and rejuvenate damaged tissues.
Skin regeneration
Skin, the largest organ of the human body, serves as a critical barrier against toxic substances, pathogens, and microorganisms[137]. Both acute and chronic skin injuries pose significant clinical challenges; for instance, severe diabetic foot wounds can lead to amputation or even fatal outcomes for patients[138-140]. Traditional wound dressings have been widely utilized for their essential functions, including antibacterial properties, hemostasis, and drug delivery[141,142]. Recently, stimuli-responsive polymeric films have gained increasing popularity in skin repair owing to their controllable structures and properties[143].
Structural variations
In terms of structural variations, responsive contraction of the biomedical films has drawn great attention, particularly for its ability to rapidly tighten wounds, reducing hemorrhaging and preventing further expansion of the injury. Blacklow et al. reported a thermally responsive adhesive film with high stretchability, toughness, tissue adhesion, and antimicrobial properties, which could tightly adhere to the skin and then actively contract the wound because of skin temperature [Figure 7A][19]. Dong et al. developed a temperature-responsive PVB fibrous film that incorporated a “stiff-elastic” binary component, which demonstrated a 70.0% contraction rate after exposure to 37 ℃ for 12 h, showing its potential in promoting wound closure and tissue regeneration [Figure 7B][22]. However, the 12-hour exposure period could increase the risk of infection and scarring. Additionally, Theocharidis et al. proposed a strain-programmed patch for diabetic wound regeneration, which contracted through a water-triggered shape-memory mechanism, enhancing wound healing in both in vitro and in vivo studies[4]. Nevertheless, water stimulus leads to a high-humidity environment that may impair skin integrity and increase the risk of ulceration. Ma et al. designed a highly contractile poly(acrylamide-co-acrylic acid)[p(AAm-co-AAc)] hydrogel film by storing and releasing elastic potential energy in the polymer network [Figure 7C][90]. The contractile strength of the film was 40 kPa, significantly higher than that of most hydrogels and even biological muscles. This force effectively promotes wound closure; however, caution must be exercised to avoid exceeding the wound’s tolerance to prevent secondary damage or scarring.
Figure 7. Skin regeneration with stimuli-responsive films. (A) Thermally responsive adhesive films with active contraction at the wound. Copyright 2019, American Association for the Advancement of Science[19]; (B) A temperature-responsive fibrous film with a 70.0% contraction rate for wound closure. Copyright 2024, John Wiley and Sons[22]. (C) A highly contractile NIR-responsive polymer film for accelerated wound healing. Copyright 2020, American Association for the Advancement of Science[90]; (D) A self-powered wound dressing film featuring a “Lock-ON/OFF” electric field-driven controlled release system combined with electrical stimulation therapy for skin regeneration. Copyright 2024, John Wiley and Sons[21]; (E) A NIR/pH dual-responsive PVA-based composite film with controlled drug release for cutaneous wound healing. Copyright 2023, American Chemical Society[101]. NIR: near-infrared; PVA: poly(vinyl alcohol).
Property variations
For property variations, controlled release of the drugs and growth factors are widely considered in film design for skin repair. Paula et al. constructed a PEG-based polyurethane-urea film modified with ROS-responsive oxanorbornene β-aminoacrylate bonds[100]. This film disrupted its structure and released pre-loaded silver sulfadiazine (AgSD) through a light/ROS responsive mechanism, significantly improving burn wound healing. Moeinipour et al. fabricated a polymer film composed of PVA, tannic acid (TA), and hydrogen-bonded organic frameworks (HOFs) loaded with metronidazole. This film employed the temperature- and pH-sensitive hydrogen bonds to enable the controlled release of metronidazole at wound sites, facilitating chronic wound treatment[144]. Sun et al. prepared a self-powered wound dress film using PVDF as a self-powered system and incorporating a “Lock-ON/OFF” electric field-driven controlled release mechanism [Figure 7D][21]. The film leveraged its piezoelectric effects to create the electric field for modulating the electrostatic balance between the drugs and their carrier, enabling on-demand drug release for improved wound regeneration. Zha et al. designed a NIR/pH dual-responsive Cu-Humic acid nanoparticles-based PVA hydrogel films loaded with SEW2781 agent for cutaneous wound healing, where the Cu ions were released in response to changes in pH values [Figure 7E][101]. Clinical translation of the stimuli-responsive films has been reported for skin wound healing. For example, Systagenix Wound Management Manufacturing Limited developed a responsive film product, Promogran®, to manage chronic non-healing wounds, which has completed a clinical trial involving 276 patients[145]. The film can transform into a soft, biodegradable gel upon contact with wound exudate, maintaining a moist healing environment and promoting tissue repair. Moreover, KoCarbonAg® Antimicrobial Dressing (Biomedical Carbon Technology Co., Ltd.) is also a humidity-responsive film product with enhanced antibacterial efficacy for wound healing[146]. However, most stimuli-responsive polymeric films are still limited by scalable manufacturing, regulatory compliance, and long-term biocompatibility for clinical applications.
Cardiovascular regeneration
Cardiovascular diseases, such as myocardial infarction (MI), atherosclerosis, and aneurysms, represent a severe global health threat associated with high disability and mortality rates[147]. Conventional treatments including pharmacological therapy and surgical intervention have respective limitations on obstructed blood circulation pathways and invasive damage to tissues[148]. Alternatively, advancements in stimuli-responsive polymeric films offer new solutions to these challenges.
Structural variations
The responsive structural changes of these films enable minimally invasive surgery to avoid tissue trauma or infection. Montgomery et al. prepared an elastic film with engineered lattice microstructures using a biodegradable shape memory polymer {poly[octamethylene maleate (anhydride) citrate]} [Figure 8A][93]. This film could be programmed and temporarily fixed to a small tubular shape, followed by being injected through a 1-mm incision into the infarcted heart region. After that, the film could expand at the defective site and serve as a cardiac patch without compromising cell viability or function. Li et al. synthesized a shape memory and self-healing polyurethane film for treating myocardial infarction [Figure 8B][92]. The semicrystalline PCL segments and interchangeable and antioxidative diselenide bonds contributed to good shape memory effects and rapid self-healing under 405 nm irradiation. This feature enabled the deployment of film through a 10-mm diameter trocar into a dynamic heart model, followed by laser-induced expansion into a larger single patch at the targeted site, promoting myocardial repair and maintaining cardiac function. However, concerns remain regarding the stimuli used in minimally invasive surgery, such as high temperature and UV light, due to their limited penetrativity and safety.
Figure 8. Cardiovascular regeneration with stimuli-responsive films. A. Shape memory porous film stents for minimally invasive cardiac implantation. Copyright 2017, Springer Nature[93]; (B) Shape memory and self-healing polyurethane films for myocardial infarction treatment. Copyright 2023, Royal Society of Chemistry[92]; (C) An electrospinning film incorporating SA and biodegradable polyurethane for controlled drug release. Copyright 2024, American Chemical Society[102]; (D) Flexible responsive multilayer films for cardiac signal detection and drug release. Copyright 2022, Elsevier[103]; (E) Thermally responsive films enhanced endothelial cell adhesion and proliferation. Copyright 2018, John Wiley and Sons[37].
Property variations
The responsive property variation of the polymeric films offers effective methods for controlled drug delivery and release. Liu et al. developed an electrospinning film incorporating salvianolic acid (SA) and biodegradable polyurethane to repair cardiovascular system damage [Figure 8C][102]. SA was released in response to the surrounding microenvironment, facilitating cellular infiltration and tissue regeneration. Huang et al. fabricated a flexible responsive multilayer film based on the synergism of polyurethane and nanofibers for cardiac signal detection and drug release [Figure 8D][103]. When abnormal symptoms were detected, electrical stimulation would be applied to control drug release rapidly for cardiac treatment. Inadequate endothelialization remains a major limitation in achieving effective treatment with implanted grafts. Zhao et al. reported thermally responsive films that can alter their planar shape to a 3D tubular shape, thereby enhancing endothelial cell adhesion and proliferation [Figure 8E][37]. When designing stimuli-responsive films with layered or microstructure surfaces for cardiovascular applications, mechanical compatibility with native cardiac tissues must be carefully considered because excessive rigidity may lead to further damage to the cardiovascular tissues, potentially causing arrhythmias and other complications. The evolving landscape of stimuli-responsive polymeric films offers significant promise in addressing the complexities associated with cardiovascular diseases, combining minimally invasive approaches with precise control over structure and properties. Nevertheless, clinical applications of these smart films on heart-like deep tissues are still challenging.
Nerve regeneration
Nerve damage significantly impairs patients’ cognitive abilities, motor functions, and daily activities, profoundly diminishing their quality of life[149]. Damage to nerves is primarily classified into central nervous system (CNS) and peripheral nervous system (PNS) injuries. CNS damage often results in irreversible injuries, such as permanent paralysis and sensory loss, because the limited regenerative capacity of neurons, formation of glial scars, and chronic inflammation could obstruct repair[150]. Current treatment strategies primarily include pharmacological interventions (e.g., anti-inflammatory drugs and neurotrophic factors), physical therapies (e.g., electrotherapy), and rehabilitation training. Deep anatomical location, complex structures and immune issues of the CNS limit the applications of stimulus-responsive polymer films for nerve regeneration. In contrast, PNS, distributed across the body surface and viscera with axons ensheathed by myelin and endoneurial tubes, benefit from a permissive regenerative microenvironment. Conventional treatments for PNS often involve surgical suturing or autologous nerve grafts, but these surgical procedures are intricate and pose risks of further damage. Recent decades have witnessed the fast development of the stimuli-responsive polymeric films applied in nerve regeneration, especially for PNS regeneration[151,152].
Structural variations
Responsive structural changes like contraction and warping could improve the interface interaction between the materials and tissues. Zhang et al. fabricated a mimosa-inspired film composed of chitosan and AAc-NHS lipid via gradient crosslinking with glutaraldehyde [Figure 9A][97]. This film could curl into a conduit under water stimulation, offering good biocompatibility, diameter adaptability, and seamless biointegration. In vitro and in vivo studies further demonstrated its efficacy in promoting nerve repair while reducing regeneration time and minimizing suture-related inflammation. Wang et al. developed a self-forming multichannel nerve guidance conduit made of PLATMC polymer synthesized from lactic acid (LA) and trimethylene carbonate (TMC), which could alter its shape from an electrospinning film to a conduit under temperature stimulation [Figure 9B][94]. This self-forming film and the nerve guidance conduit enhanced cell growth and improved the regeneration of rat sciatic nerve defects.
Figure 9. Nerve regeneration using the stimuli-responsive films. (A) Mimosa-inspired water-responsive films with good biocompatibility, diameter adaptability and seamless biointegration. Copyright 2023, John Wiley and Sons[97]; (B) A multichannel nerve guidance conduit triggered by body temperature for enhancing cell growth and improving nerve regeneration. Copyright 2020, Springer Nature[94]; (C) A responsive cascade drug delivery film scaffold for PNS injury repair. Copyright 2024, Royal Society of Chemistry[153]; D. Water-responsive polymer films with wrapping ability and mechanical compatibility for function reconstruction. Copyright 2023, Springer Nature[30].
Property variations
Besides responsive wrapping and self-forming of the film, responsive property variations like controlled release are useful for nerve generation and functional construction. Shan et al. developed a responsive cascade drug delivery film scaffold (RCDDS) to enhance PNS injury repair [Figure 9C][153]. This system employed a two-stage encapsulation with vitamin B12 (VB12) and nerve growth factors (NGFs), where the controlled release was regulated by ultrasound-responsive disassembly of polymer chains, thereby aligning treatments with the individual recovery period. Additionally, research has demonstrated that the intrinsic mechanical properties of biomaterials profoundly influence cell behaviors[154]. Roth et al. developed protein-engineered hydrogels encapsulated with human induced pluripotent stem cell (hiPSC)-derived neural progenitor cells (NPCs). Due to the fast stress relaxation rates of the hydrogels, the NPCs exhibited longer neurite projections, reduced metabolic activity, and higher gene expression[155]. Therefore, stiffness variations were further studied based on the responsive-wrapping film. Yi et al. designed a water-responsive supercontractile polymeric film made from PEO and PEG-α-cyclodextrin [Figure 9D][30]. The film immediately contracted upon exposure to moisture, transforming into a soft, stretchable material that could adaptively wrap around nerves and support functional reconstruction. The ongoing advances in stimuli-responsive polymeric films bring new opportunities for addressing the complexities of nerve injuries, offering adaptable and less invasive alternatives that can potentially transform clinical practices in the field of nerve regeneration.
Bone regeneration
Bone defects caused by severe trauma, malignant tumors, and osteoporosis are notoriously challenging to repair due to issues such as infection, large affected areas, and irregular shapes, making these defects a critical clinical concern[156,157]. Such defects are often accompanied by significant damage or loss of the periosteum, which is a connective tissue membrane tightly enveloping the surface of bones. The periosteum plays a vital role in osteogenesis and bone regeneration by providing skeletal fortification, preservation, and reparative capabilities[158]. Film materials have recently gained attention for their potential to replicate the structure and function of the periosteum[159,161]. Stimuli-responsive polymeric films pave a new pathway for promoting bone regeneration through their adaptive variations in structure and properties.
Structural variations
Zhang et al. designed an artificial periosteum with tunable degradation properties, comprising hydroxyapatite (HA) and amphiphilic PELGA [Figure 10A][98]. The film could adjust its stiffness upon hydration and wrap the bone at body temperature due to its excellent shape recovery ability, efficiently delivering skeletal progenitor cells around the target bone and promoting bone regeneration. You et al. employed 3D printing technology to prepare a multi-responsive film with a shape memory polymer (SMP) layer and a hydrogel layer [Figure 10B][25]. The SMP layer had responsive surface microstructures capable of modulating cell behaviors, including proliferation and differentiation, for improving bone regeneration. The hydrogel layer could easily be engineered into a 3D shape matching specific bone structures. Current limitations of biomedical films for bone wrapping and repair include their inability to precisely match the complex 3D curvature and irregular microstructures of the bone surface. For instance, although the bilayer film designed by You et al. could be adaptable to the macroscopical structure via the 3D printing hydrogel layer, achieving this adaptation at the micron scale remains challenging but essential. As a result, these films can effectively wrap the bone, secure the defective site, and release bioactive components for optimal bone regeneration.
Figure 10. Bone regeneration using stimuli-responsive films. (A) Shape memory artificial periosteum with controlled degradation and cell delivery. Copyright 2016, John Wiley and Sons[98]; (B) 3D printing multi-responsive films with a SMP layer and a hydrogel layer for modulating cell behaviors and matching specific structures. Copyright 2021, John Wiley and Sons[25]; (C) An immunomodulatory thermal-responsive composite film for promoting osteogenic cell proliferation and differentiation. Copyright 2022, Frontiers Media S.A.[105]; (D) A sandwich-like PDMS electrical-responsive for improving osteoblast adhesion and proliferation. Copyright 2022, American Chemical Society[107].
Property variations
Smart-responsive polymeric films can promote cell proliferation and differentiation by altering their properties. Zheng et al. reported a thermo-responsive PLLA-based composite film incorporating liquid crystalline phases (LC phases) inside [Figure 10C][105]. This film maintained stable LC phases at body temperature, facilitating materials transport and signal transmission. The results demonstrated that the liquid crystal-modified PLLA film promoted cell proliferation and differentiation through immunomodulation. Huang et al. fabricated a photoacoustic-responsive PLGA-graphene oxide(GO) film that enhanced bone regeneration through photoacoustic stimulation[106]. Qiao et al. designed a sandwich-like PDMS electroactive film, incorporating a SiO2 electret capable of generating continuous electrical stimulation. This stimulation improved the hydrophilicity of the film, promoting osteoblast adhesion and proliferation, thereby facilitating enhanced bone regeneration [Figure 10D][107]. Mechanical compatibility is important to hard tissues[162]. Liu et al. demonstrated that a higher loss modulus of hydrogels enhanced both chondrogenesis and osteogenesis in bone marrow mesenchymal stem cells (BMSCs)[163], which was crucial for bone regeneration. Although the stimuli-responsive polymeric films hold promise as tools for reconstructing the functional properties of the periosteum, further research is needed to develop films that can self-adapt to the structure and mechanical properties of natural bone for advanced and effective bone regeneration.
CONCLUSIONS
Damaged soft and hard tissues remain significant clinical challenges, demanding advanced strategies for effective repair and healing. Stimuli-responsive biomedical polymeric films have emerged as transformative tools in tissue regeneration, offering dynamic adaptability to complex biological environments. This review systematically outlines the fabrication methods, stimuli-responsive mechanisms, and applications for tissue regeneration of these smart materials, highlighting their structural and property variations under diverse stimuli. Among fabrication methods, it is noticed that solvent casting remains the most common and straightforward method for producing thin films, while 3D printing is employed to construct precise structures with high accuracy. More importantly, by categorizing responsive mechanisms into structural (e.g., contraction, bending) and functional (e.g., stiffness modulation, drug release) changes, this work provides a framework for designing films tailored to specific tissue repair needs. Recent applications in skin, cardiovascular, nerve, and bone regeneration demonstrate the potential of these smart films to enhance wound closure, enable minimally invasive implantation, promote cell behaviors, improve interfacial interactions, and so on. However, challenges such as limited deep-tissue penetration, mechanical compatibility with native tissues, and insufficient long-term biocompatibility validation underscore the need for further innovation.
Specifically, various stimuli have their own unique balance of advantages and limitations, necessitating context-specific selection for practical applications. Temperature-based stimuli offer fast response and high operability but risk normal tissue injury, particularly in deep tissues. Light-based methods provide high spatiotemporal resolution and remote controllability, but they are limited by unsatisfactory tissue penetration, reliance on photosensitizers, and potential phototoxicity. Water-triggered methods are mild and highly biocompatible, though they suffer from slow response and dependency on ambient humidity. Electrical stimulation enables precise spatiotemporal control and is minimally invasive; however, implanted electrodes may cause inflammation, and the approach often involves complex, non-portable equipment. Ultrasound stands out for its strong penetration depth and non-invasive nature, yet it can still damage normal tissues and requires expensive devices and technical operations. pH-responsive systems leverage natural targeting (e.g., acidic tumor microenvironments) and eliminate the need for external equipment. However, their effectiveness is hindered by variability in individual/tissue pH levels and challenges in achieving precise local pH control. To achieve suitable responsive functions based on the demands of damaged tissue, researchers or doctors must first consider the features of these stimuli. Additionally, films with structural variations usually generate mechanical force for wrapping, supporting, or fixing; however, mechanical compatibility with specific tissues must be carefully evaluated in vivo to prevent secondary damage. Films with property variations, such as controlled biodegradation and drug release, can adapt to the dynamic microenvironment at defective sites. These advantages make these films highly suitable throughout the entire tissue regeneration process, but their complex components and fabrication methods remain a major limitation. The clinical translation of stimuli-responsive biomedical polymeric films is another critical challenge. Although there are commercial films for skin repair and regeneration, clinical applications of most smart films for deep tissues are still intractable, particularly in achieving scalable manufacturing, regulatory compliance, and long-term biocompatibility.
Future research should prioritize multifunctional integration to address the dynamic demands of tissue regeneration. For example, bone repair is a long-term and comprehensively dynamic healing process, requiring films with minimally invasive capabilities, fixation strength, and controlled bioactivity. Therefore, combining multiple stimuli (e.g., temperature-light-pH) within a single platform to drive systematic internal changes such as critical temperature transitions and reversible chemical reactions could enable spatiotemporal control over material behaviors, particularly for deep-seated defects. Moreover, advancing mechanobiological studies to optimize film-tissue mechanical interactions (e.g., modulus adaptation for cardiovascular implants) will minimize secondary damage. In addition, simplifying fabrication processes while enhancing structural complexity (such as 4D-printed films with programmable shape memory effects) may bridge the gap between laboratory-scale prototypes and clinical scalability. Furthermore, leveraging artificial intelligence (AI)-driven design for predictive material customization and exploring biodegradable systems with eco-friendly components could align these structural and property variations with sustainable medical practices. Ultimately, the convergence of materials science, bioengineering, and clinical insights will unlock the full potential of stimuli-responsive polymeric films in personalized regenerative medicine.
DECLARATIONS
Acknowledgments
The authors would like to thank the authors of the primary studies. The graphic abstract and Figure 1 were drawn using images from Servier Medical Art. Servier Medical Art by Servieris licensed under a Creative Commons Attribution 4.0 Unported License (CC BY) (https://smart.servier.com/citation-sharing/); (https://creativecommons.org/licenses/by/4.0/).
Authors’ contributions
Conceptualization and writing - original draft: Lai, Y.; Zhang, Y.; Xu, Z.; Deng J.
Literature investigation and Figure arrangement: Xu, Z.; Deng, J.; Gao, D.
Writing-review & editing: Lai, Y.; Zhang, Y.; Xu, Z.; Deng, J.; Du, Y.
Supervision and funding acquisition: Lai, Y.; Zhang, Y.
Availability of data and materials
Not applicable.
Financial support and sponsorship
This work was supported by the National Natural Science Foundation of China (52203204, 82427809, and U24A20374), the National Key R&D Program of China, MOST (2023YFC2509900), Shenzhen Medical Research Funds (B2302050, A2403022), the Shenzhen Basic Research General Project (JCYJ20220531100408019), and Shenzhen Science and Technology Program (RCJC20231211090028053, KJZD20230923114612025, JSGG20210629144538010).
Conflicts of interest
Yuxiao Lai is an Associate Editor of the journal Microstructures. Yuxiao Lai was not involved in any steps of the editorial process, including reviewer selection, manuscript handling, and decision making. The other authors declared that there are no conflicts of interest.
Ethical approval and consent to participate
Not applicable.
Consent for publication
Not applicable.
Copyright
© The Author(s) 2025.
REFERENCES
1. Freedman, B. R.; Kuttler, A.; Beckmann, N.; et al. Enhanced tendon healing by a tough hydrogel with an adhesive side and high drug-loading capacity. Nat. Biomed. Eng. 2022, 6, 1167-79.
2. Wang, T.; Wagner, A.; Gehwolf, R.; et al. Load-induced regulation of tendon homeostasis by SPARC, a genetic predisposition factor for tendon and ligament injuries. Sci. Transl. Med. 2021, 13, eabe5738.
3. Cao, S.; Bo, R.; Zhang, Y. Polymeric scaffolds for regeneration of central/peripheral nerves and soft connective tissues. Adv. NanoBiomed. Res. 2023, 3, 2200147.
4. Theocharidis, G.; Yuk, H.; Roh, H.; et al. A strain-programmed patch for the healing of diabetic wounds. Nat. Biomed. Eng. 2022, 6, 1118-33.
5. Hoffman, T.; Antovski, P.; Tebon, P.; et al. Synthetic biology and tissue engineering: toward fabrication of complex and smart cellular constructs. Adv. Funct. Mater. 2020, 30, 1909882.
6. Ghalayani, E. A.; Altomare, L.; Bonetti, L.; et al. Micro-structured patches for dermal regeneration obtained via electrophoretic replica deposition. Appl. Sci. 2020, 10, 5010.
7. Mu, Y.; Gao, W.; Zhou, Y.; Xiao, L.; Xiao, Y. Physiological and pathological/ectopic mineralization: from composition to microstructure. Microstructures. 2023;. , 3, 2023030.
8. Almouemen, N.; Kelly, H. M.; O’Leary, C. Tissue engineering: understanding the role of biomaterials and biophysical forces on cell functionality through computational and structural biotechnology analytical methods. Comput. Struct. Biotechnol. J. 2019, 17, 591-8.
9. Shao, M.; Bigham, A.; Yousefiasl, S.; et al. Recapitulating antioxidant and antibacterial compounds into a package for tissue regeneration: dual function materials with synergistic effect. Small 2023, 19, e2207057.
10. Jia, Q.; Li, Q.; Boucetta, H.; Xu, Z.; Zhang, L. Biomimetic-smart materials for osteochondral regeneration and repair. Microstructures 2024, 4, 2024026.
11. White, E. M.; Yatvin, J.; Grubbs, J. B.; Bilbrey, J. A.; Locklin, J. Advances in smart materials: Stimuli-responsive hydrogel thin films. J. Polym. Sci. B. Polym. Phys. 2013, 51, 1084-99.
12. Moreira, J.; Vale, A. C.; Alves, N. M. Spin-coated freestanding films for biomedical applications. J. Mater. Chem. B. 2021, 9, 3778-99.
13. Zhang, Y.; Li, C.; Guo, A.; et al. Black phosphorus boosts wet-tissue adhesion of composite patches by enhancing water absorption and mechanical properties. Nat. Commun. 2024, 15, 1618.
14. Silvestro, I.; Lopreiato, M.; Scotto, D. A. A.; et al. Hyaluronic acid reduces bacterial fouling and promotes fibroblasts’ adhesion onto chitosan 2D-wound dressings. Int. J. Mol. Sci. 2020, 21, 2070.
15. Draczynski, Z.; Kolesinska, B.; Latanska, I.; Sujka, W. Preparation method of porous dressing materials based on butyric-acetic chitin co-polyesters. Materials. (Basel). 2018, 11, 2359.
16. Gong, M.; Huang, C.; Huang, Y.; et al. Core-sheath micro/nano fiber membrane with antibacterial and osteogenic dual functions as biomimetic artificial periosteum for bone regeneration applications. Nanomedicine 2019, 17, 124-36.
17. Hafezi, F.; Scoutaris, N.; Douroumis, D.; Boateng, J. 3D printed chitosan dressing crosslinked with genipin for potential healing of chronic wounds. Int. J. Pharm. 2019, 560, 406-15.
18. Zhang, Y.; Hu, J.; Xie, R.; et al. A programmable, fast-fixing, osteo-regenerative, biomechanically robust bone screw. Acta. Biomater. 2020, 103, 293-305.
19. Blacklow, S. O.; Li, J.; Freedman, B. R.; Zeidi, M.; Chen, C.; Mooney, D. J. Bioinspired mechanically active adhesive dressings to accelerate wound closure. Sci. Adv. 2019, 5, eaaw3963.
20. Tang, S.; Feng, K.; Yang, R.; et al. Multifunctional adhesive hydrogels: from design to biomedical applications. Adv. Healthc. Mater. 2025, 14, e2403734.
21. Sun, Y.; Tang, Y.; He, Y.; et al. A self-powered wound dressing based on “Lock-ON/OFF” drug release combined electric stimulus therapy for accelerated infected wound healing. Adv. Funct. Mater. 2024, 34, 2315086.
22. Dong, Y.; Zhang, X.; Chen, Y.; Yu, J.; Li, X.; Ding, B. “Stiff-elastic” binary synergistic fibrous tape with thermal-triggered shrinkable and shape recoverable performances for wound closure. Adv. Funct. Mater. 2024, 34, 2402252.
23. Yakubov, S. J.; Wittel, J.; Johnson, G. CRT-700.20 foldax TRIA TAVI: a novel-polymer transcatheter aortic valve: pilot chronic ovine model study. JACC:. Cardiovasc. Interv. 2022, 15, S59-60.
24. Zhang, Y.; Zheng, N.; Cao, Y.; et al. Climbing-inspired twining electrodes using shape memory for peripheral nerve stimulation and recording. Sci. Adv. 2019, 5, eaaw1066.
25. You, D.; Chen, G.; Liu, C.; et al. 4D Printing of multi-responsive membrane for accelerated in vivo bone healing via remote regulation of stem cell fate. Adv. Funct. Mater. 2021, 31, 2103920.
26. Zhang, Y.; Hu, J.; Zhu, S.; Qin, T.; Ji, F. A “trampoline” nanocomposite: tuning the interlayer spacing in graphene oxide/polyurethane to achieve coalesced mechanical and memory properties. Compos. Sci. Technol. 2019, 180, 14-22.
27. Lou, D.; Sun, Y.; Li, J.; et al. Double lock label based on thermosensitive polymer hydrogels for information camouflage and multilevel encryption. Angew. Chem. Int. Ed. Engl. 2022, 61, e202117066.
28. Zhang, Y.; Li, C.; Zhang, W.; et al. 3D-printed NIR-responsive shape memory polyurethane/magnesium scaffolds with tight-contact for robust bone regeneration. Bioact. Mater. 2022, 16, 218-31.
29. Cheng, S. Q.; Zhang, S. Y.; Min, X. H.; et al. Photoresponsive solid nanochannels membranes: design and applications. Small 2022, 18, e2105019.
30. Yi, J.; Zou, G.; Huang, J.; et al. Water-responsive supercontractile polymer films for bioelectronic interfaces. Nature 2023, 624, 295-302.
31. Dong, C.; Carnicer-Lombarte, A.; Bonafè, F.; et al. Electrochemically actuated microelectrodes for minimally invasive peripheral nerve interfaces. Nat. Mater. 2024, 23, 969-76.
32. Sadeghi-Goughari, M.; Jeon, S.; Kwon, H. J. Magnetic nanoparticles-enhanced focused ultrasound heating: size effect, mechanism, and performance analysis. Nanotechnology 2020, 31, 245101.
33. Fattah-alhosseini, A.; Chaharmahali, R.; Alizad, S.; Kaseem, M.; Dikici, B. A review of smart polymeric materials: recent developments and prospects for medicine applications. Hybrid. Advances. 2024, 5, 100178.
34. Park, D.; Kim, J. W.; Shin, K.; Kim, J. W. Bacterial cellulose nanofibrils-reinforced composite hydrogels for mechanical compression-responsive on-demand drug release. Carbohydr. Polym. 2021, 272, 118459.
35. Xia, Y.; He, Y.; Zhang, F.; Liu, Y.; Leng, J. A review of shape memory polymers and composites: mechanisms, materials, and applications. Adv. Mater. 2021, 33, e2000713.
37. Zhao, Q.; Wang, J.; Cui, H.; Chen, H.; Wang, Y.; Du, X. Programmed shape-morphing scaffolds enabling facile 3D endothelialization. Adv. Funct. Mater. 2018, 28, 1801027.
38. Yang, F. K.; Cholewinski, A.; Yu, L.; Rivers, G.; Zhao, B. A hybrid material that reversibly switches between two stable solid states. Nat. Mater. 2019, 18, 874-82.
39. Luo, L.; Zhang, F.; Wang, L.; Liu, Y.; Leng, J. Recent Advances in shape memory polymers: multifunctional materials, multiscale structures, and applications. Adv. Funct. Materials. 2024, 34, 2312036.
41. Vukomanović, M.; Gazvoda, L.; Kurtjak, M.; et al. Filler-enhanced piezoelectricity of poly-l-lactide and its use as a functional ultrasound-activated biomaterial. Small 2023, 19, e2301981.
42. Cheng, Y.; Xu, Y.; Qian, Y.; Chen, X.; Ouyang, Y.; Yuan, W. 3D structured self-powered PVDF/PCL scaffolds for peripheral nerve regeneration. Nano. Energy. 2020, 69, 104411.
43. Niu, W.; Tian, Q.; Liu, Z.; Liu, X. Solvent-free and skin-like supramolecular ion-conductive elastomers with versatile processability for multifunctional ionic tattoos and on-skin bioelectronics. Adv. Mater. 2023, 35, e2304157.
44. Li, M.; Chen, J.; Shi, M.; Zhang, H.; Ma, P. X.; Guo, B. Electroactive anti-oxidant polyurethane elastomers with shape memory property as non-adherent wound dressing to enhance wound healing. Chem. Eng. J. 2019, 375, 121999.
45. Yi, J.; Ren, X.; Li, Y.; et al. Rapid-response water-shrink films with high output work density based on polyethylene oxide and α-cyclodextrin for autonomous wound closure. Adv. Mater. 2024, 36, e2403551.
46. Ge, W.; Zhang, F.; Wang, D.; et al. Highly tough, stretchable, and solvent-resistant cellulose nanocrystal photonic films for mechanochromism and actuator properties. Small 2022, 18, e2107105.
47. Sun, M.; Elkhodiry, M.; Shi, L.; et al. A biomimetic multilayered polymeric material designed for heart valve repair and replacement. Biomaterials 2022, 288, 121756.
48. Zhang, X.; Li, P.; Zeng, J.; et al. Acetylated cellulose nanofibers enhanced bio-based polyesters derived from 10-undecanoic acid toward recyclable and degradable plastics. Chem. Eng. J. 2024, 479, 147797.
49. Seong, D.; Choi, Y.; Choi, I. C.; et al. Sticky and strain-gradient artificial epineurium for sutureless nerve repair in rodents and nonhuman primates. Adv. Mater. 2024, 36, e2307810.
50. Jin, B.; Liu, J.; Shi, Y.; Chen, G.; Zhao, Q.; Yang, S. Solvent-assisted 4D programming and reprogramming of liquid crystalline organogels. Adv. Mater. 2022, 34, e2107855.
51. Palo, M.; Rönkönharju, S.; Tiirik, K.; Viidik, L.; Sandler, N.; Kogermann, K. Bi-layered polymer carriers with surface modification by electrospinning for potential wound care applications. Pharmaceutics 2019, 11, 678.
52. Dai, X.; Heng, B. C.; Bai, Y.; et al. Restoration of electrical microenvironment enhances bone regeneration under diabetic conditions by modulating macrophage polarization. Bioact. Mater. 2021, 6, 2029-38.
53. Emadi, H.; Karevan, M.; Masoudi, R. M.; et al. Bioactive and biodegradable polycaprolactone-based nanocomposite for bone repair applications. Polymers. (Basel). 2023, 15, 3617.
54. Ribeiro, S.; Ribeiro, C.; Carvalho, E. O.; et al. Magnetically activated electroactive microenvironments for skeletal muscle tissue regeneration. ACS. Appl. Bio. Mater. 2020, 3, 4239-52.
55. Di, M.; Sun, X.; Hu, L.; et al. Hollow COF selective layer based flexible composite membranes constructed by an integrated “casting-precipitation-evaporation” strategy. Adv. Funct. Mater. 2022, 32, 2111594.
56. Sun, L.; Che, L.; Li, M.; et al. Reinforced nacre-like MXene/Sodium alginate composite films for bioinspired actuators driven by moisture and sunlight. Small 2024, 20, e2406832.
57. Silva, J. M.; Vilela, C.; Girão, A. V.; et al. Wood inspired biobased nanocomposite films composed of xylans, lignosulfonates and cellulose nanofibers for active food packaging. Carbohydr. Polym. 2024, 337, 122112.
58. Lv, S.; Yuan, X.; Xiao, J.; Jiang, X. Hemostasis-osteogenesis integrated Janus carboxymethyl chitin/hydroxyapatite porous membrane for bone defect repair. Carbohydr. Polym. 2023, 313, 120888.
59. Savencu, I.; Iurian, S.; Porfire, A.; Bogdan, C.; Tomuță, I. Review of advances in polymeric wound dressing films. React. Funct. Polym. 2021, 168, 105059.
60. Nasri-Nasrabadi, B.; Mehrasa, M.; Rafienia, M.; Bonakdar, S.; Behzad, T.; Gavanji, S. Porous starch/cellulose nanofibers composite prepared by salt leaching technique for tissue engineering. Carbohydr. Polym. 2014, 108, 232-8.
61. Aramwit, P.; Ratanavaraporn, J.; Ekgasit, S.; Tongsakul, D.; Bang, N. A green salt-leaching technique to produce sericin/PVA/glycerin scaffolds with distinguished characteristics for wound-dressing applications. J. Biomed. Mater. Res. B. Appl. Biomater. 2015, 103, 915-24.
62. Poonguzhali, R.; Khaleel, B. S.; Sugantha, K. V. Fabrication of asymmetric nanostarch reinforced Chitosan/PVP membrane and its evaluation as an antibacterial patch for in vivo wound healing application. Int. J. Biol. Macromol. 2018, 114, 204-13.
63. Liang, X.; Qi, Y.; Pan, Z.; et al. Design and preparation of quasi-spherical salt particles as water-soluble porogens to fabricate hydrophobic porous scaffolds for tissue engineering and tissue regeneration. Mater. Chem. Front. 2018, 2, 1539-53.
64. Kelso, M. V.; Mahenderkar, N. K.; Chen, Q.; Tubbesing, J. Z.; Switzer, J. A. Spin coating epitaxial films. Science 2019, 364, 166-9.
65. Fujie, T. Development of free-standing polymer nanosheets for advanced medical and health-care applications. Polym. J. 2016, 48, 773-80.
66. Chiarelli, P. A.; Johal, M. S.; Holmes, D. J.; Casson, J. L.; Robinson, J. M.; Wang, H. Polyelectrolyte spin-assembly. Langmuir 2002, 18, 168-73.
68. Nishiyama, T.; Sumihara, T.; Sato, E.; Horibe, H. Effect of solvents on the crystal formation of poly(vinylidene fluoride) film prepared by a spin-coating process. Polym. J. 2017, 49, 319-25.
69. Okamura, Y.; Kabata, K.; Kinoshita, M.; et al. Fragmentation of poly(lactic acid) nanosheets and patchwork treatment for burn wounds. Adv. Mater. 2013, 25, 545-51.
70. Shi, X.; Fujie, T.; Saito, A.; et al. Periosteum-mimetic structures made from freestanding microgrooved nanosheets. Adv. Mater. 2014, 26, 3290-6.
71. Fujie, T.; Saito, A.; Kinoshita, M.; et al. Dual therapeutic action of antibiotic-loaded nanosheets for the treatment of gastrointestinal tissue defects. Biomaterials 2010, 31, 6269-78.
72. Puiggalí-Jou, A.; Pérez-Madrigal, M. M.; Del, V. L. J.; et al. Confinement of a β-barrel protein in nanoperforated free-standing nanomembranes for ion transport. Nanoscale 2016, 8, 16922-35.
73. Fujie, T.; Matsutani, N.; Kinoshita, M.; Okamura, Y.; Saito, A.; Takeoka, S. Adhesive, flexible, and robust polysaccharide nanosheets integrated for tissue-defect repair. Adv. Funct. Mater. 2009, 19, 2560-8.
74. Zhao, G.; Zhang, X.; Lu, T. J.; Xu, F. Recent Advances in electrospun nanofibrous scaffolds for cardiac tissue engineering. Adv. Funct. Mater. 2015, 25, 5726-38.
75. Wang, L.; Huang, T.; Xu, X.; et al. Robust dual equivariant gradient antibacterial wound dressing-loaded artificial skin with nano-chitin particles via an electrospinning-reactive strategy. Adv. Fiber. Mater. 2025, 7, 204-18.
76. Qin, M.; Ma, J.; Wu, B.; Li, K.; Yi, X. Microstructure and hydrophobicity of PVDF-based films prepared by electrospinning technique. J. Phys. Chem. C. 2024, 128, 3609-15.
77. Mishra, R. K.; Mishra, P.; Verma, K.; et al. Electrospinning production of nanofibrous membranes. Environ. Chem. Lett. 2019, 17, 767-800.
78. Rahmati, M.; Mills, D. K.; Urbanska, A. M.; et al. Electrospinning for tissue engineering applications. Prog. Mater. Sci. 2021, 117, 100721.
79. Liu, B.; Li, H.; Meng, F.; et al. 4D printed hydrogel scaffold with swelling-stiffening properties and programmable deformation for minimally invasive implantation. Nat. Commun. 2024, 15, 1587.
80. Yang, Y.; Yao, Z.; Sun, Y.; et al. 3D-printed manganese dioxide incorporated scaffold promotes osteogenic-angiogenic coupling for refractory bone defect by remodeling osteo-regenerative microenvironment. Bioact. Mater. 2025, 44, 354-70.
81. Jeong, S.; Jo, M.; Ahn, H. 3D-printed film architecture via automatic micro 3D-printing system: Micro-intersection engineering of V2O5 thin/thick films for ultrafast electrochromic energy storage devices. Chem. Eng. J. 2023, 475, 146503.
82. Shin, C. S.; Cabrera, F. J.; Lee, R.; et al. 3D-bioprinted inflammation modulating polymer scaffolds for soft tissue repair. Adv. Mater. 2021, 33, e2003778.
83. Zhang, W.; Wang, H.; Wang, H.; et al. Structural multi-colour invisible inks with submicron 4D printing of shape memory polymers. Nat. Commun. 2021, 12, 112.
84. Ren, L.; Li, Z.; Liu, Q.; et al. Programmable 4D printing of bioinspired solvent-driven morphing composites. Adv. Mater. Technol. 2021, 6, 2001289.
85. Almotairy, A.; Alyahya, M.; Althobaiti, A.; et al. Disulfiram 3D printed film produced via hot-melt extrusion techniques as a potential anticervical cancer candidate. Int. J. Pharm. 2023, 635, 122709.
86. Wang, P.; Sun, Y.; Li, D.; et al. Extrusion-based 3D co-printing: Printing material design and novel workflow for fabricating patterned heterogeneous tissue structures. Materials. &. Design. 2023, 227, 111737.
87. Andriotis, E. G.; Eleftheriadis, G. K.; Karavasili, C.; Fatouros, D. G. Development of bio-active patches based on pectin for the treatment of ulcers and wounds using 3D-bioprinting technology. Pharmaceutics 2020, 12, 56.
88. Wang, Q.; Zhang, Y.; Shao, F.; et al. Bio-inspired design of 4D‐printed scaffolds capable of programmable multi-step transformations toward vascular reconstruction. Adv. Funct. Mater. 2024, 34, 2407592.
89. Huang, T.; Sun, Z.; Heath, D. E.; O’Brien-Simpson, N.; O’Connor, A. J. 3D printed and smart alginate wound dressings with pH-responsive drug and nanoparticle release. Chem. Eng. J. 2024, 492, 152117.
90. Ma, Y.; Hua, M.; Wu, S.; et al. Bioinspired high-power-density strong contractile hydrogel by programmable elastic recoil. Sci. Adv. 2020, 6, eabd2520.
91. Ryplida, B.; Lee, K. D.; In, I.; Park, S. Y. Light-induced swelling‐responsive conductive, adhesive, and stretchable wireless film hydrogel as electronic artificial skin. Adv. Funct. Mater. 2019, 29, 1903209.
92. Li, S.; Zhang, H.; Xie, J.; et al. In vivo self-assembled shape-memory polyurethane for minimally invasive delivery and therapy. Mater. Horiz. 2023, 10, 3438-49.
93. Montgomery, M.; Ahadian, S.; Davenport, H. L.; et al. Flexible shape-memory scaffold for minimally invasive delivery of functional tissues. Nat. Mater. 2017, 16, 1038-46.
94. Wang, J.; Xiong, H.; Zhu, T.; et al. Bioinspired multichannel nerve guidance conduit based on shape memory nanofibers for potential application in peripheral nerve repair. ACS. Nano. 2020, 14, 12579-95.
95. Cui, Y.; Li, L.; Liu, C.; et al. Water-responsive 3D electronics for smart biological interfaces. Nano. Lett. 2023, 23, 11693-701.
96. Cremonini, A.; Sol, J. A. H. P.; Schenning, A. P. H. J.; Masiero, S.; Debije, M. G. The interplay between different stimuli in a 4d printed photo-, thermal-, and water-responsive liquid crystal elastomer actuator. Chem. -. Eur. J. 2023, 29, e202300648.
97. Zhang, M.; An, H.; Gu, Z.; et al. Mimosa-inspired stimuli-responsive curling bioadhesive tape promotes peripheral nerve regeneration. Adv. Mater. 2023, 35, e2212015.
98. Zhang, B.; Filion, T. M.; Kutikov, A. B.; Song, J. Facile Stem cell delivery to bone grafts enabled by smart shape recovery and stiffening of degradable synthetic periosteal membranes. Adv. Funct. Mater. 2017, 27, 1604784.
99. Lanzalaco, S.; Turon, P.; Weis, C.; et al. Toward the new generation of surgical meshes with 4D response: soft, dynamic, and adaptable. Adv. Funct. Mater. 2020, 30, 2004145.
100. Paula, C. T.; Madeira, A. B.; Pereira, P.; et al. ROS-degradable PEG-based wound dressing films with drug release and antibacterial properties. Eur. Polym. J. 2022, 177, 111447.
101. Zha, K.; Xiong, Y.; Zhang, W.; et al. Waste to Wealth: Near-infrared/pH dual-responsive copper-humic acid hydrogel films for bacteria-infected cutaneous wound healing. ACS. Nano. 2023, 17, 17199-216.
102. Liu, Y.; Wang, L.; Liu, Z.; et al. Durable Immunomodulatory nanofiber niche for the functional remodeling of cardiovascular tissue. ACS. Nano. 2024, 18, 951-71.
103. Huang, K.; Li, X.; Chen, W.; et al. Flexible intelligent array patch based on synergy of polyurethane and nanofiber for sensitive monitor and smart treatment. Chem. Eng. J. 2022, 443, 136378.
104. Song, Q.; Wang, D.; Li, H.; et al. Dual-response of multi-functional microsphere system to ultrasound and microenvironment for enhanced bone defect treatment. Bioact. Mater. 2024, 32, 304-18.
105. Zheng, Z.; Wang, R.; Lin, J.; et al. Liquid crystal modified polylactic acid improves cytocompatibility and M2 polarization of macrophages to promote osteogenesis. Front. Bioeng. Biotechnol. 2022, 10, 887970.
106. Huang, Z.; Xu, J.; Chen, J.; et al. Photoacoustic stimulation promotes the osteogenic differentiation of bone mesenchymal stem cells to enhance the repair of bone defect. Sci. Rep. 2017, 7, 15842.
107. Qiao, Z.; Lian, M.; Liu, X.; et al. Electreted sandwich membranes with persistent electrical stimulation for enhanced bone regeneration. ACS. Appl. Mater. Interfaces. 2022, 14, 31655-66.
108. Yarger, J. L.; Cherry, B. R.; van, V. A. Uncovering the structure-function relationship in spider silk. Nat. Rev. Mater. 2018, 3, BFnatrevmats20188.
109. Yang, Y.; Li, C.; Palmer, L. C.; Stupp, S. I. Autonomous hydrogel locomotion regulated by light and electric fields. Sci. Adv. 2023, 9, eadi4566.
110. Li, C.; Iscen, A.; Palmer, L. C.; Schatz, G. C.; Stupp, S. I. Light-driven expansion of spiropyran hydrogels. J. Am. Chem. Soc. 2020, 142, 8447-53.
111. Li, C.; Xue, Y.; Han, M.; et al. Synergistic photoactuation of bilayered spiropyran hydrogels for predictable origami-like shape change. Matter 2021, 4, 1377-90.
112. Guo, K.; Yang, X.; Zhou, C.; Li, C. Self-regulated reversal deformation and locomotion of structurally homogenous hydrogels subjected to constant light illumination. Nat. Commun. 2024, 15, 1694.
113. Zhou, H.; Tang, D.; Kang, X.; et al. Degradable pseudo conjugated polymer nanoparticles with nir-ii photothermal effect and cationic quaternary phosphonium structural bacteriostasis for anti-infection therapy. Adv. Sci. (Weinh). 2022, 9, e2200732.
114. Zheng, B. D.; Xiao, M. T. Polysaccharide-based hydrogel with photothermal effect for accelerating wound healing. Carbohydr. Polym. 2023, 299, 120228.
115. Zeng, F.; Tang, L.; Zhang, Q.; et al. Coordinating the mechanisms of action of ferroptosis and the photothermal effect for cancer theranostics. Angew. Chem. 2022, 134, e202112925.
116. Aldalawi, A. A.; Suardi, N.; Ahmed, N. M.; et al. Comparison of wavelength-dependent penetration depth of 532 nm and 660 nm lasers in different tissue types. J. Lasers. Med. Sci. 2023, 14, e28.
117. Finlayson, L.; Barnard, I. R. M.; McMillan, L.; et al. Depth penetration of light into skin as a function of wavelength from 200 to 1000 nm. Photochem. Photobiol. 2022, 98, 974-81.
118. Zhang, Y.; Hu, J.; Zhao, X.; Xie, R.; Qin, T.; Ji, F. Mechanically robust shape memory polyurethane nanocomposites for minimally invasive bone repair. ACS. Appl. Bio. Mater. 2019, 2, 1056-65.
119. Clerc, M.; Sandlass, S.; Rifaie-Graham, O.; et al. Visible light-responsive materials: the (photo)chemistry and applications of donor-acceptor Stenhouse adducts in polymer science. Chem. Soc. Rev. 2023, 52, 8245-94.
120. Kuang, X.; Rong, Q.; Belal, S.; et al. Self-enhancing sono-inks enable deep-penetration acoustic volumetric printing. Science 2023, 382, 1148-55.
121. Elias, W. J.; Lipsman, N.; Ondo, W. G.; et al. A randomized trial of focused ultrasound thalamotomy for essential tremor. N. Engl. J. Med. 2016, 375, 730-9.
122. Zhou, Y.; Ji, X.; Niu, J.; et al. Ultrasound-guided high-intensity focused ultrasound for devascularization of uterine fibroid: a feasibility study. Ultrasound. Med. Biol. 2021, 47, 2622-35.
123. Zhu, Y.; Deng, K.; Zhou, J.; et al. Shape-recovery of implanted shape-memory devices remotely triggered via image-guided ultrasound heating. Nat. Commun. 2024, 15, 1123.
124. Choi, J. H.; Lee, J. S.; Yang, D. H.; et al. Development of a temperature-responsive hydrogel incorporating PVA into NIPAAm for controllable drug release in skin regeneration. ACS. Omega. 2023, 8, 44076-85.
125. Liu, W.; Wang, A.; Yang, R.; et al. Water-triggered stiffening of shape-memory polyurethanes composed of hard backbone dangling PEG soft segments. Adv. Mater. 2022, 34, e2201914.
126. Hu, H.; Wang, H.; Yang, Y.; Xu, J. F.; Zhang, X. A bacteria-responsive porphyrin for adaptable photodynamic/photothermal therapy. Angew. Chem. Int. Ed. Engl. 2022, 61, e202200799.
127. Weiser, J. R.; Saltzman, W. M. Controlled release for local delivery of drugs: barriers and models. J. Control. Release. 2014, 190, 664-73.
128. Cheah, E.; Bansal, M.; Nguyen, L.; et al. Electrically responsive release of proteins from conducting polymer hydrogels. Acta. Biomater. 2023, 158, 87-100.
129. Boehler, C.; Oberueber, F.; Asplund, M. Tuning drug delivery from conducting polymer films for accurately controlled release of charged molecules. J. Control. Release. 2019, 304, 173-80.
130. Chikar, J. A.; Hendricks, J. L.; Richardson-Burns, S. M.; Raphael, Y.; Pfingst, B. E.; Martin, D. C. The use of a dual PEDOT and RGD-functionalized alginate hydrogel coating to provide sustained drug delivery and improved cochlear implant function. Biomaterials 2012, 33, 1982-90.
131. Yun, S. H.; Kwok, S. J. J. Light in diagnosis, therapy and surgery. Nat. Biomed. Eng. 2017, 1, 0008.
132. Huo, S.; Zhao, P.; Shi, Z.; et al. Mechanochemical bond scission for the activation of drugs. Nat. Chem. 2021, 13, 131-9.
133. Mu, Q.; Cui, K.; Wang, Z. J.; et al. Force-triggered rapid microstructure growth on hydrogel surface for on-demand functions. Nat. Commun. 2022, 13, 6213.
134. Zhao, Q.; Wang, J.; Wang, Y.; Cui, H.; Du, X. A stage-specific cell-manipulation platform for inducing endothelialization on demand. Natl. Sci. Rev. 2020, 7, 629-43.
135. Hu, M.; Guo, J.; Du, J.; et al. Development of Ca2+-based, ion-responsive superabsorbent hydrogel for cement applications: self-healing and compressive strength. J. Colloid. Interface. Sci. 2019, 538, 397-403.
136. Nagase, K.; Yamato, M.; Kanazawa, H.; Okano, T. Poly(N-isopropylacrylamide)-based thermoresponsive surfaces provide new types of biomedical applications. Biomaterials 2018, 153, 27-48.
137. Harris-Tryon, T. A.; Grice, E. A. Microbiota and maintenance of skin barrier function. Science 2022, 376, 940-5.
138. Rastogi, A.; Goyal, G.; Kesavan, R.; et al. Long term outcomes after incident diabetic foot ulcer: multicenter large cohort prospective study (EDI-FOCUS investigators) epidemiology of diabetic foot complications study: epidemiology of diabetic foot complications study. Diabetes. Res. Clin. Pract. 2020, 162, 108113.
139. Zhang, P.; Lu, J.; Jing, Y.; Tang, S.; Zhu, D.; Bi, Y. Global epidemiology of diabetic foot ulceration: a systematic review and meta-analysis†. Ann. Med. 2017, 49, 106-16.
140. Chen, L.; Sun, S.; Gao, Y.; Ran, X. Global mortality of diabetic foot ulcer: a systematic review and meta-analysis of observational studies. Diabetes. Obes. Metab. 2023, 25, 36-45.
141. Liang, W.; Lu, Q.; Yu, F.; et al. A multifunctional green antibacterial rapid hemostasis composite wound dressing for wound healing. Biomater. Sci. 2021, 9, 7124-33.
142. Pandit, A. P.; Koyate, K. R.; Kedar, A. S.; Mute, V. M. Spongy wound dressing of pectin/carboxymethyl tamarind seed polysaccharide loaded with moxifloxacin beads for effective wound heal. Int. J. Biol. Macromol. 2019, 140, 1106-15.
143. Lazarus, E.; Barnum, L.; Ramesh, S.; et al. Engineering tools for stimulating wound healing. Appl. Phys. Rev. 2024, 11, 021304.
144. Moeinipour, A.; Afkhami, A.; Madrakian, T. Stimuli-responsive polymeric film based on hydrogen-bonded organic framework designing as a smart wound dressing. Iran. Polym. J. . DOI: 10.1007/s13726-024-01443-1.
145. Veves, A.; Sheehan, P.; Pham, H. T. A randomized, controlled trial of promogran (a collagen/oxidized regenerated cellulose dressing) vs standard treatment in the management of diabetic foot ulcers. Arch. Surg. 2002, 137, 822-7.
146. Lin, Y. H.; Hsu, W. S.; Chung, W. Y.; Ko, T. H.; Lin, J. H. Silver-based wound dressings reduce bacterial burden and promote wound healing. Int. Wound. J. 2016, 13, 505-11.
147. Libby, P.; Bornfeldt, K. E.; Tall, A. R. Atherosclerosis: successes, surprises, and future challenges. Circ. Res. 2016, 118, 531-4.
148. Liu, Y.; Li, C.; Yang, X.; Yang, B.; Fu, Q. Stimuli-responsive polymer-based nanosystems for cardiovascular disease theranostics. Biomater. Sci. 2024, 12, 3805-25.
149. Hu, N.; Shi, J. X.; Chen, C.; et al. Constructing organoid-brain-computer interfaces for neurofunctional repair after brain injury. Nat. Commun. 2024, 15, 9580.
150. Chen, Y.; Long, X.; Lin, W.; et al. Bioactive 3D porous cobalt-doped alginate/waterborne polyurethane scaffolds with a coral reef-like rough surface for nerve tissue engineering application. J. Mater. Chem. B. 2021, 9, 322-35.
151. Khaing, Z. Z.; Schmidt, C. E. Advances in natural biomaterials for nerve tissue repair. Neurosci. Lett. 2012, 519, 103-14.
152. Chang, W.; Shah, M. B.; Lee, P.; Yu, X. Tissue-engineered spiral nerve guidance conduit for peripheral nerve regeneration. Acta. Biomater. 2018, 73, 302-11.
153. Shan, Y.; Xu, L.; Cui, X.; et al. A responsive cascade drug delivery scaffold adapted to the therapeutic time window for peripheral nerve injury repair. Mater. Horiz. 2024, 11, 1032-45.
154. Darnell, M.; O’Neil, A.; Mao, A.; Gu, L.; Rubin, L. L.; Mooney, D. J. Material microenvironmental properties couple to induce distinct transcriptional programs in mammalian stem cells. Proc. Natl. Acad. Sci. U. S. A. 2018, 115, E8368-77.
155. Roth, J. G.; Huang, M. S.; Navarro, R. S.; Akram, J. T.; LeSavage, B. L.; Heilshorn, S. C. Tunable hydrogel viscoelasticity modulates human neural maturation. Sci. Adv. 2023, 9, eadh8313.
156. Wang, T.; Zhai, Y.; Nuzzo, M.; Yang, X.; Yang, Y.; Zhang, X. Layer-by-layer nanofiber-enabled engineering of biomimetic periosteum for bone repair and reconstruction. Biomaterials 2018, 182, 279-88.
157. Wei, H.; Cui, J.; Lin, K.; Xie, J.; Wang, X. Recent advances in smart stimuli-responsive biomaterials for bone therapeutics and regeneration. Bone. Res. 2022, 10, 17.
158. Carmeliet, P.; Tessier-Lavigne, M. Common mechanisms of nerve and blood vessel wiring. Nature 2005, 436, 193-200.
159. Roberts, S. J.; van, G. N.; Carmeliet, G.; Luyten, F. P. Uncovering the periosteum for skeletal regeneration: the stem cell that lies beneath. Bone 2015, 70, 10-8.
160. Li, Q.; Liu, W.; Hou, W.; et al. Micropatterned photothermal double-layer periosteum with angiogenesis-neurogenesis coupling effect for bone regeneration. Mater. Today. Bio. 2023, 18, 100536.
161. Yang, Y.; Rao, J.; Liu, H.; et al. Biomimicking design of artificial periosteum for promoting bone healing. J. Orthop. Translat. 2022, 36, 18-32.
162. Isomursu, A.; Park, K. Y.; Hou, J.; et al. Directed cell migration towards softer environments. Nat. Mater. 2022, 21, 1081-90.
Cite This Article
How to Cite
Download Citation
Export Citation File:
Type of Import
Tips on Downloading Citation
Citation Manager File Format
Type of Import
Direct Import: When the Direct Import option is selected (the default state), a dialogue box will give you the option to Save or Open the downloaded citation data. Choosing Open will either launch your citation manager or give you a choice of applications with which to use the metadata. The Save option saves the file locally for later use.
Indirect Import: When the Indirect Import option is selected, the metadata is displayed and may be copied and pasted as needed.
About This Article
Copyright
Data & Comments
Data
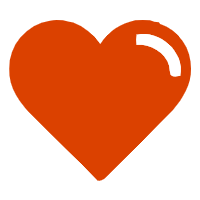
Comments
Comments must be written in English. Spam, offensive content, impersonation, and private information will not be permitted. If any comment is reported and identified as inappropriate content by OAE staff, the comment will be removed without notice. If you have any queries or need any help, please contact us at [email protected].