Towards the optimal design of optically clear adhesives for flexible display
Abstract
As device form factors evolve towards increased complexity and flexibility, the role of adhesives within the display module stack becomes increasingly crucial. These adhesives are essential for bonding functional layers with minimal thickness while mitigating stress during the dynamic behavior of flexible devices. This paper offers a comprehensive overview of the essential properties of adhesives - such as adhesion, viscoelasticity, optical characteristics, and environmental reliability - necessary for the stable operation of flexible display devices across diverse form factors and environments. In particular, it provides an in-depth look at ongoing research in simulation, material selection, polymer network control, and the integration of new functionalities to achieve optimal performance. Furthermore, this paper discusses extensive research outcomes addressing the growing demand for sustainable solutions. Building on this knowledge, we highlight the future direction of adhesives for flexible displays.
Keywords
INTRODUCTION
The advancement of information technology has transformed information exchange, enabling seamless communication with realistic content, unrestricted by time or space[1,2]. This shift has led users to favor visual content, such as videos and images, for more accurate information transmission compared to text. As a result, there is a rising demand for portable yet large displays, which has spurred the development of foldable smartphones and laptops [Figure 1A]. Additionally, the growing interest in optimizing space and enhancing interior design has driven the creation of rollable TVs, with research focused on reducing the curvature radius and improving the reliability of these displays. In healthcare, the need for personalized solutions has increased interest in realistic displays, such as feelable or implantable ones, used in medical sensors. Consequently, extensive research is being conducted on flexible, large-form-factor displays to meet diverse application needs[3-7] [Figure 1B].
Figure 1. (A) The development of IT and smart devices; (B) The changes of device form factor. Foldable and rollable devices are designed to deform primarily in 2-dimensions along a single axis. Stretchable devices are engineered to deform in 3-dimensions with more complex geometries. IT: Information technology.
Adhesives, particularly pressure-sensitive adhesives (PSAs), are widely used in various fields ranging from tapes and labels to electronics, automotive, and sealing because they can easily attach to numerous solid surfaces with light pressure[8,9]. Among them, acrylic PSAs lead the market due to their resistance to ultraviolet (UV) light, humidity, and temperature, as well as their excellent optical properties and cost-effectiveness, showing a steady growth in market share[10]. Specifically, optically clear adhesives (OCAs), a type of acrylic PSA, play a vital role in the diverse designs of modern displays. In traditional rigid displays, it is crucial to use OCAs that ensure strong adhesion for each functional layer, maintain optical transparency, preserve organic light-emitting diode (OLED) light brightness, and offer high reliability under diverse environmental conditions. With the increasing demand for space-saving and ubiquitous communication, the newly released foldable phone features additional integrated layers made of thinner, more flexible materials compared to existing rigid phones, necessitating the use of more adhesives [Figure 2A, right]. As these functional layers become thinner, they exhibit lower bending stiffness, which increases their flexibility[11-15] [Figure 2B]. Consequently, the layers used in flexible displays are composed of thin and flexible materials[16-23]. With the transition to flexible displays, the role of adhesives has become more significant. These adhesives are crucial because they not only bond adjacent layers but also mitigate stress caused by deformation[24-26]. They must accommodate dynamic movement while maintaining optical clarity and color integrity. Depending on the application and curvature radius of the flexible display, the required properties of the adhesive can vary. Therefore, the development of flexible adhesives must consider mechanical properties, adhesion properties, optical properties, and environmental stability.
Figure 2. (A) The module stack structure of OLED devices. The rigid or curved phone (left) and foldable phone (right) structures are different. The nomenclatures are generalized for each layer; (B) The bending stiffness of bending structures relates to the thickness and modulus of each layer that constitutes the device as depicted[15]. Reproduced with permission. Copyright © 2020 The Society for Information Display; (C) The failure mode of display modules occurs when they are bent. The occurrence of failure is related to the modulus of adhesives. If the shear modulus of adhesives is extremely low, the stress throughout the device is dissipated due to the existence of multiple neutral planes; (D) The requirements of environmental reliability according to various applications. OLED: Organic light-emitting diode.
As devices undergo structural and functional advancements, high-tech flexible adhesives must further enhance their already well-defined properties. Future adhesives need to retain their current properties while being manufactured thinner or maintaining stable performance across a broader temperature range. With the advancement of display technology, new functionalities such as UV blocking, stimuli responsiveness, and shock resistance are being added[27-29]. Additionally, to enhance eco-friendliness from an environmental, social, and governance (ESG) perspective, research on sustainable adhesives throughout all stages of production, use, and processing is essential.
In this paper, we explore the development of adhesives for flexible displays as influenced by changes in display form factors. We identify the key parameters required for these adhesives and, based on current research, consider how they should evolve as display form factors, functionality, and usability become more sophisticated. Additionally, we review the current status of eco-friendly adhesives and offer insights into future directions for developing flexible adhesives that meet both sustainability and functionality requirements.
THE DEVELOPMENT OF ADHESIVES FOR FLEXIBLE DEVICES
The advent of flexible display devices has necessitated the development of new technologies across various fields to ensure their sophisticated and stable operation. OLED-based devices, in particular, have been widely adopted and studied due to their inherent flexibility. To improve adoption in wearable sensors and smart textiles, alternative display technologies such as mechanoluminescence[30-32] and thermoluminescence[33] are recently being developed, moving beyond traditional electroluminescent OLEDs. Research has expanded not only on the emissive layers of displays but also on the surrounding components. Notably, adhesives play a crucial role in enhancing the mechanical durability of these devices. Flexible adhesives have been developed to effectively mitigate various forms of stress (e.g., tensile, compressive, and shear) acting on devices, thereby preventing defects such as cracking, delamination, and buckling, and ensuring the mechanical stability of display modules[34]. To address these challenges, the neutral plane splitting strategy, which is a promising technology for foldable devices, has been proposed[24,35-37]. According to this strategy, by applying adhesives with the very low complex shear modulus, multiple neutral planes can be created within the display module, allowing for efficient stress dissipation[38-42]. These neutral planes are designed to offset the stresses, thereby enhancing the structural robustness of the device and preventing defects during flexible motions [Figure 2C]. The adhesives used in current foldable devices are meticulously designed to possess a suitable low complex shear modulus, considering factors such as productivity, creep and recovery properties, and adhesion force.
As foldable devices become more prevalent, the required properties for adhesives are generally well-defined. As flexible devices encounter diverse situations and users, new problems emerge, necessitating increasingly sophisticated specifications for adhesives to address these issues. However, even within the same category of foldable devices, the reliability requirements can vary significantly based on the device’s size (e.g., mobile phones, tablets, or laptops) and application (e.g., mobile or automotive). For example, foldable devices are categorized into foldable smartphones and laptops, each tailored for different usage environments and thus requiring distinct design specifications such as curvature radii and screen sizes. Smartphones, prioritizing portability, feature a small folding radius and a maximum unfolded screen size of 7.8 inches. Laptops, designed for larger displays, range from 13 to 17 inches. Due to greater exposure to external conditions, smartphones undergo more stringent UV stability and environmental testing, with reliability test temperatures ranging from -20 to 80 °C, compared to 0 to 60 °C for laptops. This results in vastly different specifications needed for flexible adhesives [Figure 2D]. Additionally, the stress applied to the display module varies greatly depending on the device’s form (e.g., U-shape, Ω-shape, Z-shape, or G-shape) and deformation mode (e.g., foldable, rollable, or stretchable). Therefore, the adhesive’s properties must be carefully considered based on the specific deformation shape and application of the device.
Slidable or rollable devices, which undergo two-dimensional pre-designed deformations, share similar mechanisms with foldable devices. However, unlike foldable devices that bend in specific areas, slidable or rollable devices transform across most of the screen area, significantly increasing adhesive deformation. While foldable devices use plastic OLED and thin film encapsulation to enhance flexibility, rollable TVs employ glass OLED and metal encapsulation, resulting in much greater stress during deformation. Additionally, the automotive sector, a major application area for space-saving slidable or rollable devices, demands adhesives with extreme thermal and UV stability beyond those used in conventional foldable devices. Therefore, research on rollable adhesives focuses on withstanding excessive deformations and enhancing environmental reliability[43]. To prevent extreme deformation, it is crucial to enhance the adhesive's cohesion properties, ensuring it does not tear under significant stress and can recover during unrolling. Cohesion is achieved through chemical crosslinking to form covalent networks and physical crosslinking, such as hydrogen bonding. Additionally, novel approaches such as using dynamic crosslinkers[44-49] or employing mechanophores[50-52] are being explored to increase cohesion without limiting deformation due to shear[53-55].
Stretchable displays, unlike previously mentioned device forms, undergo unexpected three-dimensional deformations. Current research on textile displays and medical sensors aims to advance the technological maturity of free-form displays[56-61]. Despite significant progress in driving cell technologies[58-67], such as serendipity circuits, island-type driving layers, and micro light-emitting diode (μLED) emission layers, research on adhesives for stretchable displays remains insufficient, and the required properties are not well-defined. However, insights from related fields, such as drug delivery systems or skin adhesives for medical sensors, could help identify necessary properties for display adhesives[57,68-75].
In addition to stretchability, adhesives for the drive part must be flowable enough to fill and flatten substantial unevenness caused by μLEDs and serendipity circuits. For applications such as medical sensors or textile displays, properties such as biocompatibility, easy detachability, water resistance, and chemical resistance are also crucial. Since stretchable devices often use elastic materials such as polydimethylsiloxane (PDMS) or polyurethane as substrates[65,76-82], conventional acrylic adhesives may not provide sufficient adhesion. This has led to suggestions for using silicone adhesives or adding adhesion promoters to acrylic adhesives. Hydrogel adhesives are also being researched as candidates due to their excellent biocompatibility and stretchability[83-86]. While the required characteristics of adhesives for stretchable displays can be anticipated, specific specifications remain uncertain. Thus, in-depth research is needed to clearly define these properties.
Table 1 summarizes the essential properties of adhesives for flexible devices with different form factors. Rigid and foldable adhesives have well-defined specifications based on the requirements of commercially available display devices. In contrast, the parameters for rollable and stretchable adhesives are less established, as they are still in the research and development stage and are derived from recent publications. These specifications are approximate and may vary depending on the device's size and purpose. In the next chapters, we explain in detail how Table 1 was derived. We will first define the key parameters for adhesives used in flexible devices with different form factors and explore strategies to achieve these parameters. Additionally, we will review the solutions researchers have developed to address the trade-offs among these key parameters.
The required properties of flexible adhesives for various forms and applications
Flat | Foldable | Rollable | Stretchable | |
Thickness (μm) | 100~200 | ≤ 50 | 50~100 | 50~100 |
Peel strength (N/cm) | > 7 | > 1 | > 5 | > 1 |
Recommended adhesive behavior | Hyperelastic | Viscoelastic + hyperelastic | Viscoelastic + hyperelastic | Hyperelastic |
Material group | Acrylate, silicone | Acrylate | Acrylate, silicone | Acrylate, silicone, rubber, hydrogel |
Glass transition temperature (Tg, °C) | - | < -30 °C | < -40 °C | - |
Storage modulus (G’, Pa, @RT) | ~106 | ~104 | ~105 | ~104 |
Creep (%, @RT) | - | 100%~200% | > 150% | > 250% |
Recovery (%, @RT) | - | > 80% | > 85% | > 90% |
Required environmental stability | -20 °C 60 °C / 90% RH 85 °C | -20 °C 60 °C / 90% RH 85 °C | -40 °C 85 °C / 85% RH 105 °C | - |
Fatigue stabilitya (@RT, dynamic/static) | - | > 1,000,000 times / 720 h | > 100,000 times / 1,000 h | > 1,000 times / N.D.c |
Fatigue stabilitya (@Environ.b, dynamic/static) | - | > 50,000 times / 300 h | > 10,000 times / 500 h | N.D.c |
UV stability | 2.4 W/m2 300 h | 2.4 W/m2 300 h | 55 W/m2 500 h | N.D.c |
Applications | TV, IT, mobile | IT, mobile | TV, auto | Medical sensor Textile display |
Additional requirements | - | UV-blocking | - | Biocompatibility Water-resistance Detachable |
KEY PARAMETERS FOR ADHESIVES IN FLEXIBLE DEVICES
Low modulus across a wide temperature range
Adhesives for flexible devices relieve stress across device modules by forming multiple neutral planes, which decouple strain in adjacent layers. The formation of these neutral planes and the resulting strain decoupling reduce the amount of stress and strain acting on each layer, thus preventing defects such as cracks or delamination. For these neutral planes to be effective, the adhesive’s complex shear modulus must be significantly low. An adhesive with a shear modulus of under 5 × 104 Pa at room temperature (RT) can alleviate overall module stress[15,25,35,39,87-91] [Figure 3A]. It is crucial to maintain the adhesive’s modulus close to its RT value across a wide range of operating temperatures to ensure the temperature reliability of flexible devices. Near and below the glass transition temperature (Tg), a rapid increase in complex shear modulus can disrupt the neutral plane design strategy. At higher temperatures, the polymer might become more flowable and lose elasticity, degrading its recovery properties. To prevent a rapid increase in modulus at low temperatures, low Tg monomers are introduced to lower the adhesive’s Tg close to the lower boundary of the industrial temperature range. This results in increased flowability near the upper boundary of the industrial temperature range, thus necessitating control through an appropriate introduction of chemical/physical crosslinking to manage cohesion. This strategy has been chosen to maintain low modulus across a wide temperature range. This approach helps maintain designed strain decoupling and neutral plane splitting within a broad temperature range, based on the properties set at RT. Therefore, to preserve the mechanical stability of flexible devices, the adhesive must maintain an extremely low modulus across a broad temperature range[24,42].
Figure 3. (A) The ideal shear modulus of flexible adhesives in temperature sweep test. With low Tg adhesives, it facilitates the formation of multiple neutral planes through the entire integrated structures; (B) DMA and rheometer are used to measure the viscoelastic properties of flexible adhesives such as temperature sweep, frequency sweep, creep and recovery, S-S curve, and so on; (C) The plastic-viscoelastic deformation occurs during the peel test. The stronger deformation occurs at peel front, the larger adhesion force is detected. Tg: Transition temperature; DMA: dynamic mechanical analysis.
Creep and recovery (Stress relaxation)
Adhesives for flexible devices must deform effectively in response to dynamic changes and return to their original state once external stress is removed[92]. Otherwise, repeated deformations could lead to permanent damage, such as buckling and screen distortion from wrinkles. As mentioned in Section “Low modulus across a wide temperature range”, although adhesives with an extremely low modulus offer superior stress relaxation compared to conventional adhesives, their enhanced flowability can adversely affect their recovery characteristics. Enhancing the recovery properties necessitates adjusting the polymer network through chemical/physical crosslinking and increasing polymer entanglement. The adhesive’s creep and recovery characteristics, as well as its stress relaxation properties, are analyzed using dynamic mechanical analysis (DMA) and a rheometer [Figure 3B].
To control the viscoelastic properties of the adhesive effectively, it is essential to manage both the combination of monomers and the properties of the polymer network that forms the adhesive. This polymer network is created through crosslinking and polymer entanglement. Polymer entanglement is closely related to viscoelasticity, while crosslinked networks primarily affect elasticity. The polymer network determines the rheological properties of the adhesive, which vary with temperature, frequency, and shear force. Rheological testing methods, such as frequency sweep, temperature sweep, and creep and recovery, enable detailed analysis of the adhesive polymer’s network[93-99]. Based on these analytical results, the adhesive polymer structure can be precisely adjusted to optimize its rheological properties, thereby enhancing the mechanical stability of flexible devices.
Adhesion strength
Compared to adhesives used in conventional rigid devices, those for flexible devices are thinner and have lower adhesion force, making it challenging to maintain sufficient bonding and ensure cohesive movement of adjacent layers[34,100-103]. The adhesion force is primarily determined by the interfacial interactions and the bulk deformation loss within the adhesive. During peel tests, energy dissipation occurs with the formation of a plastic-viscoelastic zone at the peel front, where the debonding process takes place [Figure 3C]. The formation of this zone is influenced by the adhesive thickness and peeling speed. As the thickness decreases, a fully developed plastic-viscoelastic zone cannot form at the peel front, leading to a drop in adhesion force[104]. For adequate bonding with adjacent layers, the adhesion force of flexible adhesives should be at least 1 N/cm, based on standards for foldable adhesives[34]. To achieve this, flexible adhesives should be formulated to allow for effective crosslinking, enhancing resistance to cohesive failure, and ensuring sufficient wetting and bonding at the interface[105-109].
Optical properties
In addition to the previously mentioned key characteristics, adhesives used in displays must exhibit optical clarity, especially in the front layers, and are classified as OCAs. Optical transparency and refractive index are critical to ensure the efficient transmission of light emitted from OLEDs without additional loss. To maintain the integrity of the OLED’s color, specifications related to color, such as the yellow index and Lab, are also crucial. Furthermore, driven by the trend towards minimizing components, enhancing light transmittance, and reducing power consumption in flexible devices, innovative technologies such as color filter on encapsulation (CoE) and touch on encapsulation (ToE) have been developed. These advancements have enabled the elimination of polarizers. In OLED devices, the role of the polarizer has diminished compared to its application in LCDs, yet it still performs critical functions such as blocking external light reflections, enhancing contrast ratio, and providing UV-blocking ability to prevent OLED degradation[110-112]. Since the removal of the polarizer in foldable devices, technological developments have been underway to address the reduction of external light reflections and enhancement of contrast ratio through the application of anti-reflection coatings on the cover window and the black matrix on color filters, respectively. However, since other layers cannot compensate for the UV-blocking ability required to prevent OLED degradation, it necessitates incorporating UV-block capabilities into the adhesive[27-29]. Reflecting these needs, recent research has advanced to incorporate the evolving requirements of display technology.
The aging resistance
Given that electronic devices are typically used over extended periods, the aging resistance of adhesives is critically important for maintaining their long-term functionality and reliability[113]. Aging resistance is the ability of an adhesive to preserve its physical and chemical properties over time, which is essential for ensuring the long-term reliability of flexible displays. Aging of adhesives typically results from exposure to factors such as heat, humidity, UV, oxygen, and various chemicals. These factors could change or degrade the polymer structure of the adhesive, thereby reducing its mechanical and rheological properties. For example, at high temperatures, the chemical bonds within the adhesive could be degraded by oxidation, reducing mechanical strength and adhesion[114]. Humidity and oxygen could accelerate the oxidation and hydrolysis reactions in the adhesive, weakening its flexibility and tensile strength[115]. To counteract these issues, adhesives could be formulated with stabilizers such as antioxidants, UV absorbers, and thermal stabilizers, to enhance aging resistance. Additionally, selecting monomers and crosslinkers with high thermal and moisture resistance could further improve aging resistance. To evaluate aging resistance, various accelerated aging tests can be conducted. These tests expose the adhesive to extreme conditions such as high temperatures, high humidity, and UV exposure to observe the long-term aging effects within a short period[116,117]. Beyond evaluating the adhesive itself, the aging resistance of adhesives can be further assessed by applying adhesives to display modules and conducting stability tests under varying temperature, humidity, and UV exposure conditions [Table 1]. These tests can identify phenomena such as bleaching, color changes, or bubbles resulting from adhesion loss. Collectively, ensuring the aging resistance of adhesives is essential to maintain the mechanical and optical reliability of flexible displays.
RECENT ADVANCES IN ADHESIVES FOR FLEXIBLE DEVICES
Employing finite element analysis for adhesive design
Finite element analysis (FEA) is a method that uses partial differential equations to numerically and mathematically simulate and analyze specific physical phenomena. This technique is particularly valuable for predicting the behavior of individual components, layered structures, or assemblies under various conditions. Researchers actively use FEA software in adhesive design to optimize layered components and determine the necessary physical properties of adhesives, thereby reducing trial-and-error experimentation and lowering costs. In the field of flexible devices, FEA with the modulus of the adhesives effectively predicts the mechanical stress distribution in multi-layered structures, identifies the location of neutral planes, and assesses the potential for delamination or cracking[14,16,25,39,118].
For example, FEA simulations have been utilized to analyze how variations in modulus and thickness of adhesives affect stress distribution across the entire device and impact adjacent elastic layers. Kim et al. demonstrated that using extremely soft adhesives prevents damage to electrodes by splitting the neutral plane[90]. They experimentally tested whether the indium tin oxide (ITO) electrodes formed on polyethylene terephthalate (PET) films were damaged during bending tests, depending on the modulus of the adjacent adhesive, by measuring the resistance of the electrodes. As shown in Figure 4A, the device with low modulus adhesives operated well without any damage to the ITO electrode.
Figure 4. (A) The multiple neutral planes formation applying low modulus adhesives in integrated structure[90]. Reproduced with permission. Copyright © 2017 IOP Publishing; (B) The location of multiple neutral planes is controlled by a combination of high and low modulus adhesives in top and bottom sides[119]. Reproduced with permission. Copyright © 2020 KMEPS (CC BY NC); (C) The strain splitting of rigid layers (orange) occurs well with increasing thickness of adhesives through forming neutral multiple planes[40]. Reproduced with permission. Copyright © 2016 The Royal Society.
Another study by Ma et al. reported how the modulus of adhesives in rollable devices affects the location of the neutral plane and strain decoupling in adjacent layers[119]. They indicated that by adjusting the properties of the adhesive layers, it is possible to place the neutral plane in brittle and hard-to-replace layers such as thin-film transistors (TFT), electroluminescent (EL) layers, and electrodes, thereby enhancing the mechanical stability of the device [Figure 4B].
Li et al. conducted research showing that the primary deformation of adhesives during flexible operations is shear deformation[120]. They found that thicker adhesives better facilitate the formation of neutral planes through shear deformation, effectively decoupling strain in rigid layers[40] [Figure 4C]. Strain decoupling refers to the phenomenon where a layer with extremely low modulus between two high modulus layers causes stress inversion through shear deformation in the middle layer, resulting in independent strain behavior in the top and bottom layers. These studies suggest that a stack structure with alternating high-elastic films and extremely low-modulus adhesives can effectively alleviate stress in the device by facilitating the formation of multiple neutral planes, thereby ensuring mechanical stability[121].
The aforementioned studies assumed that adhesives behave as linear elastic materials. However, this approach does not account for the complex shear deformations caused by hyperelasticity and viscoelasticity, leading to notable discrepancies when simulating smaller folding radii or more complicated deformations such as rollable or stretchable forms[26]. Hyperelasticity represents nonlinear elastic behavior, while viscoelasticity reflects changes in material properties over time[122].
To accurately predict the real behavior of devices, it is necessary to use theoretical models that account for both hyperelasticity and viscoelasticity, matching the measured properties of adhesives and incorporating these into simulations[25,26,92,122-125]. Hyperelasticity is typically derived from stress-strain curves and represented using models such as the Ogden, Yeoh, or Mooney-Rivlin models, which are based on the strain energy density function. Viscoelasticity, which is time-dependent, is represented by properties extracted from stress relaxation curves or master curves of adhesives, often using the generalized Maxwell model or the standard linear model, represented by Prony series.
Zhang et al. analyzed the actual behavior of flexible adhesives using various fitting models to determine the most appropriate one[125]. They measured stress-strain curves at different strain rates to find the optimal model for hyperelastic properties and correlated the data with the Yeoh, Ogden, and Mooney-Rivlin models. They concluded that the 5-parameter Mooney-Rivlin model best fits all data sets regardless of strain rate. For viscoelastic properties, they measured stress relaxation and master curves at different temperatures and found that the generalized Maxwell model, using the 3rd Prony series, provided a good fit. This research underscores the importance of selecting the constitutive model that best represents the genuine properties of the adhesive, which is crucial for improving the accuracy of simulation results.
Reflecting these insights, recent studies have significantly advanced the understanding of the role of adhesives in flexible displays and the stress distribution across different device shapes. Jia et al. incorporated hyperelastic properties into their FEA simulations using the 3rd Yeoh model. Their results[122], as shown in Figure 5A, indicate that increasing the thickness of the first OCA layer reduces the maximum strain, primarily affecting only the adjacent layer. This can be attributed to strain decoupling in the rigid layers due to the stress relaxation of the low modulus adhesive, as well as the shielding effect that obscures the influence between adhesives caused by the rigid layers.
Figure 5. (A) The changes of max strain based on OCA thickness (blue shadow, left) and the shielding effect of rigid layer (right). The more distant layers are less affected by 1st adhesive thickness[122]. Reproduced with permission. Copyright © 2019 Elsevier B.V.; (B) The max stress distribution based on folding shape. The Ω-shape is favorable for reducing folding radius[123]. Reproduced with permission. Copyright © 2022 MDPI (Basel, Switzerland) (CC BY); (C) The strain splitting across all rolling angle ranges is modeled in the FEA simulation by inputting viscoelastic data of the adhesives[26]. Reproduced with permission. Copyright © 2023 Springer Nature Limited (CC BY). OCA: Optically clear adhesive.
Niu et al. used the 4th Ogden model and Prony model to incorporate hyperelastic and viscoelastic properties into FEA simulations, respectively, predicting stress variations for different folding shapes
Han et al. reported that incorporating the measured properties of adhesives into rollable display simulations provides a more precise prediction of stress distribution compared to using conventional linear elastic models[26]. When using a conventional linear elastic model for OCAs, multiple neutral planes do not form well across the entire rolling angle range. However, incorporating nonlinear elastic properties with the Yeoh model results in well-distributed stress and strain throughout the rolling region [Figure 5C].
Advancements in FEA modeling enable accurate predictions of the intricate physical behavior in stacked structures, providing researchers with logical and visual insights. The ability to rapidly accumulate extensive data makes FEA simulations valuable for developing artificial intelligence (AI) models that identify mechanically stable structures and determine the necessary physical and mechanical properties of component layers.
Materials selection
To facilitate the formation of multiple neutral planes and manage sudden changes in modulus at low temperatures, adhesives should have an extremely low modulus, achieved by lowering the polymer’s Tg. Adhesives with a low Tg maintain viscoelastic behavior similar to that at RT over a wider temperature range, including low temperatures[126-130]. Monomers with long or branched alkyl chains, which have a high free volume, act as low Tg monomers. Examples include 2-ethylhexyl acrylate (EHA, Tg: -68 °C), butyl acrylate (BA, Tg: -55 °C), and isononyl acrylate (iNA, Tg: -58 °C). Typically, adhesives consist of a combination of low Tg monomers, high Tg monomers, and functional monomers. Flexible adhesives are primarily composed of extremely low Tg monomers combined with monomers possessing hydrophilic functionalities, which influence the wettability and cohesion of the adhesive. Commonly used monomers with hydrophilic functionalities include 4-hydroxybutyl acrylate (HBA), 2-hydroxyethyl acrylate (HEA), and acrylic acid (AA).
Following this monomer selection strategy, Lee et al. used EHA as the low Tg monomer and investigated the impact of various functional monomers on stretchability[126,127]. They incorporated acrylamide (AM), methyl acrylate (MA), AA, and HEA to create adhesives that fully recovered after 25% elongation, demonstrating significant flexibility. Stress-strain hysteresis analysis confirmed the recovery properties imparted by the functional monomers. Cohesion through entanglement and hydrogen bonding with AM or AA resulted in significant hysteresis loss, while HEA showed minimal loss, indicating its superiority for recovery in stretchable adhesives. This study suggested HEA as a suitable functional monomer for stretchable adhesives [Figure 6A]. The same research group developed a fully reversible adhesive with minimal stress-strain hysteresis using 2-carboxyethyl acrylate (CEA) as a functional monomer alongside EHA[128]. They determined that the recovery delay, previously identified due to carboxy groups, was caused by strong hydrogen bonds formed by these groups after deformation, resulting in residual strain[126]. They found that the residual strain was dependent on the amount of CEA and remained consistent even after repeated experiments. To address this issue, they introduced a pre-strain strategy. The pre-strained adhesive demonstrated immediate recovery without hysteresis loss after additional deformation [Figure 6B]. This study is significant as it resolves the recovery delay issue by analyzing the characteristics of the monomer, particularly when incorporating a carboxy-containing monomer that dramatically enhances adhesion properties.
Figure 6. (A) The stretch hysteresis of several acrylate adhesives. Incorporating with HEA or MA, the elastic energy is conserved rather than lost as heat[126]. Reproduced with permission. Copyright © 2019 Elsevier Ltd; (B) The pristine adhesive remained ~10% residual strain after 100 cycle hysteresis due to its newly formed hydrogen bonds. The pre-strained adhesive was recovered immediately[128]. Reproduced with permission. Copyright © 2022 Elsevier B.V.; (C) The dynamic temperature sweep (C1) and creep and recovery test (C2) of adhesives with ethylene glycol silane acrylate. It enhanced cohesion and controlled the shear flow[132]. Reproduced with permission. Copyright © 2023 MDPI (Basel, Switzerland). HEA: 2-hydroxyethyl acrylate; MA: methyl acrylate.
Studies have also explored the application of specialized monomers, such as silane acrylates and ethylene glycol-containing acrylates, in flexible adhesives. Seok et al. demonstrated that using various silane acrylates increases the polymer’s free volume due to the larger Si−O−Si bond angle compared to the C−O−C bond angle, effectively lowering the shear modulus at low temperatures (≤ -20 °C)[131]. They also showed that ethylene glycol silane acrylate ensures polymer entanglement through physical crosslinking from ethylene glycol, while the bulky silane group reduces the low-temperature modulus, resulting in a stable modulus across a wide temperature range[132]. Creep and recovery tests confirmed that the bulky silane group controls the elasticity of acrylic adhesives, preventing excessive deformation [Figure 6C].
Additionally, studies are being conducted on hydrogels, demonstrating their potential as adhesives for stretchable and wearable devices through 2-axis stretch tests[83-86]. These studies utilize substances that act as movable linkers, such as cyclodextrin or sulfonic acid, or use polymers such as polyvinylpyrrolidone (PVP), which easily form a hydrogen bond. As adhesives, hydrogels exhibit desirable properties in folding tests or 2-axis stretch tests, stretching and recovering without tearing or buckling issues. For example, Han et al. designed a novel hydrogel adhesive with a dopamine-containing diacrylate crosslinker[86]. Tri(ethylene glycol) diacrylate reacted with dopamine via the aza-Michael reaction to synthesize the crosslinker, which was then copolymerized with AA to create the hydrogel adhesive. This hydrogel demonstrated a high lab shear strength of about 70 kPa on skin tissue, attributed to the hydrophilic dopamine unit. It also showed complete recovery without residual strain up to 150% elongation. Given their biocompatible and stretchable properties, hydrogels are considered suitable materials for stretchable applications, emphasizing the need to develop mechanically stable hydrogels similar to conventional acrylic adhesives.
These investigations have highlighted that the structure of monomers can significantly influence the overall properties of a polymer, such as its Tg, complex shear modulus, elasticity, and recovery properties. The geometry or composition of monomers and linkers affects not only the structure of the polymer network but also determines rheological properties such as the temperature stability of the complex shear modulus, creep, recovery, and adhesion properties.
Optimizing network structure
Polymer networks in adhesives are formed through two primary mechanisms: chemical/physical crosslinking and entanglement. Chemical crosslinking typically occurs via crosslinkers or hydrogen atom transfer (HAT) processes[133-135], creating covalent bonds. Physical crosslinking, on the other hand, happens through secondary interactions such as hydrogen bonding or coordination bonds, allowing for dynamic exchange. In network polymers, the gel content can be experimentally quantified to determine the proportion of crosslinked polymer within the entire polymer matrix. The crosslinking density can also be estimated from the storage modulus in the rubbery region during a temperature sweep.
Entanglement results from the interlocking of polymer backbones or long side chains and is significantly influenced by the molecular weight and functionality of the polymer. The molecular weight can be controlled by polymerization conditions, including the amount of the initiator, reaction time, and temperature[130,136,137]. When polymers have a molecular weight above the entanglement molecular weight (Me), the flowability of the polymer is restricted through inter- or intra-polymer entanglements[138,139]. Increased entanglement strength broadens the plateau region observed in rheological data, indicating its impact on the polymer’s elasticity. Particularly, a low Tg polymer with appropriate cohesion through polymer configuration can achieve a wider temperature range of shear modulus stability, encompassing both low and high temperatures, and exhibit excellent recovery properties.
The use of diacrylate crosslinkers to induce chemical crosslinking is common practice. Adjusting the structure and quantity of the crosslinker allows control over the crosslinking density. Generally, shorter, structurally rigid crosslinkers, and linkers with stronger secondary interactions result in higher polymer crosslinking density, thereby enhancing cohesion properties. To increase the cohesion of flexible adhesives, researchers have introduced chemical and physical crosslinking methods or explored novel linker designs. These studies are crucial for guiding the design of polymer networks using crosslinkers.
Yi et al. also investigated the rheological properties resulting from the formation of crosslinking networks using cyclodextrin-based acrylate (CDA) and 1,6-Hexanediol diacrylate (HDDA)[87]. They studied three network structures: the covalent crosslinking network (CCN) formed solely with HDDA, the movable crosslinking network (MCN) with CDA alone, and the confined sliding network (CSN) with both HDDA and CDA. The investigation revealed significant differences in rheological properties, particularly in strain recovery. Polymers with CCN and CSN, which possess chemical crosslinking, demonstrated over 80% strain recovery due to their elasticity. In contrast, the MCN polymer, featuring only physical crosslinking, exhibited poor strain recovery of 50% due to plastic deformation. During a 3mmR folding test, the CCN polymer sample cracked and delaminated, while the CSN polymer sample showed no defects [Figure 7A]. This suggested that the strictly crosslinked structure in the CCN polymer, resulting from full chemical crosslinking, induces a stiff network that leads to crack initiation and subsequent delamination.
Figure 7. (A) The different crosslinked network structure determines the strain recovery property and foldability. The CSN network is suitable for flexible adhesives because it incorporates both covalent crosslinked network and movable crosslinked network, allowing controlled movement of the polymer[87]. Reproduced with permission. Copyright © 2022 Elsevier B.V.; (B) The PEG contained urethane acrylate linker shows superior strain recovery rate compared with commercial linker[2]. Reproduced with permission. Copyright © 2023 Wiley‐VCH GmbH; (C) The hyperelastic adhesive prevents wrinkles after deformation. Viscoelastic materials on the surface and hyperelastic materials in the bulk created a heterogeneous network[140]. Reproduced with permission. Copyright © 2022 American Chemical Society; (D) The cross-linked network is formed by photo iCVD method. The frequency sweep test and the dynamic temperature sweep test exhibit network formation of polymer indicating plateau region and rubbery region[141]. Reproduced with permission. Copyright © 2018 American Chemical Society; (E) The crosslinking density of adhesive could be controlled by manipulating UV intensity through grayscale mask[144]. Reproduced with permission. Copyright © 2021 Elsevier B.V.; (F) The schematic depicts the bicontinuous phase of a heterogeneous adhesive induced by polymerization-induced microphase separation[145]. Reproduced with permission. Copyright © 2024 Wiley‐VCH GmbH. CSN: Confined sliding network; PEG: poly(ethylene glycol); iCVD: initiated chemical vapor deposition; UV: ultraviolet.
Park et al. overcame the limitations of traditional crosslinkers, such as their rigid structure and consequent reduction in adhesion, by designing a novel urethane acrylate linker[2]. They incorporated two key concepts into the core structure. First, they used m-Xylylene diisocyanate (XDI) and 1,3-bis(isocyanatomethyl)cyclohexane (H6XDI) to enhance light stability and strength; Second, they employed poly(ethylene glycol) (PEG)-acrylate to improve chain mobility and cohesion, resulting in the creation of PEG-contained urethane diacrylate. The XDI-PEG linker (XPD) and H6XDI-PEG linker (HPD) were compared to the commercially used linker HDDA. The findings showed that, while maintaining similar adhesion properties, the recovery speed at 20% strain was significantly faster [Figure 7B]. Notably, HPD exhibited a strain recovery speed of over ten times faster. This rapid recovery is attributed to the cyclohexane in HPD acting as a soft segment with PEG, providing flexibility and limiting urethane hydrogen bond formation due to steric hindrance. Conversely, XPD showed a slower recovery speed compared to HPD, as the benzene acts as a hard segment in the linker and allows urethane hydrogen bond formation. This study suggests that in designing crosslinkers, ensuring sufficient mobility through the use of soft moieties and suppressing hydrogen bond formation can significantly improve the strain recovery rate of adhesives.
Zhang et al. studied how network structure affects the behavior of stretchable adhesives[140]. They found that adhesives with hyperelastic properties recover well without wrinkles after deformation, unlike those with viscoelastic properties. Their adhesive system involves coating an elastic adhesive surface with a viscoelastic adhesive, creating interlinks between the elastic and viscoelastic polymers, forming a heterogeneous network. This combination of interlinks and conventional crosslinks preserves the overall elasticity of the adhesive while maintaining interface adhesion and shear flow due to the viscoelastic polymer [Figure 7C]. A similar strategy involving the formation of a heterogeneous network was also employed by Jeong et al., who developed an adhesive using PDMS as the hyperelastic layer[42]. This adhesive maintains the extremely low modulus even at -50 °C, ensuring foldability without delaminations or cracks across a broad temperature range from -50 to 100 °C. The significance of their research lies in maintaining the heterogeneous network while ensuring low modulus across the harsh temperatures. These studies highlight the importance of elasticity for flexible adhesives by introducing new lamellar structured adhesives.
Additionally, the degree of crosslinking can be controlled by modifying the manufacturing process. Unlike traditional methods, using photo-initiated chemical vapor deposition (iCVD) allows the formation of crosslinked networks without the need for crosslinkers[24,141]. Moon et al. revealed that adhesives produced by thermal iCVD do not form crosslinked structures, whereas those produced by photo iCVD do[141]. By using both thermal and photo iCVD simultaneously, the degree of crosslinking can be adjusted, which affects the formation of the plateau region in frequency sweeps and the rubbery region in temperature sweeps [Figure 7D].
Adjusting the UV light intensity or exposure time can effectively control the crosslinking density of adhesives prepared by photocuring. Kim et al. demonstrated that increasing the UV exposure time, while maintaining a constant light intensity, enhances the gel content. This increase in gel content promotes the formation of the crosslinked network, allowing for precise control over stress relaxation properties[142,143]. Employing this approach, they fabricated patterned adhesives with varying crosslinking densities by manipulating the UV dosages. They cured the low-cured area via primary UV curing and formed the high-cured area through secondary curing using the photomask. Compared to the non-patterned sample, the patterned sample showed a significant improvement in the recovery performance. The patterned sample also exhibited a relatively high modulus compared to the non-patterned sample, while demonstrating similar adhesion strength. Subsequently, by changing the gray scale and size of the patterned mask, they effectively tuned the density of crosslinked network[144]. Smaller pattern sizes enhanced adhesion forces and recovery properties, demonstrating the potential of UV-patterned adhesives as flexible adhesives [Figure 7E]. These studies highlight that the crosslinked network can be controlled not only by changing materials but also through manufacturing processes, offering significant insights into the versatility of adhesive technologies.
Recently, Back et al. successfully developed a highly resilient adhesive with low crosslinking density but excellent strain recovery characteristics by leveraging polymerization-induced microphase separation (PIMS) that incorporates both hard and soft domains to form a nano-scaled bicontinuous phase[145]. When a macro chain transfer agent (CTA) and monomers are blended and cured, the linear monomer blocks grown from the CTA ends are kinetically trapped by the crosslinked polymer domains, resulting in phase separation that ultimately forms a nanoscale bicontinuous phase [Figure 7F]. In the bicontinuous phase, the soft domains, formed solely by entanglement without crosslinking, deform immediately under folding strain, allowing for a wide range of deformation, while the hard domains, possessing a crosslinked network, exhibit superior recovery characteristics that enable them to return to their original state once external deformation is removed. This research presents a significant strategy that overcomes the limitations previously faced in controlling the behavior of traditional flexible adhesives, where it was challenging to independently control flowability and recovery properties solely based on gel contents, i.e., the content of crosslinked polymers.
Sustainability
For several decades, environmental concerns have driven increased attention to the sustainability of materials across various fields[146-152]. This societal interest has significantly influenced the research and development of adhesives, with studies focusing on monomer perspectives, manufacturing methods, and post-use considerations.
To produce acrylate monomers in an environmentally friendly manner, AA reacts with hydroxy-containing materials derived from natural sources[91,153-163]. AA, a crucial precursor for acrylate monomers, has already been commercialized using an eco-friendly method[164-167]. Commonly used hydroxy-containing sources include terpenoid and lignin-based materials [Figure 8A]. Depending on the type of hydroxy-containing material used, either high Tg monomers or low Tg monomers are produced. High Tg monomers such as menthyl acrylate and isobornyl acrylate have been extensively studied[168-171], while innovations in low Tg monomers have introduced candidates such as stearyl acrylate, 2-octyl acrylate, and tetrahydrogeranyl acrylate (THGA)[172-174]. Functional monomers, such as HBA and HEA, can be derived from CO2 or biomass-derived ethylene glycol[158,175].
Figure 8. (A) The representative starting materials for preparing bio-based acrylate monomers. All starting materials can be obtained from plants or trees; (B) The photocatalyst (blue) and co-initiators (green) for visible-light-driven polymerization. Using the photocatalyst and co-initiators, the PIS mechanisms were proposed[27]. Reproduced with permission. Copyright © 2024 Springer Nature; (C) The concept and preparation method for debondable adhesives. The UV is a stimulus for adhesion control[190]. The mechanism of adhesion control was proved through MD simulation. Reproduced with permission. Copyright © 2024 Wiley‐VCH GmbH; (D) Thermo-switchable adhesion is achieved by controlling the number of hydrogen bonding sites[196]. Reproduced with permission. Copyright © 2024 Royal Society of Chemistry. PIS: Photoinitiating system; UV: ultraviolet.
Baek et al. have utilized tetrahydrogeranyl (meth)acrylates to create UV-curable adhesives with a Tg between -20 and 30 °C, demonstrating the potential for plant-derived ingredients in flexible adhesives[171,172]. Park et al. successfully prepared OCAs for foldable displays using fully terpene-based monomers[91]. They selected two terpenes: tetrahydrogeraniol from geranium and citronellol from rose oil. THGA, chosen as a low Tg monomer, and vicinal diol-contained citronellol-based acrylate (CDA) were identified as suitable functional monomers for flexible adhesives. The resulting OCAs exhibited low Tg and moduli across a wide temperature range. Their foldability was confirmed through a 2 mmR dynamic folding test under various conditions.
Current adhesive production methods predominantly involve solvent-based thermal polymerization and solvent-free photopolymerization. To make these processes more environmentally friendly, several approaches have been suggested, including the use of water and other eco-friendly solvents[176-181], as well as substituting UV light with harmless visible light[182-188]. Noppalit et al. used cyclademol and tetrageraniol-based (meth)acrylates to produce adhesives with a Tg of -30 °C, employing a miniemulsion polymerization method that uses both toluene and water as solvents, reflecting an environmentally conscious manufacturing approach[178]. Kwon et al. successfully developed a highly efficient visible-light photoinitiating system (PIS) by combining a previously developed visible-light-absorbing photocatalyst combined with appropriate co-initiators[27,189] [Figure 8B]. Unlike existing visible-light PIS, this new system can efficiently facilitate polymerization with a minimal amount of photocatalyst, enabling the production of highly transparent OCAs suitable for display applications. Compared to existing UV systems, this method is energy-efficient, ensures user safety, and allows for the creation of functional OCAs previously unattainable with UV-light curing. The visible-light curing system can be easily scaled up using halogen lamps or LEDs, making it a cost-effective alternative to expensive xenon lamps for UV curing and directly applicable to industrial processes. Building upon this technology, they have developed UV-blocking[27,28], UV-debondable[190], and sustainable OCAs[91].
Research on the after-use of adhesives has gained attention due to the challenges of recycling or disposing of them, mainly caused by their crosslinked networks and the tackiness of residues left on surfaces[190-197]. Several research groups have been investigating methods, such as stimuli-responsive adhesive, to selectively control adhesion forces for clean detachment from surfaces[190,196,197]. Stimuli-responsive adhesives are crucial in the display industry as they enable error correction and facilitate the recycling of display components after use. Recent studies by Kim et al. have focused on adhesion control for displays using benzophenone moieties that respond selectively to UV light[190]. This allows additional crosslinking responding to post-UV irradiation, which increases cohesion while reducing adhesion force, thereby enhancing the recyclability of displays or cover glass after use [Figure 8C]. Hwang et al. reported on an adhesive with switchable adhesion properties activated by temperature changes[196]. The lower critical solution temperature (LCST) phenomenon of N-isopropylacrylamide is a key factor in modulating adhesive strength based on temperature. They successfully demonstrated that the adhesion force is reversible, with high adhesion forces at RT and 97% reduction in adhesion at 90 °C, by utilizing changes in inter- and intra-hydrogen bonding formation [Figure 8D].
For several years, research on (bio)degradable adhesives has been actively advancing, with studies focusing on copolymerizing acrylate monomers with degradable units and creating adhesives entirely from degradable monomers[198-209]. Current research underscores the need to understand how degradable units influence rheological properties. This insight is crucial for developing adhesives that maintain high performance while minimizing environmental impact.
Various optical properties for flexible devices
The adhesive used above the light-emitting layer in display devices must be optically transparent to ensure that light reaches the user without any loss. Additionally, its refractive index (n) should closely match that of the cover glass to prevent light loss due to surface reflection or diffraction. Acrylic adhesives, with n = 1.49, are well-suited for this purpose. Their refractive index is slightly lower than that of cover glass (n~1.50) and slightly higher than that of TAC film (n~1.47) used in polarizers, minimizing light loss at interfaces. Moreover, the adhesive must be colorless to avoid distorting displayed colors. This can be verified using parameters such as the yellowness index and the Lab color index to ensure a true white color.
Recent trends in flexible devices aim to enhance energy efficiency, minimize thickness, and reduce manufacturing costs by utilizing multifunctional layers. A notable example is the replacement of polarizers with color filters in new OLED displays [Figure 9A]. However, removing the polarizer exposes the OLED to UV light, potentially accelerating the degradation of emitters. This underscores the need for UV-blocking adhesives. These adhesives are also in high demand for automotive displays, which are exposed to strong sunlight for extended periods. Traditional UV-cured polymerization processes face challenges when incorporating UV-cut additives, as UV light is essential for curing.
Figure 9. (A) The conventional OLED module structure (left) and pol-less OLED module structure (right). Excluding the polarizer necessitates the introduction of a black matrix on the color filter and a UV-blocking adhesive; (B) The UV-blocking adhesives were prepared via visible-light-driven polymerization. With UV-block adhesive, the luminescence and voltage change were negligible after UV exposure test[27]. Reproduced with permission. Copyright © 2024 Springer Nature. OLED: Organic light-emitting diode; UV: ultraviolet.
To address this, Kwon et al. utilized a visible-light-driven polymerization method to successfully create a flexible adhesive with UV-blocking capabilities[27]. This adhesive not only completely blocks UV light below 400 nm but also uses minimal amounts of a photocatalyst that absorbs visible light, ensuring optical transparency and colorlessness. Additionally, the rapid polymerization process makes it economically viable. The UV-blocking adhesive effectively blocks UV light, preventing luminescence loss and voltage drop in blue OLEDs [Figure 9B]. This study is significant for its industrial applicability and for incorporating additional functions without compromising the characteristics of existing flexible adhesives.
PERSPECTIVES
With the advancement of information technology (IT) infrastructure and increasing demand for real-time information sharing, display form factors are expected to become more diverse and complex. The development of innovative designs such as foldable, rollable, and transparent displays enhances consumer convenience and introduces new user experiences. As these devices evolve, the adhesives used in them are also being developed to meet various requirements, with ongoing efforts to push existing material properties to their limits or incorporate new functionalities. While adhesives for foldable phone and laptop/tablet displays are relatively well-developed, those for automotive displays still require improvements due to more stringent reliability conditions. According to the temperature standards for existing automotive displays[210], adhesives must maintain their rheological properties over a broader range, ensuring reliability down to
Rollable displays, which offer significant enhancements in space utilization and portability, are particularly promising for automotive applications and thus demand high environmental reliability. Adhesives for these displays need to endure considerable deformation without tearing and recover effectively when unrolled, requiring a blend of existing techniques and innovative approaches. Similarly, adhesives for stretchable displays, such as those used in attachable medical sensors or textile displays, necessitate additional functionalities. These adhesives should exhibit well-defined properties tailored to specific applications, including the capacity to fill micro LED step heights and considerations for biocompatibility and water resistance. Textile displays, in particular, demand adhesives with fiber compatibility, enhanced wash durability, and chemical resistance, necessitating a sophisticated set of properties. As evidenced, the properties required for adhesives used in rollable or stretchable displays are not as well-defined as those used in foldable displays. Therefore, in-depth research is essential to identify the target properties crucial for ensuring the durability and reliability of these devices.
Additionally, adhesives used in all types of flexible displays must ensure long-term stability to maintain the durability of the display and the device in which it is utilized. The repeatability and stability of adhesives are crucial for the practical application of flexible devices. Given that flexible devices such as foldable smartphones, rollable TVs, wearable sensors, and human-computer interaction displays are used over long periods, these adhesives should retain their properties without degradation, demonstrating aging resistance and maintaining mechanical stability despite repeated flexible motions. Furthermore, aging resistance against environmental conditions such as temperature, humidity, and UV can be accomplished by appropriately formulating the monomers and additives that compose the adhesive, thereby creating a stable adhesive under various environmental conditions.
In the future, flexible devices will need to operate reliably in extreme environments, be thinner yet more efficient, and exhibit greater energy efficiency. Consequently, future adhesives must maintain consistent viscoelastic properties over a wide temperature range, offer improved impact resistance despite low thickness, and provide versatile functionalities. These functionalities may include UV blocking properties to protect against everyday UV exposure and excellent heat dissipation properties to manage thermal loads. Increasing energy efficiency can be approached from various perspectives. From the standpoint of using a flexible device longer within a limited battery capacity, energy efficiency can be enhanced by minimizing the drop-in brightness from the display to the user. This can be achieved by matching the refractive index of the adhesive with adjacent layers and maximizing optical transmittance. Another perspective is to look at the production aspect of adhesives. Energy efficiency in adhesive production can be increased by using faster curing speeds and lower intensity light sources, which can be achieved by utilizing efficient photocatalysts and initiators. Additionally, the development of flexible adhesives must support environmental sustainability. There is significant demand for OCAs made from degradable, recyclable, or reusable eco-friendly materials, aligning with global sustainability goals. This requires a comprehensive approach encompassing the entire lifecycle of adhesives, from monomer synthesis and polymerization to post-use disposal or recycling, ensuring they meet technical requirements while contributing to a sustainable future.
In summary, the requirements for adhesives used in flexible displays become increasingly complex as display form factors evolve and functional demands grow. For foldable displays, particularly as the technology matures in mobile phones, adhesives must reliably operate over a wider temperature range and provide excellent UV blocking capabilities to expand their use to automotive devices. Conversely, adhesives for rollable and stretchable displays are not yet fully commercialized, so their exact specifications remain undetermined. However, these adhesives need superior viscoelastic properties and functionality to perform reliably under broader temperature ranges and harsher mechanical deformation compared to those for foldable displays. As commercialization progresses and user feedback is gathered, these specifications will be refined and improved. Additionally, global requirements for environmental sustainability must be considered, necessitating active research into adhesives that can be degraded or reused in flexible displays.
DECLARATIONS
Authors’ contributions
Conceived the topic and supervised the articles: Kwon MS
Wrote, discussed, and revised the manuscript: Park Y, Kim J, Kim D, Lee S, Hwang D
Availability of data and materials
Not applicable.
Financial support and sponsorship
This paper was supported by the Technology Innovation Program (20011317, Development of an adhesive material capable of morphing more than 50% for flexible devices with a radius of curvature of 1 mm or less) and “Development of PFAS-free, eco-friendly, and highly reliable module materials for automotive foldable displays (RS202400419769)” funded by the Ministry of Trade, Industry & Energy (MOTIE, Korea). It was also backed by LG Display through the LGD-SNU Incubation Program.
Conflicts of interest
All authors declared that there are no conflicts of interest.
Ethical approval and consent to participate
Not applicable.
Consent for publication
Not applicable.
Copyright
© The Author(s) 2024.
REFERENCES
1. Koo JH, Kim DC, Shim HJ, Kim T, Kim D. Flexible and stretchable smart display: materials, fabrication, device design, and system integration. Adv Funct Mater 2018;28:1801834.
2. Park H, Lim D, Lee G, Baek MJ, Lee DW. Tailoring pressure sensitive adhesives with H6XDI-PEG diacrylate for strong adhesive strength and rapid strain recovery. Adv Funct Mater 2023;33:2305750.
3. Kim J, Shim HJ, Yang J, et al. Ultrathin quantum dot display integrated with wearable electronics. Adv Mater 2017;29:1700217.
4. Jeong YC. P-212: Late-News Poster: flexible cover window for foldable display. Symp Digest Tech Papers 2018;49:1921-4.
5. [SID 2021 Keynote speech] “The metaverse and the great future of display”. Available from: https://www.samsungdisplay.com/eng/media/movie/detail/movie210520.jsp. [Last accessed on 30 Jul 2024].
6. Kim DW, Kim SW, Lee G, et al. Fabrication of practical deformable displays: advances and challenges. Light Sci Appl 2023;12:61.
7. Bi S, Gao B, Han X, et al. Recent progress in printing flexible electronics: a review. Sci China Technol Sci 2023;67:2363-86.
8. Benedek I, Feldstein MM. Applications of pressure-sensitive products. 1st ed. Boca Raton: CRC Press; 2008.
9. Satas D. Handbook of pressure sensitive adhesive technology. New York, NY: Springer; 1989. Available from: https://link.springer.com/book/9781475708684. [Last accessed on 26 Jul 2024].
10. Acrylic pressure sensitive adhesives (PSA) market size & share analysis - growth trends & forecasts (2024-2029). Available from: https://www.mordorintelligence.com/industry-reports/acrylic-pressure-sensitive-adhesives-market. [Last accessed on 26 Jul 2024].
11. Gengnagel C, Hernández EL, Bäumer R. Natural-fibre-reinforced plastics in actively bent structures. Proc Inst Civ Eng Constr Mater 2013;166:365-77.
12. Kim TW, Lee JS, Kim YC, Joo YC, Kim BJ. Bending strain and bending fatigue lifetime of flexible metal electrodes on polymer substrates. Materials 2019;12:2490.
13. Ugural AC, Fenster SK. Chapter 5: Bending beams. In: Advanced mechanics of materials and applied elasticity. 5th ed. Prentice; 2019. pp. 226-91. Available from: https://ptgmedia.pearsoncmg.com/images/9780137079209/samplepages/0137079206.pdf. [Last accessed on 30 Jul 2024].
14. Yeh M, Chang L, Cheng H, Wang P. Bending stress analysis of laminated foldable touch panel. Procedia Eng 2014;79:189-93.
15. Nishimura M, Hishinuma M, Yamaguchi H, Murayama A. 56-3: quantitative evaluation of neutral-plane splitting in foldable displays using folding stiffness measurements and finite element method simulations. SID Int Symp Dig Tech 2020;51:834-7.
16. Chae Y, Chae GS, Youn YO, Woo S, Shin SH, Lee J. Optimal design of thickness and young’s modulus of multi-layered foldable structure considering bending stress, neutral plane and delamination under 2.5 mm radius of curvature. Int J Precis Eng Manuf 2018;19:1143-54.
17. Hwang B, Lim S, Park M, Han S. Neutral plane control by using polymer/graphene flake composites for flexible displays. RSC Adv 2017;7:8186-91.
18. Park Y, Bang S, Hwang B, et al. 69-2: Development of flexible cover window for large foldable display with a pen-touch function. Symp Digest Tech Papers 2021;52:1033-5.
19. Choi GM, Jin J, Shin D, et al. Flexible hard coating: glass-like wear resistant, yet plastic-like compliant, transparent protective coating for foldable displays. Adv Mater 2017;29:1700205.
20. Jeong YC, Kim DG, Kim H. 66-1: invited paper: flexible yet robust cover window with enhanced bending stiffness. Symp Digest Tech Papers 2023;54:932-5.
21. Jeong SY, Shim HR, Na Y, et al. Foldable and washable textile-based OLEDs with a multi-functional near-room-temperature encapsulation layer for smart e-textiles. npj Flex Elect 2021;5:15.
22. Jeong EG, Kwon JH, Kang KS, Jeong SY, Choi KC. A review of highly reliable flexible encapsulation technologies towards rollable and foldable OLEDs. J Inform Display 2020;21:19-32.
23. Ha M, Choi J, Park B, Han K. Highly flexible cover window using ultra-thin glass for foldable displays. J Mech Sci Technol 2021;35:661-8.
24. Jo W, Jeong K, Park Y, Lee J, Gap Im S, Kim T. Thermally stable and soft pressure-sensitive adhesive for foldable electronics. Chem Eng J 2023;452:139050.
25. Salmon F, Everaerts A, Campbell C, Pennington B, Erdogan-haug B, Caldwell G. 64-1: modeling the mechanical performance of a foldable display panel bonded by 3M optically clear adhesives. Symp Digest Tech Papers 2017;48:938-41.
26. Han SH, Shin JH, Choi SS. Analytical investigation of multi-layered rollable displays considering nonlinear elastic adhesive interfaces. Sci Rep 2023;13:5697.
27. Kwon Y, Lee S, Kim J, et al. Ultraviolet light blocking optically clear adhesives for foldable displays via highly efficient visible-light curing. Nat Commun 2024;15:2829.
28. Back JH, Kwon Y, Cho H, et al. Visible-light-curable acrylic resins toward UV-light-blocking adhesives for foldable displays. Adv Mater 2023;35:e2204776.
29. Choi JW, Lee JH. Selectively UV-blocking and visibly transparent adhesive films embedded with TiO2/PMMA hybrid nanoparticles for displays. Materials 2020;13:5273.
30. Wu C, Zeng S, Wang Z, et al. Efficient mechanoluminescent elastomers for dual-responsive anticounterfeiting device and stretching/strain sensor with multimode sensibility. Adv Funct Mater 2018;28:1803168.
31. Wang J, Yao K, Cui K, et al. Contact electrification induced multicolor self-recoverable mechanoluminescent elastomer for wearable smart light-emitting devices. Adv Opt Mater 2023;11:2203112.
32. Wang C, Hu H, Peng D, Dong L, Zhu D. Soft devices empowered by mechanoluminescent materials. Soft Sci 2023;3:39.
33. Li L, Cheng L, Yang L, Zhang J. Multistimuli-responsive artificial skin with dual output of photoelectric signals. Macro Mater Eng 2021;306:2100017.
34. Abrahamson JT, Beagi HZ, Salmon F, Campbell CJ. Optically clear adhesives for OLED. In: Pyshkin S, editor. Luminescence - OLED technology and applications. IntechOpen; 2019.
35. Nishimura M, Takebayashi K, Hishinuma M, Yamaguchi H, Murayama A. A 5.5-inch full HD foldable AMOLED display based on neutral-plane splitting concept. J Soc Info Display 2019;27:480-6.
36. Su Y, Li S, Li R, Dagdeviren C. Splitting of neutral mechanical plane of conformal, multilayer piezoelectric mechanical energy harvester. Appl Phys Lett 2015;107:041905.
37. Hishinuma M, Nishimura M, Yamaguchi H, Murayama A. [FLX3-1(Invited)] Analysis of neutral-plane splitting for foldable displays using digital image correlation method. Proc Int Display Workshops 2020;27:898.
38. Lecavelier des Etangs-levallois A, Chen Z, Lesecq M, et al. A converging route towards very high frequency, mechanically flexible, and performance stable integrated electronics. J Appl Phys 2013;113:153701.
39. Shi Y, Rogers JA, Gao C, Huang Y. Multiple neutral axes in bending of a multiple-layer beam with extremely different elastic properties. J Appl Mech 2014;81:114501.
40. Li S, Su Y, Li R. Splitting of the neutral mechanical plane depends on the length of the multi-layer structure of flexible electronics. Proc Math Phys Eng Sci 2016;472:20160087.
41. Wald MJ, Salmon FT, Cosgrove DT, Everaerts AI, inventors; 3M Innovative Properties Co., assignee. Flexible displays having stiff layers for neutral plane adjustment. United States patent US10334723B2. 2016. Available from: https://patents.google.com/patent/US10334723B2/en?oq=US10334723B2. [Last accessed on 30 Jul 2024].
42. Jeong K, Kim D, Ahn D, et al. A hyperelastic adhesive forming multiple neutral planes even at extreme temperatures. Chem Eng J 2024;480:148151.
43. Nam J, Lee S, Han M, Lee H. Improved stack structure of rollable display to prevent delamination and permanent deformation. Int J Precis Eng Manuf 2021;22:671-8.
44. Okumura Y, Ito K. The polyrotaxane gel: a topological gel by figure-of-eight cross-links. Adv Mater 2001;13:485-7.
45. Kato K, Ito K. Dynamic transition between rubber and sliding states attributed to slidable cross-links. Soft Matter 2011;7:8737-40.
46. Fleury G, Schlatter G, Brochon C, et al. Topological polymer networks with sliding cross-link points: the “sliding gels”. relationship between their molecular structure and the viscoelastic as well as the swelling properties. Macromolecules 2007;40:535-43.
47. Du R, Xu Z, Zhu C, et al. A Highly stretchable and self-healing supramolecular elastomer based on sliding crosslinks and hydrogen bonds. Adv Funct Mater 2020;30:1907139.
48. Bin Imran A, Esaki K, Gotoh H, et al. Extremely stretchable thermosensitive hydrogels by introducing slide-ring polyrotaxane cross-linkers and ionic groups into the polymer network. Nat Commun 2014;5:5124.
49. Yi M, Lee T, Han G, et al. Movable cross-linking in adhesives: superior stretching and adhesion properties via a supramolecular sliding effect. ACS Appl Polym Mater 2021;3:2678-86.
50. Watabe T, Otsuka H. Enhancing the reactivity of mechanically responsive units via macromolecular design. Macromolecules 2024;57:425-33.
51. Wang S, Hu Y, Kouznetsova TB, et al. Facile mechanochemical cycloreversion of polymer cross-linkers enhances tear resistance. Science 2023;380:1248-52.
52. Ghanem MA, Basu A, Behrou R, et al. The role of polymer mechanochemistry in responsive materials and additive manufacturing. Nat Rev Mater 2021;6:84-98.
53. Wang S, Beech HK, Bowser BH, et al. Mechanism dictates mechanics: a molecular substituent effect in the macroscopic fracture of a covalent polymer network. J Am Chem Soc 2021;143:3714-8.
54. Beech HK, Wang S, Sen D, et al. Reactivity-guided depercolation processes determine fracture behavior in end-linked polymer networks. ACS Macro Lett 2023;12:1685-91.
55. Zhao C, Gong X, Wang S, Jiang W, Xuan S. Shear stiffening gels for intelligent anti-impact applications. Cell Rep Phys Sci 2020;1:100266.
56. Zhong D, Wu C, Jiang Y, et al. Author correction: high-speed and large-scale intrinsically stretchable integrated circuits. Nature 2024;630:E12.
57. Chun S, Kim DW, Baik S, et al. Conductive and stretchable adhesive electronics with miniaturized octopus-like suckers against dry/wet skin for biosignal monitoring. Adv Funct Mater 2018;28:1805224.
58. Ates HC, Nguyen PQ, Gonzalez-Macia L, et al. End-to-end design of wearable sensors. Nat Rev Mater 2022;7:887-907.
59. Choi S, Kwon S, Kim H, et al. Highly flexible and efficient fabric-based organic light-emitting devices for clothing-shaped wearable displays. Sci Rep 2017;7:6424.
60. Lee S, Kwon JH, Kwon S, Choi KC. A review of flexible OLEDs toward highly durable unusual displays. IEEE Trans Electron Devices 2017;64:1922-31.
61. Song YJ, Kim JW, Cho HE, et al. Fibertronic organic light-emitting diodes toward fully addressable, environmentally robust, wearable displays. ACS Nano 2020;14:1133-40.
62. Rogers JA, Someya T, Huang Y. Materials and mechanics for stretchable electronics. Science 2010;327:1603-7.
64. Kang SH, Jo JW, Lee JM, et al. Full integration of highly stretchable inorganic transistors and circuits within molecular-tailored elastic substrates on a large scale. Nat Commun 2024;15:2814.
65. Shi Y, Zhao J, Zhang B, et al. Freestanding serpentine silicon strips with ultrahigh stretchability over 300% for wearable electronics. Adv Mater 2024;36:e2313603.
66. Wang Y, Han X, Jin L, et al. Excitation threshold reduction techniques for organic semiconductor lasers: a review. Coatings 2023;13:1815.
67. Chen J, Zhang W, Wang L, Yu G. Recent research progress of organic small-molecule semiconductors with high electron mobilities. Adv Mater 2023;35:2210772.
68. Jung D, Ju H, Cho S, Lee T, Hong C, Lee J. Multilayer stretchable electronics with designs enabling a compact lateral form. npj Flex Electron 2024;8:13.
69. Park J, Kim HW, Lim S, et al. Conformal fixation strategies and bioadhesives for soft bioelectronics. Adv Funct Mater 2024;34:2313728.
70. Efstathiou S, Nurumbetov G, Ross A, Li Y, Haddleton DM. Moisture-cured solvent free silylated poly(ether-urea) pressure-sensitive adhesives (PSAs) for use as skin adhesives for application in transdermal drug delivery (TDD). Mater Adv 2024;5:3396-410.
71. Zheng Y, Wu M, Duan M, et al. Skin temperature-triggered switchable adhesive coatings for wearing comfortable epidermal electronics. Chem Eng J 2024;488:150459.
72. Fialho L, Albuquerque J, Pinho AS, et al. Exploring innovative adhesive approaches to manage medical adhesive-related skin injuries (MARSI). Int J Adhes Adhes 2024;130:103636.
73. Guo H, Zhang W, Jia Z, et al. A biodegradable supramolecular adhesive with robust instant wet adhesion for urgent hemostasis and wound repair. Adv Funct Mater 2024;34:2401529.
74. Yuan X, Kong W, Xia P, et al. Implantable wet-adhesive flexible electronics with ultrathin gelatin film. Adv Funct Mater 2024:2404824.
75. Zhou Y, Wang L, Liu Y, et al. Transparent, stretchable, self-healing, and self-adhesive ionogels for flexible multifunctional sensors and encryption systems. Chem Eng J 2024;484:149632.
76. Roslan MF, Shaffiar NM, Khairusshima MKN, Sharifah ISS. Finite element analysis on deformation of stretchable electronic interconnect substrate using polydimethylsiloxanes (PDMS). IOP Conf Ser Mater Sci Eng 2018;290:012022.
77. Shao Y, Tan X, Novitski R, et al. Uniaxial cell stretching device for live-cell imaging of mechanosensitive cellular functions. Rev Sci Instrum 2013;84:114304.
78. Hong JH, Kim S, Lee J, Yoon J, Kim S, Kim Y. 74-1: Invited paper: highly stretchable and shrinkable AMOLED for free deformation. Symp Digest Tech Papers 2023;54:1041-4.
79. Wang CL, Ho ST, Wang WT, et al. 41-3: Invited paper: high resolution stretchable micro-LED displays. Symp Digest Tech Papers 2022;53:521-3.
80. Sluka T. 42-1: Invited paper: high-resolution light-field AR at comparable computing cost to stereo 3D. Symp Digest Tech Papers 2022;53:526-7.
81. Kang J, Luo H, Tang W, et al. 71-2: Enabling processes and designs for tight-pitch micro-LED based stretchable display. Symp Digest Tech Papers 2021;52:1056-9.
82. Khang DY, Jiang H, Huang Y, Rogers JA. A stretchable form of single-crystal silicon for high-performance electronics on rubber substrates. Science 2006;311:208-12.
83. Dana SF, Nguyen D, Kochhar JS, Liu X, Kang L. UV-curable pressure sensitive adhesive films: effects of biocompatible plasticizers on mechanical and adhesion properties. Soft Matter 2013;9:6270-81.
84. Roy A, Manna K, Ray PG, Dhara S, Pal S. β-cyclodextrin-based ultrahigh stretchable, flexible, electro- and pressure-responsive, adhesive, transparent hydrogel as motion sensor. ACS Appl Mater Interfaces 2022;14:17065-80.
85. Mao J, Zhao C, Liu L, et al. Adhesive, transparent, stretchable, and strain-sensitive hydrogel as flexible strain sensor. Compos Commun 2021;25:100733.
86. Han GY, Park JY, Lee TH, Yi MB, Kim HJ. Highly resilient dual-crosslinked hydrogel adhesives based on a dopamine-modified crosslinker. ACS Appl Mater Interfaces 2022;14:36304-14.
87. Yi M, Lee T, Lee S, Kim J, Kim H. Topologically designed cross-linking network for stretchable and recoverable pressure-sensitive adhesives with exceptional softness. Mater Today Chem 2022;26:101141.
88. Campbell CJ, Clapper J, Behling RE, et al. P-198: optically clear adhesives enabling foldable and flexible OLED displays. Symp Digest Tech Papers 2017;48:2009-11.
89. Lee TI, Jo W, Kim W, Kim JH, Paik KW, Kim TS. Direct visualization of cross-sectional strain distribution in flexible devices. ACS Appl Mater Interfaces 2019;11:13416-22.
90. Kim W, Lee I, Yoon Kim D, et al. Controlled multiple neutral planes by low elastic modulus adhesive for flexible organic photovoltaics. Nanotechnology 2017;28:194002.
91. Park Y, Kim J, Ahn D, Yu Y, Lee W, Kwon MS. Biomass-derived optically clear adhesives for foldable displays. ChemSusChem 2024:e202301795.
92. Lee MH, Jang S, Hwang BH, Kwak T, Kim JJ, Yoon S. 53-1: The foldable display architecture technique depending on the wide temperature range and the folding curvature. Symp Digest Tech Papers 2022;53:692-5.
93. Gower MD, Shanks RA. Acrylic acid level and adhesive performance and peel master-curves of acrylic pressure-sensitive adhesives. J Polym Sci B Polym Phys 2006;44:1237-52.
95. Tanaka F, Edwards SF. Viscoelastic properties of physically crosslinked networks. 1. Transient network theory. Macromolecules 1992;25:1516-23.
96. Tanaka F, Edwards S. Viscoelastic properties of physically crosslinked networks: Part 2. Dynamic mechanical moduli. J Non-Newton Fluid Mech 1992;43:273-88.
97. Eckstein A, Suhm J, Friedrich C, et al. Determination of plateau moduli and entanglement molecular weights of isotactic, syndiotactic, and atactic polypropylenes synthesized with metallocene catalysts. Macromolecules 1998;31:1335-40.
98. Dobrynin AV, Tian Y, Jacobs M, et al. Forensics of polymer networks. Nat Mater 2023;22:1394-400.
99. Malvern Instrument. A basic introduction to rheology. Available from: https://cdn.technologynetworks.com/TN/Resources/PDF/WP160620BasicIntroRheology.pdf. [Last accessed on 26 Jul 2024].
100. Lee TH, Kim JS, Lee JH, Kim HJ. Pressure-sensitive adhesives for flexible display applications. In: Vargas-Bernal R, He P, Zhang S, editors. Hybrid nanomaterials - flexible electronics materials. IntechOpen; 2019.
102. Yuk H, Zhang T, Lin S, Parada GA, Zhao X. Tough bonding of hydrogels to diverse non-porous surfaces. Nat Mater 2016;15:190-6.
103. Krenceski MA, Johnson JF, Temin SC. Chemical and physical factors affecting performance of pressure-sensitive adhesives. J Macromol Sci Part C Polym Rev 1986;26:143-82.
104. Kinloch AJ, Williams JG. Chapter 8 - The mechanics of peel tests. Adhes Sci Eng 2002;1:273-301.
105. Czech Z. Crosslinking of pressure sensitive adhesive based on water-borne acrylate. Polym Int 2003;52:347-57.
106. Lee J, Lee T, Shim K, et al. Effect of crosslinking density on adhesion performance and flexibility properties of acrylic pressure sensitive adhesives for flexible display applications. Int J Adhes Adhes 2017;74:137-43.
107. Kinloch AJ. Adhesion and adhesives: science and technology. Springer Science & Business Media; 1987.
108. Pocius AV. Adhesion and adhesives technology: an introduction. 3rd ed. Carl Hanser Verlag GmbH & Company KG; 2012.
110. Won Y, Shin HS, Jo M, Lim YJ, Manda R, Lee SH. An electrically switchable dye-doped liquid crystal polarizer for organic light emitting-diode displays. J Mol Liquids 2021;333:115922.
111. Vaenkatesan V, Wegh R, Teunissen J, Lub J, Bastiaansen C, Broer D. Improving the brightness and daylight contrast of organic light-emitting diodes. Adv Funct Mater 2005;15:138-42.
112. Hack M, Ma R, inventors; Universal Display Corporation., assignee. Integrated circular polarizer and permeation barrier for flexible OLEDs. United States patent US20180047945A1. 2016. Available from: https://patents.google.com/patent/US20180047945A1/en?oq=US20180047945A1. [Last accessed on 30 Jul 2024].
113. Antosik AK, Bednarczyk P, Czech Z. Aging of silicone pressure-sensitive adhesives. Polym Bull 2018;75:1141-7.
114. Schwotzer W, Käser F, Roduit B, Ferrand D. Simulating the aging of adhesives. Adhesives & Sealants Industry, 2008. Available from: https://www.adhesivesmag.com/articles/87025-simulating-the-aging-of-adhesives. [Last accessed on 26 Jul 2024].
115. Rudawska A, Abdel Wahab M, Müller M. Effect of ageing process on mechanical properties of adhesive tubular butt joints in aqueous environment. Int J Adhes Adhes 2020;96:102466.
116. ASTM D3632-98. Standard test method for accelerated aging of adhesive joints by the oxygen-pressure method. Available from: https://www.astm.org/d3632-98.html. [Last accessed on 30 Jul 2024].
117. ASTM D3611-06. Standard practice for accelerated aging of pressure-sensitive tapes. Available from: https://www.astm.org/d3611-06.html. [Last accessed on 30 Jul 2024].
118. Chiang C, Winscom C, Bull S, Monkman A. Mechanical modeling of flexible OLED devices. Org Electron 2009;10:1268-74.
119. Ma BS, Jo W, Kim W, Kim TS. Mechanical modeling of rollable OLED display apparatus considering spring component. J Microelect Pack Soc 2020;27:19-26.
120. Li S, Liu X, Li R, Su Y. Shear deformation dominates in the soft adhesive layers of the laminated structure of flexible electronics. Int J Solids Struct 2017;110-1:305-14.
121. Jang SJ, Myung NJ, Hwang BH, Woo SW, Kwak TH, inventors; LG Display, assignee. Foldable display device. United States patent US10198038B2. 2019. Available from: https://patents.google.com/patent/US10198038B2/en?oq=US10198038B2. [Last accessed on 30 Jul 2024].
122. Jia Y, Liu Z, Wu D, Chen J, Meng H. Mechanical simulation of foldable AMOLED panel with a module structure. Org Electron 2019;65:185-92.
123. Niu L, Ding J, Liu W. Analysis on the mechanical behavior of flexible screens. Materials 2022;15:2829.
124. Chen ZP, Li SS, Wang S, Lee BG, Yuan Z, Yu X. P-119: modeling of mechanical effects in flexible display. Symp Digest Tech Papers 2020;51:1818-21.
125. Zhang Y, Wang S, Dong F, et al. Mechanical behavior and constitutive model characterization of optically clear adhesive in flexible devices. Micromachines 2022;13:301.
126. Lee JH, Myung MH, Baek MJ, Kim H, Lee DW. Effects of monomer functionality on physical properties of 2-ethylhexyl acrylate based stretchable pressure sensitive adhesives. Polym Test 2019;76:305-11.
127. Lee JH, Park J, Myung MH, Baek M, Kim H, Lee DW. Stretchable and recoverable acrylate-based pressure sensitive adhesives with high adhesion performance, optical clarity, and metal corrosion resistance. Chem Eng J 2021;406:126800.
128. Lim D, Baek M, Kim H, Baig C, Lee DW. Carboxyethyl acrylate incorporated optically clear adhesives with outstanding adhesion strength and immediate strain recoverability for stretchable electronics. Chem Eng J 2022;437:135390.
129. Lee K, Tiu BDB, Martchenko V, et al. A modular strategy for functional pressure sensitive adhesives. ACS Appl Mater Interfaces 2021;13:3161-5.
130. Seok WC, Leem JT, Song HJ. Acrylic pressure-sensitive adhesives based on ethylene glycol acrylate for flexible display application: Highly elastic and recoverable properties. Polym Test 2022;108:107491.
131. Seok WC, Park JH, Song HJ. Effect of silane acrylate on the surface properties, adhesive performance, and rheological behavior of acrylic pressure sensitive adhesives for flexible displays. J Ind Eng Chem 2022;111:98-110.
132. Seok WC, Leem JT, Song HJ. The effect of silane acrylate containing ethylene glycol chains on the adhesive performance and viscoelastic behavior of acrylic pressure-sensitive adhesives for flexible displays. Polymers 2023;15:3601.
133. Moussa K, Decker C. Light-induced polymerization of new highly reactive acrylic monomers. J Polym Sci A Polym Chem 1993;31:2197-203.
134. Lee TY, Roper TM, Jönsson ES, Guymon CA, Hoyle CE. Influence of hydrogen bonding on photopolymerization rate of hydroxyalkyl acrylates. Macromolecules 2004;37:3659-65.
135. Vleeschouwer F, Van Speybroeck V, Waroquier M, Geerlings P, De Proft F. Electrophilicity and nucleophilicity index for radicals. Org Lett 2007;9:2721-4.
136. Taghizadeh SM, Ghasemi D. Rheological and adhesion properties of acrylic pressure-sensitive adhesives. J Appl Polymer Sci 2011;120:411-8.
137. Sanai Y, Kagami S, Kubota K. Cross-linking photopolymerization of monoacrylate initiated by benzophenone. J Polym Sci Part A Polym Chem 2018;56:1545-53.
138. Novikov V, Rössler E. Correlation between glass transition temperature and molecular mass in non-polymeric and polymer glass formers. Polymer 2013;54:6987-91.
139. Hintermeyer J, Herrmann A, Kahlau R, Goiceanu C, Rössler EA. Molecular weight dependence of glassy dynamics in linear polymers revisited. Macromolecules 2008;41:9335-44.
140. Zhang P, Zhou W, He Y, et al. Stretchable heterogeneous polymer networks of high adhesion and low hysteresis. ACS Appl Mater Interfaces 2022;14:49264-73.
141. Moon H, Jeong K, Kwak MJ, Choi SQ, Im SG. Solvent-free deposition of ultrathin copolymer films with tunable viscoelasticity for application to pressure-sensitive adhesives. ACS Appl Mater Interfaces 2018;10:32668-77.
142. Kim J, Hwang J, Baek D, Kim H, Kim Y. Characterization and flexibility properties of UV LED cured acrylic pressure-sensitive adhesives for flexible displays. J Mater Res Technol 2021;10:1176-83.
143. Kim J, Kim H, Kim Y. Flexibility properties of pressure-sensitive adhesive with different pattern of crosslinking density for electronic displays. J Mater Res Technol 2021;15:1408-15.
144. Lee J, Kim K, Kim H, Kim Y. Ultraviolet-patterned acrylic pressure-sensitive adhesives for flexible displays. Polymer 2021;237:124324.
145. Back JH, Kim JS, Kim Y, Kim HJ. Heterogeneous acrylic resins with bicontinuous nanodomains as low-modulus flexible adhesives. Small 2024:e2403497.
146. Bonnotte T, Paul S, Araque M, Wojcieszak R, Dumeignil F, Katryniok B. Dehydration of lactic acid: the state of the art. ChemBioEng Rev 2018;5:34-56.
147. Haque FM, Ishibashi JSA, Lidston CAL, et al. Defining the macromolecules of tomorrow through synergistic sustainable polymer research. Chem Rev 2022;122:6322-73.
148. Gabriel VA, Dubé MA. Toward a fully biobased pressure-sensitive adhesive. Ind Eng Chem Res 2023;62:478-88.
149. Droesbeke MA, Aksakal R, Simula A, Asua JM, Du Prez FE. Biobased acrylic pressure-sensitive adhesives. Prog Polym Sci 2021;117:101396.
150. Chen TTD, Carrodeguas LP, Sulley GS, Gregory GL, Williams CK. Bio-based and degradable block polyester pressure-sensitive adhesives. Angew Chem Int Ed Engl 2020;132:23656-61.
151. Albanese KR, Okayama Y, Morris PT, et al. Building tunable degradation into high-performance poly(acrylate) pressure-sensitive adhesives. ACS Macro Lett 2023;12:787-93.
152. Machado TO, Stubbs CJ, Chiaradia V, et al. A renewably sourced, circular photopolymer resin for additive manufacturing. Nature 2024;629:1069-74.
153. Castagnet T, Aguirre G, Asua JM, Billon L. Bioinspired enzymatic synthesis of terpenoid-based (meth)acrylic monomers: a solvent-, metal-, amino-, and halogen-free approach. ACS Sustainable Chem Eng 2020;8:7503-12.
154. Hermens JGH, Jensma A, Feringa BL. Highly efficient biobased synthesis of acrylic acid. Angew Chem Int Ed Engl 2022;61:e202112618.
155. Droesbeke MA, Du Prez FE. Sustainable synthesis of renewable terpenoid-based (meth)acrylates using the CHEM21 green metrics toolkit. ACS Sustainable Chem Eng 2019;7:11633-9.
156. Obermeier F, Hense D, Stockmann PN, Strube OI. Syntheses and polymerization of monoterpene-based (meth)acrylates: IBO(M)A as a relevant monomer for industrial applications. Green Chem 2024;26:4387-416.
157. Jarach N, Dodiuk H. Debondable, recyclable and/or biodegradable naturally-based adhesives. In: Dunky M, Mittal K, editors. Biobased adhesives. Wiley; 2023. pp. 427-61.
158. Veith C, Diot-néant F, Miller SA, Allais F. Synthesis and polymerization of bio-based acrylates: a review. Polym Chem 2020;11:7452-70.
159. Nasiri M, Saxon DJ, Reineke TM. Enhanced mechanical and adhesion properties in sustainable triblock copolymers via non-covalent interactions. Macromolecules 2018;51:2456-65.
160. Badía A, Agirre A, Barandiaran MJ, Leiza JR. Removable biobased waterborne pressure-sensitive adhesives containing mixtures of isosorbide methacrylate monomers. Biomacromolecules 2020;21:4522-31.
161. Gallagher JJ, Hillmyer MA, Reineke TM. Acrylic triblock copolymers incorporating isosorbide for pressure sensitive adhesives. ACS Sustainable Chem Eng 2016;4:3379-87.
162. Heo J, Kang T, Jang SG, et al. Improved performance of protected catecholic polysiloxanes for bioinspired wet adhesion to surface oxides. J Am Chem Soc 2012;134:20139-45.
163. Li Y, Sun XS. Synthesis and characterization of acrylic polyols and polymers from soybean oils for pressure-sensitive adhesives. RSC Adv 2015;5:44009-17.
164. Fouilloux H, Qiang W, Robert C, Placet V, Thomas CM. Multicatalytic transformation of (meth)acrylic acids: a one-pot approach to biobased poly(meth)acrylates. Angew Chem Int Ed Engl 2021;60:19374-82.
165. Abraham TW, Allen E, Hahn JJ, Tsobanakis P, Bohnert EC, Frank CL, inventors; Cargill Inc., assignee. Recovery of 3-hydroxypropionic acid. United States patent US10442748B2. 2019. Available from: https://patents.google.com/patent/US10442748B2/en?oq=US10442748B2. [Last accessed on 30 Jul 2024].
166. Abubakar UC, Bansod Y, Forster L, Spallina V, D’agostino C. Conversion of glycerol to acrylic acid: a review of strategies, recent developments and prospects. React Chem Eng 2023;8:1819-38.
167. Bio-acrylic acid market by type (methyl acrylate, ethyl acrylate, butyl acrylate, elastomers, 2-ethylhexyl acrylate, superabsorbent polymers), application and tegion (north america, europe, the asia pacific, and the rest of the world.) - global forecast to 2027. Available from: https://www.marketsandmarkets.com/Market-Reports/bio-acrylic-acid-market-144896040.html. [Last accessed on 26 Jul 2024].
168. Atkinson RL, Monaghan OR, Elsmore MT, et al. RAFT polymerisation of renewable terpene (meth)acrylates and the convergent synthesis of methacrylate-acrylate-methacrylate triblock copolymers. Polym Chem 2021;12:3177-89.
169. Zhang L, Cao Y, Wang L, Shao L, Bai Y. Polyacrylate emulsion containing IBOMA for removable pressure sensitive adhesives. J Appl Polym Sci 2016;133:42886.
170. Droesbeke MA, Simula A, Asua JM, Du Prez FE. Biosourced terpenoids for the development of sustainable acrylic pressure-sensitive adhesives via emulsion polymerisation. Green Chem 2020;22:4561-9.
171. Baek SS, Hwang SH. Preparation of biomass-based transparent pressure sensitive adhesives for optically clear adhesive and their adhesion performance. Eur Polym J 2017;92:97-104.
172. Baek SS, Jang SH, Hwang SH. Construction and adhesion performance of biomass tetrahydro-geraniol-based sustainable/transparent pressure sensitive adhesives. J Ind Eng Chem 2017;53:429-34.
173. Badía A, Santos JI, Agirre A, Barandiaran MJ, Leiza JR. UV-tunable biobased pressure-sensitive adhesives containing piperonyl methacrylate. ACS Sustainable Chem Eng 2019;7:19122-30.
174. Agirre A, Nase J, Degrandi E, Creton C, Asua JM. Improving adhesion of acrylic waterborne PSAs to low surface energy materials: introduction of stearyl acrylate. J Polym Sci A Polym Chem 2010;48:5030-9.
175. Iso T, Ninomiya T, Kagami S, Kubota K, Sanai Y. Environmentally-friendly UV-curable coatings utilizing bio-based polyester acrylates. Prog Org Coat 2023;175:107356.
176. Hub L, Koll J, Held M, Radjabian M, Abetz V. Amphiphilic block copolymers via blue-light-induced iniferter RAFT ab initio emulsion polymerization in water–alcoholic media. Macromolecules 2024;57:2273-86.
177. Lovell PA, Schork FJ. Fundamentals of emulsion polymerization. Biomacromolecules 2020;21:4396-441.
178. Noppalit S, Simula A, Billon L, Asua JM. On the nitroxide mediated polymerization of methacrylates derived from bio-sourced terpenes in miniemulsion, a step towards sustainable products. Polym Chem 2020;11:1151-60.
179. Yan Y, Wu J, Wang Y, et al. Strong and UV-responsive plant oil-based ethanol aqueous adhesives fabricated via surfactant-free RAFT-mediated emulsion polymerization. ACS Sustainable Chem Eng 2021;9:13695-702.
180. Lee Y, Kwon Y, Kim Y, et al. A water-soluble organic photocatalyst discovered for highly efficient additive-free visible-light-driven grafting of polymers from proteins at ambient and aqueous environments. Adv Mater 2022;34:e2108446.
181. Niu J, Page ZA, Dolinski ND, et al. Rapid visible light-mediated controlled aqueous polymerization with in situ monitoring. ACS Macro Lett 2017;6:1109-13.
182. Chung KY, Page ZA. Boron-methylated dipyrromethene as a green light activated type i photoinitiator for rapid radical polymerizations. J Am Chem Soc 2023;145:17912-8.
183. Tucker BS, Coughlin ML, Figg CA, Sumerlin BS. Grafting-from proteins using metal-free PET-RAFT polymerizations under mild visible-light irradiation. ACS Macro Lett 2017;6:452-7.
184. Borjigin T, Schmitt M, Giacoletto N, et al. The blue-LED-sensitive naphthoquinone-imidazolyl derivatives as type II photoinitiators of free radical photopolymerization. Adv Mater Interfaces 2023;10:2202352.
185. Lee Y, Boyer C, Kwon MS. Photocontrolled RAFT polymerization: past, present, and future. Chem Soc Rev 2023;52:3035-97.
186. Jeon W, Kwon Y, Kwon MS. Highly efficient dual photoredox/copper catalyzed atom transfer radical polymerization achieved through mechanism-driven photocatalyst design. Nat Commun 2024;15:5160.
187. Corrigan N, Yeow J, Judzewitsch P, Xu J, Boyer C. Seeing the light: advancing materials chemistry through photopolymerization. Angew Chem Int Ed Engl 2019;58:5170-89.
188. Fors BP, Hawker CJ. Control of a living radical polymerization of methacrylates by light. Angew Chem Int Ed Engl 2012;51:8850-3.
189. Singh VK, Yu C, Badgujar S, et al. Highly efficient organic photocatalysts discovered via a computer-aided-design strategy for visible-light-driven atom transfer radical polymerization. Nat Catal 2018;1:794-804.
190. Kim D, Kim H, Jeon W, et al. Ultraviolet light debondable optically clear adhesives for flexible displays through efficient visible-light curing. Adv Mater 2024;36:e2309891.
191. Song Y, He J, Zhang Y, Gilsdorf RA, Chen EYX. Recyclable cyclic bio-based acrylic polymer via pairwise monomer enchainment by a trifunctional Lewis pair. Nat Chem 2023;15:366-76.
192. Jehanno C, Alty JW, Roosen M, et al. Critical advances and future opportunities in upcycling commodity polymers. Nature 2022;603:803-14.
193. Deacy AC, Gregory GL, Sulley GS, Chen TTD, Williams CK. Sequence control from mixtures: switchable polymerization catalysis and future materials applications. J Am Chem Soc 2021;143:10021-40.
195. Wang ZH, Liu BW, Zeng FR, et al. Fully recyclable multifunctional adhesive with high durability, transparency, flame retardancy, and harsh-environment resistance. Sci Adv 2022;8:eadd8527.
196. Hwang J, Lim D, Lee G, et al. Ambient air-operated thermo-switchable adhesion of N-isopropylacrylamide-incorporated pressure sensitive adhesives. Mater Horiz 2023;10:2013-23.
197. Mulcahy KR, Kilpatrick AFR, Harper GDJ, Walton A, Abbott AP. Debondable adhesives and their use in recycling. Green Chem 2022;24:36-61.
198. Beharaj A, Ekladious I, Grinstaff MW. Poly(alkyl glycidate carbonate)s as degradable pressure-sensitive adhesives. Angew Chem 2019;131:1421-5.
199. Beharaj A, McCaslin EZ, Blessing WA, Grinstaff MW. Sustainable polycarbonate adhesives for dry and aqueous conditions with thermoresponsive properties. Nat Commun 2019;10:5478.
200. Shieh P, Zhang W, Husted KEL, et al. Cleavable comonomers enable degradable, recyclable thermoset plastics. Nature 2020;583:542-7.
201. Pesenti T, Nicolas J. 100th Anniversary of Macromolecular Science Viewpoint: degradable polymers from radical ring-opening polymerization: latest advances, new directions, and ongoing challenges. ACS Macro Lett 2020;9:1812-35.
202. Kim HJ, Jin K, Shim J, Dean W, Hillmyer MA, Ellison CJ. Sustainable triblock copolymers as tunable and degradable pressure sensitive adhesives. ACS Sustainable Chem Eng 2020;8:12036-44.
203. Bakar R, Hepburn KS, Keddie JL, Roth PJ. Degradable, ultraviolet-crosslinked pressure-sensitive adhesives made from thioester-functional acrylate copolymers. Angew Chem Int Ed Engl 2023;62:e202307009.
204. Bakar RA, Keddie JL, Roth PJ. New chemistries for degradable pressure-sensitive adhesive networks. Chempluschem 2024;89:e202400034.
205. Albanese KR, Morris PT, Read de Alaniz J, Bates CM, Hawker CJ. Controlled-radical polymerization of α-lipoic acid: a general route to degradable vinyl copolymers. J Am Chem Soc 2023;145:22728-34.
206. Korpusik AB, Adili A, Bhatt K, Anatot JE, Seidel D, Sumerlin BS. Degradation of polyacrylates by one-pot sequential dehydrodecarboxylation and ozonolysis. J Am Chem Soc 2023;145:10480-5.
207. Abel BA, Snyder RL, Coates GW. Chemically recyclable thermoplastics from reversible-deactivation polymerization of cyclic acetals. Science 2021;373:783-9.
208. Bandl C, Kern W, Schlögl S. Adhesives for “debonding-on-demand”: triggered release mechanisms and typical applications. Int J Adhes Adhes 2020;99:102585.
209. Lundberg DJ, Ko K, Kilgallon LJ, Johnson JA. Defining reactivity-deconstructability relationships for copolymerizations involving cleavable comonomer additives. ACS Macro Lett 2024;13:521-7.
Cite This Article

How to Cite
Park, Y.; Kim J.; Kim D.; Lee S.; Hwang D.; Kwon M. S. Towards the optimal design of optically clear adhesives for flexible display. Soft Sci. 2024, 4, 28. http://dx.doi.org/10.20517/ss.2024.22
Download Citation
Export Citation File:
Type of Import
Tips on Downloading Citation
Citation Manager File Format
Type of Import
Direct Import: When the Direct Import option is selected (the default state), a dialogue box will give you the option to Save or Open the downloaded citation data. Choosing Open will either launch your citation manager or give you a choice of applications with which to use the metadata. The Save option saves the file locally for later use.
Indirect Import: When the Indirect Import option is selected, the metadata is displayed and may be copied and pasted as needed.
About This Article
Special Issue
Copyright
Data & Comments
Data
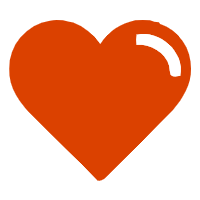
Comments
Comments must be written in English. Spam, offensive content, impersonation, and private information will not be permitted. If any comment is reported and identified as inappropriate content by OAE staff, the comment will be removed without notice. If you have any queries or need any help, please contact us at support@oaepublish.com.