Recent advances of two-dimensional molybdenum oxides
Abstract
Over two decades have passed since the successful exfoliation of graphene, which initiated the exploration of two-dimensional (2D) materials. Since then, this vibrant group has expanded to encompass a variety of new materials. Among these, molybdenum (Mo)-based oxides with 2D structures have attracted growing interest. Due to their remarkable properties, 2D Mo-based oxides have ensured their prominent position in cutting-edge scientific domains, including energy applications, catalysis and electronic devices. This review systematically examines recent advancements in the synthesis, structural regulation, and applications of 2D Mo-based oxides. Firstly, a detailed overview of various synthesis techniques is given, including but not limited to hydrothermal methods, physical vapor deposition, and chemical vapor deposition, enabling the production of high-quality 2D Mo-based oxides. Subsequently, strategies are presented for structural regulation through doping, interface engineering, and interlayer tuning. Finally, recent application developments in energy conversion and storage, catalysis, sensing, and optoelectronic devices are highlighted. Furthermore, an outlook on potential future trends is provided at the conclusion of the review, aiming to advance the practical deployment of 2D Mo-based oxides in emerging technologies.
Keywords
INTRODUCTION
With the ongoing advancement of research efforts, two-dimensional (2D) materials have achieved remarkable progress and become a highlight in the field of materials science. Graphene, transition metal chalcogenides, black phosphorus, hexagonal boron nitride (h-BN), and MXenes are widely acknowledged as representative 2D materials, each exhibiting unique physicochemical properties that have revolutionized fields such as electronics, catalysis, and energy storage. These materials have established high standards for low-dimensional systems due to their exceptional electronic, optical, and mechanical properties.
Notably, the exploration of 2D materials is not limited to these well-studied systems. Metal oxides, a class of materials with diverse compositions and functionalities, have also garnered significant attention in recent years. Metal oxides, including transition metal oxides and rare earth metal oxides, are traditionally known for their stability, tunable electronic properties, and redox activity[1]. These properties make them indispensable in applications ranging from catalysis to energy storage. While bulk and nanoparticle forms of metal oxides have been extensively investigated, their 2D counterparts offer distinct advantages, such as enhanced specific surface area, improved charge transport properties, and the ability to precisely engineer electronic properties through compositional and structural regulation[2].
Despite these advantages, the development of 2D metal oxides faces several challenges. For instance, the synthesizing of high-quality, large-area 2D metal oxides with precise control over thickness and composition remains a significant hurdle. Additionally, the fundamental understanding of 2D metal oxide structure-property relationships, particularly in comparison to their bulk counterparts, is still incomplete. These gaps hinder the full exploitation of their potential in practical applications.
Among the emerging 2D metal oxides, molybdenum (Mo)-based oxides have recently emerged as a promising subcategory. These materials combine the inherent advantages of metal oxides with the unique characteristics of 2D structures, such as tunable bandgaps, robust redox activity, and distinctive electrical and optical properties. These attributes position 2D Mo-based oxides as potential candidates for addressing pressing global challenges, including energy scarcity and environmental contamination. For instance, high surface area and active sites make them ideal for catalytic applications, while their tunable electronic properties are advantageous for energy storage and optoelectronic devices.
However, despite their potential, research on 2D Mo-based oxides is still in its early stages. Several critical challenges remain unresolved, including the development of effective synthesis methods for producing high-quality 2D Mo-based oxides, the precise tuning of their electronic and optical properties to meet specific application requirements, and the identification of key factors governing their performance in energy storage and catalytic applications. Addressing these questions is essential for unlocking the full potential of 2D Mo-based oxides and advancing their practical applications.
In this context, this review aims to provide a comprehensive overview of the latest research developments in 2D Mo-based oxides. The article will systematically discuss the various synthesis methods, strategies for structural regulation, and applications in fields such as catalysis, energy storage, and optoelectronics [Figure 1]. Furthermore, we will highlight the challenges and future directions for 2D Mo-based oxides, offering insights into their potential to drive innovations in materials science and technology. By addressing the existing research gaps and providing a roadmap for future research, this review aims to inspire further exploration and accelerate the development of 2D Mo-based oxides for practical applications.
STRUCTURE AND PROPERTIES OF Mo-BASED OXIDES
Two-dimensional Mo-based oxides can be classified into two categories based on their structure: layered and non-layered. Molybdenum trioxide (MoO3) belongs to the layered structures, while molybdenum dioxide (MoO2) is classified as the non-layered structures. Layered structures consist of multiple atomic planes stacked together, with layers weakly interacting via van der Waals forces while being strongly bonded through covalent interactions within the plane. These materials can be reduced to atomic thicknesses without compromising their crystalline quality. In contrast, non-layered structures tend to grow laterally while being confined vertically, leading to some exposed Mo or oxygen atoms at the edges that are not fully bonded, thus creating unsaturated dangling bonds[3]. These structural differences lead to significant variations in the physical and chemical properties of the materials, thereby influencing their applicability. Layered structures are noted for their exceptional mechanical robustness and optical performance, rendering them particularly advantageous for integration into electronic and optoelectronic devices. Meanwhile, non-layered structures are primarily exploited for their catalytic activity and potential in energy storage systems.
MoO3
MoO3 is an important transition metal oxide that naturally occurs in the form of molybdenite and is generally observed as being white or colorless. Its crystalline forms include α-MoO3, β-MoO3, ε-MoO3, and h-MoO3[4], with the thermodynamically stable orthorhombic phase (α-MoO3) being the most widely studied and utilized. The α-MoO3 exhibits a unique 2D layered structure, as illustrated in Figure 2A and B. In
Figure 2. (A) MoO6 octahedra as found in the thermodynamically stable α-MoO3 composed of molybdenum and oxygen atoms. (B) Thermodynamically stable orthorhombic α-MoO3 with layered structure held together by van der Waals’ forces. (C) Crystal structure of monoclinic MoO2. (D) MoO6 distorted octahedra highlighting the bond ordering. (A and B) reprinted with permission[5]. Copyright 2017, Wiley-VCH. (C and D) reprinted with permission[18]. Copyright 2010, American Chemical Society.
As an n-type semiconductor, MoO3 is characterized by a wide bandgap, high electron affinity, and high ionization energy. When present in reduced oxidation states, such as MoO3-x, defect states can form within the bandgap, leading to a color change from transparent to yellow or even blue-gray. This photochromic property renders it suitable for applications in photochromic devices[6].
Recent studies have highlighted 2D α-MoO3 as a natural material for hyperbolic phonon polaritons (PhPs). α-MoO3 demonstrates significant in-plane optical anisotropy, which allows it to support hyperbolic PhPs in the mid-infrared region. These properties result in strong spatial confinement effects and lower losses, positioning α-MoO3 as an ideal platform for investigating new optical phenomena[7-9]. Furthermore, the tunable localized surface plasmon resonance (LSPR) effect exhibited by 2D MoO3, coupled with its active performance in twistronics and near-field radiative heat transfer, highlights its substantial potential for a wide range of applications[10-16].
MoO2
MoO2 adopts a monoclinic crystal structure (space group P21/c), which bears similarities to the rutile structure. Rutile, which belongs to the tetragonal system, is characterized by a metal center coordinated with six oxygen atoms, forming an octahedral environment. Each oxygen atom is bonded to three metal atoms at the vertices of an equilateral triangle, thereby maintaining a uniform metal-metal bond length[17].
In contrast, MoO2 exhibits a distorted crystal structure, where MoO6 octahedra are linked by shared edges along the [001] direction [Figure 2C]. This distortion leads to an alternation in Mo-Mo bond lengths along the rutile c-axis, with values of 2.51 and 3.02 Å. Consequently, two distinct oxygen coordination environments are created, each with three different M-O bond lengths [Figure 2D][18].
The differing crystal structures of MoO2 and MoO3 give rise to variations in the orbitals occupied by valence electrons, which in turn affects their electronic properties. MoO3 is an n-type semiconductor due to its electronic structure, whereas MoO2 exhibits metallic conductivity[19]. Despite these differences, phase transitions between MoO2 and MoO3 can occur through electron migration, such as the conversion of MoO2 into MoO3.
SYNTHESIS METHODS
The novel properties of 2D Mo-based oxides and their potential applications in numerous fields have drawn significant attention, driving rapid advancements in synthetic strategies. Obtaining stable and high-quality 2D films or nanosheets remains challenging. Generally, synthesis methods can be categorized into top-down and bottom-up approaches[20].
Top-down strategies
Top-down strategies begin at the macroscopic level and involve the use of physical or chemical means to exfoliate monolayer or few-layer nanosheets from bulk materials. Common 2D materials exfoliation techniques encompass mechanical exfoliation, liquid-phase exfoliation, chemical exfoliation, and electrochemical exfoliation. For 2D Mo-based oxides, this section will focus on two prominent exfoliation methods that have garnered significant attention in recent years: liquid-phase exfoliation and supercritical CO2-assisted exfoliation. Table 1 summarizes the advantages and limitations of these various exfoliation techniques. While mechanical exfoliation is often the most straightforward and efficient method to obtain layered 2D materials, the lack of dimensional control renders it unsuitable for large-scale synthesis.
Comparison of various exfoliation methods for 2D Mo-based Oxides
Technique | Advantages | Limitations |
Mechanical exfoliation | High-quality, defect-free monolayers Simple and cost-effective No chemical contamination | Low yield and poor thickness control Not scalable |
Liquid-phase exfoliation | Higher yield than mechanical exfoliation Scalable and cost-effective Customizable nanosheet properties | Limited size/thickness control Potential defects from sonication Requires solvent removal |
Supercritical CO2-assisted exfoliation | Suitable for amorphous nanosheets Environmentally friendly | Requires specialized equipment, increasing cost and complexity Not suitable for all 2D materials |
Compared to traditional mechanical exfoliation, liquid-phase exfoliation offers advantages in terms of yield and the elimination of repetitive operations. The process of liquid-phase exfoliation involves several steps, including mechanical grinding, ultrasonic treatment, and centrifugal separation. These steps are designed to disrupt the interlayer van der Waals forces, thereby facilitating the exfoliation of large-area layered materials into individual or few-layer nanosheets. Furthermore, by adjusting the type of exfoliating solvent and processing conditions, such as temperature and duration, the performance of the nanosheets can be further optimized.
Kuriakose et al. introduced the liquid-phase exfoliation process for 2D MoO3 nanosheets[21]. High-purity MoO3 powder was initially mixed and ground with acetonitrile. The resulting mixture was then dispersed in an equal volume of ethanol and deionized water. This dispersion was subjected to ultrasonication in an ice bath for 2 h, followed by high-speed centrifugation. The supernatant was collected for subsequent device fabrication. This process not only achieved the uniform dispersion of MoO3 nanosheets but also enabled customization of the nanosheet properties by adjusting the concentration of MoO3 in the solution according to specific application requirements.
The liquid-phase exfoliation method is cost-effective and suitable for large-scale production. However, traditional liquid-phase exfoliation techniques still have certain limitations, and practical applications require overcoming challenges related to finer control over size and thickness. To address these limitations, supplementary methods are employed alongside traditional liquid-phase exfoliation. For example, Wei et al. synthesized 2D MoO3-x nanosheets in 2023 using a microwave-assisted exfoliation technique [Figure 3A][22]. Figure 3B reveals that these nanosheets have an average thickness of approximately 2.8 nm. Atomic Force Microscopy (AFM) analysis indicated an average lateral dimension of 2 μm, resulting in an aspect ratio of about 670 [Figure 3C]. This aspect ratio surpasses that of previously reported 2D MoO3 materials produced by other exfoliation techniques. Meng et al. successfully exfoliated monolayer α-MoO3 using a liquid-phase ultrasonic irradiation method[23]. AFM confirmed the thickness of α-MoO3 crystals (~1.48 nm), which is consistent with the previously reported thickness of monolayer α-MoO3 (~1.4 nm). The results indicate that after ultrasonic and hydrothermal treatments, the van der Waals forces between MoO3 layers were disrupted, leading to the successful production of monolayer MoO3.
Figure 3. (A) Synthesis of MoO3-x via microwave-assisted exfoliation technique. (B) Thickness measurement of exfoliated 2D MoO3-x nanosheets. (C) AFM image of 2D MoO3-x nanosheets. All panels reprinted with permission[22]. Copyright 2023, Elsevier.
Two-dimensional amorphous materials possess unique mechanical properties, and their electrical and optical properties differ from those of crystalline materials. Supercritical CO2-assisted exfoliation can produce 2D amorphous nanosheets, leveraging the special properties of CO2 under supercritical conditions. When carbon dioxide is pressurized and heated beyond its critical point, it exhibits both the good diffusivity of gas and the high solvency of liquid. For example, Wu et al. used SC-CO2 to prepare amorphous MoO3 nanosheets. The amorphous structure of am-MoO3 was confirmed by a series of characterization methods[24].
Bottom-up strategies
Bottom-up strategies for synthesizing 2D materials involve constructing these materials from the atomic or molecular level, ultimately forming macroscopic films or nanostructures. Typically, these methods can be divided into liquid-phase and vapor-phase approaches.
Hydrothermal method
Hydrothermal synthesis is a commonly used method for preparing MoO3 nanostructures with specific morphologies by controlling the synthesis conditions. This approach is favored for its simplicity, low cost, and suitability for the fabrication of composite materials. However, because the process takes place under high-temperature and high-pressure conditions, factors such as temperature, pressure, pH, and reaction time are critical in determining the final product. Even minor variations can lead to changes in the size and morphology of the product, posing a challenge to the precise control of the dimensions of 2D materials.
The general process for the hydrothermal synthesis of 2D MoO3 involves the following steps: Initially, a Mo precursor is dissolved in deionized water. An appropriate acid is then added, and the mixture is stirred. Subsequently, the solution is transferred to a stainless-steel autoclave reactor lined with Teflon and subjected to a hydrothermal reaction in a high-temperature oven. The resulting white powder is filtered using deionized water and ethanol, and then dried at a specified temperature. Depending on the desired control strategy and application, additional post-processing steps beyond thermal annealing can be employed, such as chemical reduction or ion exchange, to further refine the structural properties of the 2D materials. Research on the hydrothermal synthesis of 2D MoO3 has utilized different precursors, such as
Physical vapor deposition
Physical vapor deposition (PVD) is a technique used to deposit thin films onto substrates. This process typically involves heating a material to its evaporation point, enabling the evaporated atoms or molecules to deposit onto the substrate under vacuum or controlled atmosphere conditions. PVD technology is widely used in industrial and research applications for its ability to produce high-quality films, including in semiconductor manufacturing, optical coatings, tool coatings, and the preparation of 2D materials.
Thermal evaporation is one of the simplest PVD techniques, involving heating the raw material above its melting point until it evaporates, and then depositing it onto a cooled substrate. Although relatively simple, thermal evaporation lacks the precision needed to obtain single-layer or few-layer 2D materials.
Electron beam evaporation (EBE) uses an electron beam to heat and vaporize the material, providing higher evaporation temperatures and better control over the evaporation rate compared to thermal evaporation. Consequently, this method allows for more precise deposition of thin films. For example, Madani et al. used EBE to deposit MoOx films of different thicknesses (5 and 10 nm) on glass substrates[30].
Molecular beam epitaxy (MBE) provides a highly precise and controllable methodology for the fabrication of high-quality monolayer and few-layer α-MoO3 thin films. This technique facilitates the accurate deposition of materials through molecular beams under ultra-high vacuum (UHV) conditions, yielding superior-quality 2D films that are especially well-suited for the preparation of ultrathin films in microelectronic devices. Through MBE growth on SrTiO3 (001) substrates, Du et al. successfully achieved self-limiting growth of monolayer α-MoO3 thin films[31].
Pulsed laser deposition (PLD) is an advanced thin-film deposition technique that employs high-energy laser pulses to irradiate a target material, resulting in its instant vaporization and the formation of a plasma. Particles from this plasma then deposit onto a substrate to form a thin film. Due to the high energy involved and rapid cooling during the deposition process, high-quality films with superior crystallinity and uniformity can be obtained. Bisht et al. used PLD technology to synthesize α-MoO3 2D layers and films[32]. Another widely used PVD technique is magnetron sputtering, which utilizes a magnetic field to enhance the electron density in the plasma, thereby increasing the sputtering efficiency and deposition rate. Zhao et al. used magnetron sputtering to deposit Ag and MoO3 on a polystyrene (PS) array, forming Ag/MoO3@PS composite materials[33].
Hot plasma-assisted techniques represent an innovative, efficient, and environmentally sustainable method for synthesizing nanomaterials. Rahman et al. used a cascaded arc plasma jet to generate a high-density flowing plasma stream, which interacted with a Mo metal target to synthesize 2D MoO3 films[34]. The device is shown in Figure 4. This method not only achieves high yields but also ensures dimensional uniformity of the 2D materials, offering new possibilities for the preparation of 2D materials using physical vapor deposition techniques.
Figure 4. The plasma assisted novel experimental reactor configuration for synthesis of molybdenum-oxide nanomaterials. Inset shows that the laminar plasma beam engulfs the large molybdenum target plate, leading to high-rate generation of the metal-oxide powders (reprinted with permission[34]. Copyright 2024, Elsevier).
Chemical vapor deposition
Chemical vapor deposition (CVD) is a technique used for preparing high-purity, high-performance semiconductor material films. In the field of 2D materials, CVD has become one of the critical methods for fabricating large-area, high-quality 2D materials. During the CVD process, reactant gases are introduced into a high-temperature reaction chamber. These gases undergo chemical reactions or decomposition on the substrate surface, depositing to form the desired solid film. Although CVD shows great potential in the preparation of 2D materials, there remain several challenges, such as controlling the crystal phase, size uniformity, and defect density, as well as efficiently transferring the grown films to different substrates without compromising their properties. Additionally, this method imposes stringent requirements on the substrate, making it difficult for the target product to grow on substrates that do not match its lattice.
Hong et al. achieved controllable synthesis of ultrathin α-MoO3 single crystals using a combination of CVD and plasma pre-treatment assistance[35]. AFM images show that the grown material has a thickness of approximately 3 nm, equivalent to about two layers of MoO3 nanosheets. Deng et al. reported a flux growth strategy using KCl as a flux agent to prepare 2D non-layered MoO2 via the CVD method[36]. This approach shares similarities with the “molten salt assisted method” (MSAM) previously reported in the literature for the preparation of large-area 2D materials. By controlling the reaction temperature, homogenization time, and cooling rate, they successfully synthesized high-crystallinity non-layered 2D MoO2 nanosheets with lateral dimensions reaching sub-millimeter scales (~464 micrometers), which represent the largest known non-layered 2D metal oxide single crystals to date [Figure 5A][36].
Figure 5. (A) Flux-grown method, typical SEM image of flux-grown MoO2 flake and HAADF image of MoO2 flake viewed along [201] zone axis. (B) UV-ozone oxidation of wafer-scale MoS2. Under the UV light, ambient oxygen converts into ozone (ozone generation) and the generated ozone reverts (ozone decomposition) to ambient oxygen. Atomic oxygen is produced during ozone generation and decomposition, which reacts with MoS2 and converts it to MoO3. Two-inch sapphire wafer with MOCVD grown monolayer MoS2 (C) before and (D) after 30 min of UV-ozone oxidation at 120 °C. The 2D MoS2 converts into a transparent film after oxidation. (A) reprinted with permission[36],2024, CC BY license. (B-D) reprinted with permission[42].Copyright 2022, American Chemical Society.
Atomic layer deposition (ALD), based on self-limiting surface reactions, provides an effective method for preparing MoO3 thin films with complex optoelectronic device functionalities. Xu et al. used C12H30N4Mo as the Mo precursor and O2 plasma as the oxygen source to deposit MoO3 films of varying thicknesses on gold electrodes using ALD technology[37]. Kim et al. used ALD technology to deposit MoOx films at 170 °C, utilizing Mo(CO)6 and ozone as the Mo precursor and reactant, respectively[38]. Through rapid annealing treatment at different temperatures, they achieved the formation of self-isolated electrodes of conductive MoO3 on TiN surfaces and insulating MoO3 on SiO2 surfaces.
Vapor phase epitaxy (VPE) is a specialized form of CVD known for its fast growth rates and the alignment of crystal orientation with the substrate. Lee et al. used the VPE method to obtain high crystalline MoO3 nanosheets[39].
Other methods
In addition to the aforementioned methods, several alternative strategies for the preparation of 2D Mo-based oxides have been reported in the literature.
Kundu et al. employed a modified co-precipitation technique, in which they adjusted the synthesis temperature and introduced a surfactant (PEG 6000) to control the phase and morphology of MoO3[40]. They successfully synthesized 2D MoO3 nanostructures with high specific surface area and porosity.
Zhu et al. used C3N4 as a sacrificial soft template to prepare MoO3 nanosheets via high-temperature thermal treatment[41]. This approach avoids the use of strong acids for template removal, thereby mitigating environmental pollution.
As shown in Figure 5B, Alam et al. prepared wafer-scale amorphous MoO3 monolayers through ultraviolet (UV)-ozone oxidation of MoS2 [Figure 5C], achieving high uniformity and stability [Figure 5D][42]. Liu et al. utilized a simple photoradiation method to prepare MoO3-x nanosheets with a layered structure[43]. Using a tungsten iodide lamp filament as the light source, they placed a piece of Mo foil on a glass or ceramic slide positioned centrally within a quartz tube. By controlling the temperature, the Mo foil was vaporized and oxidized into MoO3-x. This method allows the nanosheets to grow in situ without any catalysts or templates, forming within approximately 2 min at around 535 °C in an air atmosphere.
Qi et al. used H2MoO4 as a precursor and oleic acid (OA) and oleylamine (OAm) as additives to prepare 2D MoO3-x nanosheets via a one-step colloidal method[44]. Under the influence of OA and OAm, H2MoO4 undergoes a topological phase transformation, leading to the breaking of Mo-O bonds and a transition from the hexagonal phase to layered α-MoO3, while also creating oxygen vacancies. The resulting MoO3-x nanosheets had a thickness of approximately 2.01 nm.
STRUCTURAL REGULATION
Two-dimensional Mo-based oxides exhibit significant potential across various fields due to their unique structural characteristics and excellent physicochemical properties. However, they also face certain limitations, such as poor conductivity and insufficient density of active sites, which restrict their performance in practical applications. To overcome these limitations, researchers have proposed multiple strategies for structural modulation of 2D Mo-based oxides, aiming to enhance their performance by altering the structural features and expanding their application scope.
Here we summarize some of the structural modulation strategies for 2D Mo-based oxides, including traditional material engineering approaches such as phase transformation control, doping, and defect engineering. Given the unique structural characteristics of 2D Mo-based oxides, such as interlayer spacing, we also discuss special structural modulation strategies, specifically interlayer modulation and interface engineering.
Doping and defect engineering
Two-dimensional MoO3 exhibits high dopability, which allows for the adjustment of its physical and chemical properties through the doping of various atoms or molecules. For example, Shkir et al. investigated the effects of doping rare earth elements such as Er, Gd, and Pr on the performance of MoO3 thin films[45]. Doping led to structural changes within the MoO3 lattice. These changes may introduce additional defects, increasing the number of adsorption sites, thereby enhancing the gas-sensing performance of MoO3.
Non-metallic elements are also popular choices for doping. Li et al. found that by introducing hydrogen, 2D MoO3 nanodisks exhibit tunable plasmonic resonance characteristics, enhancing the interaction between light and matter[46]. H-doped MoO3 demonstrates enhanced surface plasmon resonance response in the visible to near-infrared wavelength range, thereby improving the potential application of the material in optical sensing. Furthermore, Li et al. proposed a method for inducing the synthesis of MoO3 nanosheets through sulfur doping (S-doping)[47]. By adjusting the amount of S used in the solvothermal process, they prepared MoO3 nanosheets with different morphologies. The S-doped MoO3 nanosheets exhibited high responsiveness and selectivity towards ethanol and isopropanol[47].
For 2D metal oxides, oxygen vacancies are a critically important type of defect. By precisely controlling oxygen vacancies, the functional properties of the material can be significantly improved. In oxygen-deficient molybdenum oxides such as MoO3-x (2 < x < 3), the presence of oxygen vacancies increases the free charge carrier density. Under illumination, the free electrons on the surface resonate collectively with incident light, leading to strong photon absorption, a phenomenon known as LSPR[48]. MoO3-x exhibits a broad LSPR range (600 to 2,500 nm), and the intensity and range of LSPR can be modulated by changing the concentration of oxygen vacancies. More importantly, oxygen vacancies can act as active sites. Therefore, molybdenum oxides with controlled oxygen defects have garnered significant attention for their superior performance in electrochromic, photothermal, sensor, and catalytic applications. Sub-stoichiometric Mo-based oxides can exhibit various properties due to differing levels of oxygen vacancy content. As a result, strategies for regulating oxygen vacancy defects have received extensive research interest.
The hydrothermal method can introduce abundant oxygen vacancies during the preparation process without requiring additional steps. Li et al. used the hydrothermal method to prepare MoO3-x nanosheets rich in oxygen vacancies and doped them with Ru elements, successfully producing Ru/MoO2-x catalysts[49]. Figure 6A illustrates that the Mo5+ ion is in a low oxidation state, indicative of the formation of MoO3-x with oxygen vacancies. Furthermore, as depicted in Figure 6B, the MoO3-x phase exhibits a blue color, which transitions to white MoO3 upon annealing in air, corroborating the presence of oxygen vacancies[50,51]. The ζ potential measurement in Figure 6C reveals a negative surface charge for MoO3-x in deionized water, suggesting that the high concentration of oxygen vacancies endows MoO3-x with a significant negative charge, thereby offering ample anchoring sites for Ru3+ adsorption. The X-ray diffraction (XRD) pattern in Figure 6D confirms the crystal structure of Ru/MoO2-x. In contrast, the absence of Ru3+ leads to the A-MoO3-x sample annealing into the MoO3 phase (PDF#01-0706), rather than the MoO2 phase, indicating the structural transition induced by Ru incorporation. Figure 6E and F shows that Ru/MoO2-x retains the nanolayered structure of the MoO3-x precursor, with Ru nanoclusters visible within the red box in the high-resolution transmission electron microscopy (HRTEM) image [Figure 6G]. The annular dark field scanning transmission electron microscopy (ADF-STEM) image displays lattice fringes with a spacing of 0.208 nm, corresponding to the (101) plane of Ru [Figure 6H and I]. Consequently, the presence of oxygen vacancies facilitates the anchoring of Ru3+, and the addition of Ru promotes the formation of Ru-O-Mo sites, thereby enhancing the conversion of MoO3 to MoO2. Research by Sun et al. demonstrated that by adjusting the temperature and the ratio of ethanol to deionized water during the hydrothermal synthesis process, the concentration of oxygen vacancies can be effectively controlled[52]. They fabricated MoO3-x-based H2S sensors and found that the MoO3-x material with the highest concentration of oxygen vacancies exhibited the best detection performance for H2S.
Figure 6. (A) Mo 3d core level spectrum. (B) The photographs of the MoO3-x and MoO3 powder dispersed in ethanol. (C) Comparison of zeta potential of MoO3-x and MoO3. (D) XRD pattern. (E) SEM image of MoO3-x. (F) SEM, (G) HRTEM, (H and I) ADF-STEM images of Ru/MoO2-x. All panels reprinted with permission[49]. Copyright 2022, Elsevier.
In addition, Sun et al. reported a strategy utilizing a rapid carbon thermal shock method to construct oxygen vacancies on MoO2 and prepare Fe-MoO2 electrocatalysts[53]. In this strategy, rapid carbon thermal shock was used to introduce Fe atoms into MoO2. Due to the different spin states of Fe and Mo, the introduction of Fe atoms leads to abundant lattice misalignments in Fe-MoO2, promoting the formation of oxygen vacancies. During the rapid heating and cooling process, extreme temperature changes cause lattice distortion and stress, further facilitating the formation of oxygen vacancies. The incorporation of Fe atoms and the abundance of oxygen vacancies in MoO2 can alter the distance of Mo-O bonds, modulate the electronic structure, and optimize the performance of the catalyst[53].
Studies have shown that combining doping and defect engineering strategies can yield better results.
Interface engineering
Interface engineering involves the design and control of the chemical and physical properties at the interfaces between materials. Heterojunctions have been a popular topic in the study of interface engineering for 2D materials. By stacking different types of 2D semiconductor materials, artificial heterojunction structures can be created. These structures can give rise to new physical phenomena and can be designed to have specific functionalities for devices. Due to the single-layer nature of 2D materials, their interactions can be achieved through van der Waals forces, eliminating the need for precise lattice matching required in traditional semiconductors.
Ou et al. employed a simple probe sonication method to synthesize Dy2O3/MoO3 2D heterostructures, which enable high sensitivity ammonia detection at lower temperatures[25]. Figure 7A-C shows the energy levels within Dy2O3/MoO3 composite. The abundance of heterojunctions enhances the regulation of carrier concentration, and the compensatory/protective effect of Dy2O3 mitigates the impact of humidity, giving the sensor good humidity independence. Due to the difference in work functions between the two materials, electrons transfer from Dy2O3 to MoO3, causing more oxygen molecules to be attracted to the
Figure 7. (A) Schematic illustration of the energy levels within Dy2O3/MoO3 composites before contact and after contact, (B) In air, and (C) In NH3 and the corresponding diagram of the gas-sensing process (D) In air and (E) In NH3. All panels reprinted with permission[25].Copyright 2023, American Chemical Society.
Similar to p-n junctions, Schottky junctions are another type of interface structure formed by the contact between a metal and a semiconductor. For example, Ding et al. prepared Mo-modified MoO3 nanosheets using a method that enhances photocatalytic efficiency through the synergistic effect of in-situ constructed Schottky junctions and oxygen vacancies[28]. The Schottky barrier formed between Mo and MoO3 creates a built-in electric field, which facilitates charge separation during the photocatalytic process. Characterization methods such as electrochemical impedance spectroscopy can further confirm that the construction of a Schottky junction is more beneficial for charge transfer and separation of photogenerated charges compared to oxygen vacancies alone.
Z-type heterojunctions exhibit several advantages over traditional heterojunctions, particularly in enhancing photocatalytic efficiency. For instance, in the Z-type heterojunction constructed by Wang et al. between MoO3 and g-C3N4, the conduction band position of MoO3 is close to the valence band position of g-C3N4, which facilitates the migration of carriers[56]. Additionally, oxygen vacancies present in MoO3 act as shallow donors, imparting metallic conductivity to MoO3 and serving as new active sites, which help adjust the bandgap and promote visible light absorption and utilization. The 2D/2D Z-type heterojunction formed by MoO3/g-C3N4 not only increases the density of active sites and promotes the separation of photogenerated carriers but also enhances photocatalytic activity. Furthermore, the matched energy band positions and reduced aggregation contribute to enhanced catalytic activity and stability.
Peng et al. synthesized 0D/2D CdS/MoO3-x heterojunctions via a simple co-precipitation method[57]. The introduction of CdS nanoparticles forms an S-scheme heterojunction with MoO3-x. In this heterojunction, the interface between CdS and MoO3-x creates an internal electric field, which effectively separates photogenerated electrons and holes, enhancing charge separation effect. The structure of the S-scheme heterojunction also shortens the migration distance between electrons and holes, accelerating charge separation. This charge transfer mechanism [Figure 8A] helps to enhance the plasmonic resonance effect caused by the abundant oxygen vacancies in MoO3-x and enables the 0D/2D CdS/MoO3-x heterojunction to possess higher photocatalytic H2 generation capability, approximately 10.3 times that of the original CdS nanoparticles [Figure 8B and C]. Similarly, Wei et al. synthesized a 1D/2D Zn0.1Cd0.9S/MoO3-x S-scheme heterojunction by loading 1D Zn0.1Cd0.9S nanorods onto 2D MoO3-x nanosheets[58]. The charge transfer mechanism of the S-scheme heterojunction enhances the redox capabilities of the carriers, resulting in excellent photocatalytic H2 generation rates, approximately six times higher than those of pure Zn0.1Cd0.9S. Wang et al. prepared a dual plasmon-enhanced 2D/2D/2D g-C3N4/Pd/MoO3-x (CPM) S-scheme heterojunction[59]. The dual LSPR effect of MoO3-x and Pd nanosheets synergistically broadens the optical response range of CPM, enhancing the photocatalytic activity.
Figure 8. (A) Mechanism of photocatalytic H2-production using CdS/MoO3-x composites. Photocatalytic H2 evolution performances (B) and H2 evolution rates (C) of CdS, MoO3-x, MC-15 is a composite material containing 15 wt% MoO3-x nanosheets. All panels reprinted with permission[57]. Copyright 2021, Elsevier.
When material type is not a limiting factor, the contact between materials can be extended to multiple layers to form heterostructures. Yoon et al. found that the high work function of MoO3 can reduce the contact resistance with MoS2, eliminating the Fermi level pinning effect[60]. This makes the MoS2-MoO3 heterostructure highly promising for applications as p-type transistors. Krishnan et al. revealed that the MoO3-hBN heterostructure exhibits incoherent stacking due to lattice mismatch, which enables ultralow friction because they possess low shear strength in all sliding directions[61]. Compared to homo-bilayers such as MoO3-MoO3 or hBN-hBN, the heterostructure provides a lower coefficient of friction.
The studies presented in this section highlight the significant potential of interface engineering in enhancing the performance of 2D Mo-based oxides. A common trend across these studies is the critical role of band alignment, interfacial defects, and charge transfer dynamics in determining the performance of heterojunction-based devices. However, challenges remain in achieving precise control over interface properties, such as defect density and interfacial strain, which can significantly impact device performance.
Interlayer tuning
MoO3 has its internal layers connected by covalent bonds and separated by van der Waals forces. When foreign substances such as metal ions or other compounds are intercalated between these layers, the interlayer spacing of MoO3 is altered, thus influencing its physical properties. Wang et al. introduced a composite material prepared by inserting Keggin-type polycationic AlO4Al12(OH)24(H2O)127+ (Al13) into the interlayers of MoO3[62]. These composites can rapidly adsorb anionic dye methyl orange (MO) through strong electrostatic interactions, leading to the formation of tight and stable aggregates around the charged Al13 clusters.
Intercalation can improve the electrochemical performance of MoO3 when used as a cathode material, as confirmed by the research of Tan et al. By intercalating organic molecules such as polyaniline (PANI) into MoO3, the storage capacity for Zn2+ can be effectively enhanced[63]. The insertion of PANI not only increases the interlayer spacing of MoO3 but also stabilizes the layered structure of MoO3, thereby improving the capacity and cycling stability of the material.
Gao et al. synthesized ultra-thin nanosheets of Li-ion intercalated MoO2-x and prepared Fe@MoO2-x/Li ultra-thin nanosheets for use as catalysts in ammonia synthesis[64]. Through thermal treatment, Li ions and electrons were intercalated into the MoO2 layers, causing lattice expansion. The introduction of electrons reduces the oxidation state of Mo, generating MoO2-x. The downshift of the d-band in MoO2-x lowers the activation energy of the reaction, thus promoting ammonia synthesis. The introduction of Fe significantly facilitates the intercalation of Li ions and the exfoliation of MoO2, further lowering the energy barrier of the reaction[64].
Reed et al. chose different metal intercalants (Au, Cr, Fe, Ge, Mn, and Ni) to modulate α-MoO3[65]. The study demonstrated that the anisotropy of α-MoO3 can be regulated by selecting different metal intercalants. Except for Ge-MoO3, all other intercalations reduced the anisotropy of MoO3. Additionally, the color of the materials changed from transparent white to near black or dark blue, which is associated with a reduction in the bandgap and increased absorption at wavelengths greater than 600 nm, particularly for Cr-, Ge-, and Mn-MoO3 intercalated samples.
Hu et al. reported a strategy for preparing organic/inorganic superlattice structures through organic intercalation[66]. By co-intercalating Na+/H2O into MoO3 nanoribbons and then performing guest exchange in situ polymerization with aniline monomers, they obtained 2D PANI/MoO3-x superlattice nanosheets. The embedding of PANI not only induces the structural morphological transformation of MoO3 nanoribbons into 2D PANI/ MoO3-x superlattice nanosheets but also partially reduces Mo6+ to Mo5+, creating abundant oxygen vacancies. Consequently, the 2D PANI/MoO3-x superlattice nanosheets exhibit excellent Fenton-like catalytic activity in the generation of ·OH from H2O2.
Interlayer tuning represents a versatile strategy for modulating the properties of 2D Mo-based oxides. However, achieving precise control over the intercalation process remains challenging, particularly with respect to uniformity and scalability. Future research should focus on exploring novel intercalants with multifunctional properties, such as those capable of simultaneously enhancing conductivity and catalytic activity. The development of large-scale intercalation methods will also be critical for the practical application of these materials in energy storage and catalysis. By addressing these challenges, interlayer tuning can continue to unlock new possibilities for the design and optimization of 2D Mo-based oxides.
Phase transition
Phase transitions, as a common regulation strategy, have been extensively studied in the field of materials science. For 2D transition metal oxides such as MoO3, phase transitions can bring about unique physical properties, such as enhanced electrochemical performance, improved optical characteristics, and mechanical properties. By applying different external conditions to the material, such as pressure or temperature changes, a phase transition can be induced, altering the crystal structure, bandgap width, and optical absorption properties. Under high pressure, MoO3 undergoes significant structural changes. At around 11 GPa, the orthorhombic α-MoO3 (Pnma) phase transforms into the monoclinic MoO3-II phase (P21/m). This transition is driven by shear stress, which causes a rearrangement of the MoO6 octahedra and a change in the stacking sequence of the layers, leading to the loss of the original 2D layered structure. As the pressure increases further to around 27 GPa, MoO3-II undergoes another phase transition to form the orthorhombic MoO3-III phase (Pmma). This transition is marked by the disappearance of out-of-plane vibrational modes, indicating the complete loss of the layered structure and the formation of a 3D crystalline phase[67]. These high-pressure phases exhibit distinct optical and electronic properties, such as a narrower bandgap and enhanced optical absorption, which are crucial for potential applications in optoelectronics and energy storage.
Wang et al. focused on the effects of high pressure on the phase transitions of MoO3 and the changes in its optical properties[68]. They found that under a pressure of 10 GPa, the α-phase MoO3 transforms into another high-pressure phase, MoO3-II. When the pressure exceeds 25 GPa, it transforms into MoO3-III. After releasing the pressure, the MoO3-II phase remains stable, indicating that the phase transition is irreversible. In terms of optical properties, the quenched MoO3-II phase exhibits a narrower bandgap (reduced by approximately 25%) and stronger optical absorption within the bandgap compared to the original low-pressure α-MoO3 phase. This discovery is significant for exploring high-pressure phase materials for applications under ambient pressure conditions.
The work by Kundu et al. involves using an improved co-precipitation method to prepare MoO3 nanostructures[40]. Through a simple surfactant-assisted synthesis process and slight temperature variations, researchers successfully achieved a phase transition from hexagonal phase MoO3 nanorods to α-MoO3 nanosheets. These 2D α-MoO3 nanosheets exhibited superior electrochemical performance, attributed to their larger specific surface area and the presence of open van der Waals gaps. Particularly when used as electrode materials for asymmetric all-solid-state supercapacitors, they showed a high pseudocapacitive response. Additionally, these materials retained up to 91% of their initial specific capacitance after 2,000 charge-discharge cycles at a current density of 5 A g-1, demonstrating excellent electrochemical stability.
APPLICATIONS
This section will focus on the recent applications of 2D Mo-based oxides in various fields, including energy and catalysis, sensors, and electronic and optoelectronic devices. While some studies have previously discussed synthesis methods and tuning strategies, this section will primarily emphasize the performance of these materials. Table 2 summarizes the applications of 2D Mo-based oxides in recent years.
Summary of recent 2D Molybdenum oxide applications
Applications | Materials | Performance metrics | Refs. | |
Energy and catalysis | Supercapacitors and batteries | MoO3 | Specific capacitance: 994.2 F g-1 at 0.5 A g-1 Energy density: 35.5 Wh kg-1 | [41] |
α-MoO3 | Capacity: 285 mA h g-1 at 0.4 A g-1 Capacity retention: 86% after 40 cycles | [71] | ||
Photocatalysis | MoO3@ZrO2 | DS removal rate: 90.94% | [73] | |
Cu-α-Fe2O3/α-MoO3 | NPOC removal rate: 76.29% | [74] | ||
Ce-MoO3 | Photocatalytic activity: 2,858 mA mg-1 | [75] | ||
MoO3/g-C3N4 | H2 generation rate: 328.75 μmol·g-1·h-1 | [56] | ||
Mo@MoO3 | NH3 production rate: 50.78 μmol·g-1·h-1 | [28] | ||
cellulose over MoO3 | NH3 production rate: 68 μmol·h-1·g-1 | [27] | ||
Electrocatalysis | Ru/MoO2-x | Overpotential: 29 mV at 10 mA cm-2 Tafel slope: 22 mV dec-1 | [49] | |
P- Fe3O4-MoO2 | Overpotential: 189 mV at 10 mA cm-2 | [77] | ||
Pd@MoO3 | Overpotential: 71 mV at 10 mA cm-2 Tafel slope: 42.8 mV dec-1 | [78] | ||
am-MoO3 | NH3 production rate: 480.4 μmol·cm-2·h-1 Faraday efficiency: 94.8% | [24] | ||
Solar cells | L-MoOx | PCE: 22% | [80] | |
SnS/MoO3 | PCE: 4.4%; VOC: 437 mV | [81] | ||
US-MoOx | PCE: 19.6% | [82] | ||
Sensors | a-MoO3 | Limit of detection for o-AAT: 10-9 M | [23] | |
Dy2O3/MoO3 | 10 ppm NH3 response: 30.52 at 328.2 °C; | [25] | ||
MoO3 | 10 ppm DIPA response: 30.4 at 217 °C; | [29] | ||
MoO3 | Humidity sensitivity: 9,794 Ω/RH at 25 °C | [84] | ||
Electrochromism | W-doped MoO3 | Switching time: 3.9 s coloring efficiency: 40.43 cm2 C-1 | [86] | |
MoO3 | N/A | [21] | ||
Electronic and optoelectronic devices | MoO3 | OTFT-Ag/MoO3/NPB on/off ratio: 3.72 × 103 | [88] | |
α-MoO3 | Memristor response: 10 μs Roff/Ron: > 104 | [89] | ||
α-MoO3 | Set voltage: 0.5 V; on/off ratio: 104 | [35] | ||
MoO3 | Threshold switching voltage: +0.73 V; Applied current: 4-6 × 107 A | [37] | ||
CsPbBr3 QDs/MoO3 | Photodetector responsivity: 8.4 A/W in UV light; on/off ratio: 118 | [39] |
Energy and catalysis
The superior performance of 2D materials in energy-related applications is attributed to their atomic-scale thickness and unique physical properties, including high surface area and tunable electronic properties. These features enable higher density of active sites and controllable catalytic properties, rendering them highly adaptable for various energy technologies[69]. Moreover, their mechanical flexibility, optical transparency, and superior thermal conductivity further enhance their applicability in fields ranging from energy storage to conversion and harvesting.
Supercapacitors and batteries
Two-dimensional materials such as graphene exhibit high specific surface area, excellent electrical conductivity, and chemical stability, qualifying them as ideal electrode materials for supercapacitors. The capacitive performance of these materials can be further enhanced by manipulating their layer count or through integration with other materials.
Two-dimensional MoO3, with its multiple possible oxidation states, can engage in numerous REDOX reactions during the charge-discharge cycle, thereby broadening the electrochemical window. Additionally, MoO3 is cost-effective, eco-friendly, and boasts a high theoretical capacity (1,005 C g-1). Notably, α-MoO3 has been the subject of extensive research due to its anisotropic layered structure, which aids in the intercalation and deintercalation of electrolyte ions. Nevertheless, MoO3 faces challenges such as its low electrical conductivity, which affects its current response and capacitance characteristics. To enhance its performance, it is imperative to optimize crystal size and morphology[40]. Zhu et al. enhanced the properties of MoO3 nanosheets using a templating method, achieving a specific capacitance of up to 994.2 F g-1 in an acidic electrolyte at a current density of 0.5 A g-1[41]. A supercapacitor device constructed with YP50 as the negative electrode and MoO3 as the positive electrode reached an energy density of 35.5 Wh kg-1. Moreover, the device operated at a power density of 350.0 W kg-1 and maintained 84% of its initial specific capacitance after 1500 cycles, demonstrating commendable cycle stability.
In lithium-ion batteries and beyond, 2D MoO3 serves as an electrode material, offering enhanced capacity and rapid charging capabilities. Moreover, it can be employed as a separator coating to bolster the safety and cycle stability of batteries. Studies have shown that MoO3 can modify lithium-sulfur battery separators, enhancing their initial capacity and cycle stability[70]. Hsu et al. introduced α-MoO3 as a material for Al-ion energy storage within an air-stabilized hydrated eutectic electrolyte (ASHEE) system[71]. The α-MoO3 electrode exhibits a high capacity of approximately 285 mA h g-1 and maintains about 86% of its capacity after 40 charge-discharge cycles at a current density of 0.4 A g-1. These findings suggest that α-MoO3 holds promise as a positive electrode material for Al-ion storage in ASHEE systems.
Photocatalysis
In photocatalysis, 2D MoO3 absorbs specific light wavelengths and generates electron-hole pairs due to its unique band structure, facilitating chemical reactions. Introducing oxygen vacancies or forming heterojunctions can significantly enhance the catalyst’s performance, thereby increasing the efficiency of the target reaction. Photocatalysis, encompassing applications such as pollutant degradation, H2 production, CO2 reduction, and N2 fixation, represents a promising approach to realizing green, efficient, and safe technologies[72].
Ashraf et al. synthesized a novel MoO3@ZrO2 nanocomposite with a Z-type heterostructure for the degradation of diclofenac sodium (DS)[73]. This nanocomposite achieved a DS removal rate of 90.94% under simulated light/peroxymonosuifate (PMS) conditions, demonstrating high stability and recyclability. This study underscores the significant potential of MoO3@ZrO2 composites for innovative water purification solutions powered by visible light. Wang et al. developed a hydrothermal oxygen decoupling (HTOU) system using a Cu-α-Fe2O3/α-MoO3 catalyst for biogas sludge (BS) treatment[74]. This catalyst exhibits van der Waals heterojunction and REDOX properties. Experimental results indicate that the Cu-α-Fe2O3/α-MoO3 catalyst achieved removal efficiencies of 76.29% for non-volatile organic carbon (NPOC), 45.56% for total nitrogen (TN), and 29.03% for ammonia nitrogen (AN) after 30 min reaction at 225 °C. The significant deactivation of the catalyst was attributed to aromatic polymer deposition and heavy metal contamination. This research highlights the potential of efficient and cost-effective oxygen decoupling to enhance hydrothermal treatment of highly concentrated organic wastewater.
Zhao et al. synthesized a Ce-MoO3 nanosheet-supported Pt nanoparticle catalyst with oxygen vacancies (OVs-Ce-MoO3/Pt), demonstrating exceptional performance in electrocatalysis and photocatalytic oxidation of methanol[75]. The catalyst exhibited a photocatalytic activity of 2,858 mA mg-1, surpassing other catalysts in terms of anti-poisoning ability and long-term stability[75].
Wang et al. constructed a 2D/2D Z-type heterojunction photocatalyst, MoO3/g-C3N4, with enhanced REDOX capabilities for photocatalytic hydrogen production and tetracycline (TC) degradation[56]. The 3% MoO3/g-C3N4 composite achieved an optimal hydrogen generation rate of 328.75 μmol·g-1·h-1, approximately 87-fold higher than that of pure g-C3N4 without any noble metal cocatalyst. Additionally, the degradation kinetic constant for TC was 0.0196 min-1, about 8.28 times that of pure g-C3N4.
The research by Ding et al. revealed that the N2 photocatalytic nitrogen fixation performance of
Figure 9. Schematic presentation of photocatalytic nitrogen fixation coupled with the generation of value-added chemicals from N2 and cellulose over MoO3 nanosheets as well as the composition and structure of cellulose in lignocellulosic biomass. Reprinted with permission[27].Copyright 2024, American Chemical Society.
Electrocatalysis
Electrolysis of water or electrochemical water splitting for hydrogen production has been receiving attention due to its promising prospects, with advantages such as stable output, high purity of hydrogen, and scalability. However, due to the slow rate of the hydrogen evolution reaction (HER), there has been ongoing research into efficient electrocatalysts to accelerate this process[76].
Li et al. prepared a Ru/MoO2-x catalyst that demonstrated exceptional performance in alkaline HER, achieving an ultra-low overpotential of 29 mV at a current density of 10 mA cm-2[49]. The current density of this catalyst was 7.3 times higher than that of commercial Pt/C [Figure 10A and B], and it maintained its performance stability even after 1,000 cycles of cyclic voltammetry [Figure 10C]. This positions the Ru/MoO2-x catalyst as a potential replacement for precious metal catalysts. Similarly, Wang et al. have demonstrated an enhancement in the electrocatalytic performance of MoO2 by synthesizing P-doped Fe3O4-MoO2 nanosheets supported on N-doped carbon flakes, thereby altering the electronic configuration of MoO2[77]. Benefiting from the synergistic effects of P doping, the heterojunction interface, and the support provided by the N-doped carbon matrix, the synthesized P-Fe3O4-MoO2/NC material displayed superior activity for water electrolysis, exhibiting notable stability and surpassing the performance of commercially available RuO2 catalysts. In addition, Li et al. successfully designed a Pd@MoO3 heterostructure by confining Pd nanoparticles and atomically dispersed Pd sites into defect-rich 2D MoO3 nanosheets through the interface engineering strategy, significantly enhancing its HER performance in an acidic medium[78]. The catalyst exhibited outstanding HER activity, with an overpotential of 71 mV and a Tafel slope of
Figure 10. (A) LSV curves of Ru/MoO2-x, A-MoO3-x, Ru/C, and commercial Pt/C catalyst in 1 M KOH. (B) The mass activities of Ru/MoO2-x and Pt/C. (C) Durability of Ru/MoO2-x for 1,000 cycles CV cycles in 1 M KOH. All panels reprinted with permission[49]. Copyright 2022, Elsevier.
Wu et al. reported on a novel, efficient, and durable catalyst for the electroreduction of nitrite ions to ammonia - amorphous MoO3 nanosheets[24]. The am-MoO3 catalyst achieved a maximum NH3 yield of 480.4 μmol·cm-2·h-1 at -0.6 V vs. the reversible hydrogen electrode (RHE) and exhibited a Faraday efficiency of up to 94.8% for converting NO2- to NH3. Moreover, the catalyst demonstrated excellent long-term stability, withstanding continuous electrolysis tests for up to 30 h.
Solar cells
Mo-based oxide films are commonly employed in solar cells due to their high work function and broad band gap. As a hole transport layer, they effectively facilitate hole transport and minimize contact recombination, thereby enhancing the device’s efficiency and stability[79]. Li et al. investigated the use of low-oxygen MoOx (L-MoOx) films as hole transport layers to boost the efficiency and stability of crystalline silicon solar cells[80]. The L-MoOx films, deposited using a MoO2 evaporation source, possess a wide band gap that inhibits the diffusion of Ag and Si into MoOx, thereby improving the device’s stability in ambient conditions.
Suzuki et al. fabricated solar cells using n-type SnS single crystal junctions combined with MoO3 thin films to elevate the VOC. They achieved a record-high VOC of 437 mV for SnS solar cells[81]. Wang et al. developed a series of organic photovoltaic cells with an impressive photoconversion efficiency of 19.6%[82]. In their design, a 7 nm-thick MoOx layer was incorporated into the interconnecting layer (ICL). The MoOx layer, integrated with materials such as Ag and ZnO:PFN-Br, formed a comprehensive interconnect structure within the series-connected cells [Figure 11A and B][82].
Figure 11. (A) Device structure of tandem cells. (B) Energy level diagram of each layer in tandem cells. All panels reprinted with permission[82].Copyright 2021, Wiley-VCH.
Liu et al. employed UV ozone treatment (UVO) and non-thermal annealing to modify MoOx films (US-MoOx)[83]. This treatment yielded dense films with elevated energy levels by decomposing
Sensors
Surface-enhanced raman scattering (SERS) sensors work by exploiting the significant enhancement of Raman scattering signals that occur when a substance adheres to specific rough metal surfaces or in the proximity of nanoscale metallic particles. This methodology enables the highly sensitive and selective detection of chemical compounds and has extensive applications in the fields of chemistry, biomedicine, and environmental monitoring. In the study conducted by Meng et al., they employed an amorphous monolayer of MoO3 (a-MoO3) as the substrate for SERS sensors to detect trace levels of carcinogenic aromatic amines. a-MoO3 exhibits an LSPR effect within the visible light spectrum, facilitating the plasma-induced hot electron transfer (PIHET) process and thereby enhancing the performance of SERS detection[23].
Two-dimensional nanostructures, characterized by their high surface areas and porosity, endow 2D nanosheets or films with enhanced potential for use in gas sensing applications. Mo-based oxides, which possess various oxidation states and a layered structure, can fine-tune the selectivity for specific gases by altering their chemical composition, morphology, and structure, thereby significantly improving the specificity of gas sensors. Recent research has focused particularly on detecting NO2[32], NH3[45], and other harmful gases[26,47]. For instance, 2D Dy2O3/MoO3 heterostructure sensors developed by Ou et al. demonstrated a 4.49-fold superior response to 10 ppm NH3 at a reduced temperature of 328.2 °C, with quicker response/recovery times, 52.6-fold enhanced sensitivity, and a lower detection limit compared to a pure MoO3 sensor. Moreover, the response of the Dy2O3/MoO3 sensor remains largely unaffected by humidity[25]. Xiao et al. designed a porous MoO3 nanosheet gas sensor for detecting diisopropylamine (DIPA), which exhibited remarkable performance at 217 °C, including an exceptionally high response (30.4 at 10 ppm), a low detection limit (10 ppb), and rapid response times (4.3 s), along with moisture resistance, excellent selectivity, and long-term stability for up to 100 days[29].
Additionally, Jiang et al. investigated the utility of 2D MoO3 for humidity sensing. The MoO3 derived from liquid-phase exfoliation demonstrated heightened sensitivity (9,794 Ω/RH at 25 °C) and robust stability in humidity detection[84]. Research indicates that the humidity-sensing mechanism of MoO3 involves electron transfer facilitated by interfacial water molecules. The adsorbed H2O molecules create a conductive pathway via hydrogen bonding, which diminishes the electron transfer barrier and introduces additional electron states within the valence bands[84].
Electrochromism
Electrochromism refers to the reversible change in color or transparency of certain materials when subjected to an electric field. This phenomenon has been harnessed to develop intelligent glass that changes color, garnering significant interest in energy efficiency and environmental conservation. MoO3, known for its low manufacturing cost, broad light modulation capability, rapid response time, and extended cycle life, serves as an effective electrochromic material. Its electrochromic properties can be further enhanced through doping with other elements[85]. Gao et al., for instance, fabricated tungsten-doped MoO3 films on Indium Tin oxide (ITO) substrates using electrochemical deposition, as shown in Figure 12[86]. These films exhibited superior electrochromic properties. The layered structure of MoO3, combined with the incorporation of W atoms to promote efficient W-O-Mo bonding, reduced the switching time from 5.5 to 3.9 s. Moreover, doping increased the optical modulation amplitude by 1.33 times, decreased the coloring and bleaching times to 4.9 and 3.9 s, respectively, and improved the coloring efficiency to 40.43 cm2 C-1[86].
Figure 12. Mechanism underlying the electrochromism of W-doped molybdenum oxide films. Reprinted with permission[86]. Copyright 2023, Elsevier.
Kuriakose et al., after successfully isolating 2D MoO3 nanosheets through liquid phase exfoliation, formulated them into a customizable photochromic MoO3 ink[21]. This ink, designed according to the Fitzpatrick skin type system, is incorporated into a bio-based elastomer to create an adhesive UV patch. The patch facilitates a qualitative evaluation of UV-A radiation exposure, with its photochromic response under UV light correlating to a calculated minimum erythema dose (MED). This feature offers visual cues to users, assisting them in avoiding excessive exposure and protecting their skin from damage.
Electronic and optoelectronic devices
Two-dimensional monolayer MoO3, with a work function of approximately 6.9 eV, is well-suited for electronic and optoelectronic applications[87]. It serves as an effective interface modification layer, addressing the issue of high contact resistance at the metal-organic interface in organic thin-film transistors (OTFTs). However, its propensity to diffuse into organic semiconductors (OSCs) can compromise device stability. Yang et al. mitigated MoO3 diffusion by creating high-density organic barrier layer (OBL) films on the OSC active layer[88]. These interlayer materials (IMLs) not only reduced contact resistance and prevented minority carrier injection into the OTFT channel but also enhanced device stability and the on/off current ratio.
Shan et al. developed a memristor utilizing an α-MoO3 cross-point structure, which exhibited superior electrical characteristics, including endurance over 103 cycles, a switching ratio (ROFF/RON) exceeding 104, multilevel storage capability, and a fast response time of 10 μs[89]. Hong et al. discovered that ultra-thin α-MoO3 memristors with silver/graphene electrodes achieved a high on/off ratio of approximately 104, a low set voltage of about 0.5 V, a long retention time exceeding 104 s, and excellent repeatability in over 150 voltage cycle scans[35]. These studies indicate that 2D MoO3 is a promising material for post-Moore era memristors.
Xu et al. demonstrated that MoO3 films possess excellent photoelectric synaptic functionality, capable of simulating long-term potentiation (LTP) and long-term depression (LTD) processes under near-infrared light[37]. They also highlighted the potential of MoO3 films in advanced optoelectronic systems, particularly their unique ability to simulate image processing functions. Lee et al. fabricated a hybrid photodetector by coating CsPbBr3 quantum dots onto 2D MoO3 nanomaterials, resulting in a significantly improved photocurrent response across the UV to visible light spectrum[39].
CONCLUSIONS
This article systematically reviews the synthesis, structural modulation, and applications of 2D Mo-based oxides, specifically highlighting recent advances in MoO3 and MoO2, as well as substoichiometric MoO3-x and MoO2-x. Through a comprehensive summary of existing research, it is not difficult to see that the microstructure critically influences the performance of 2D Mo-based oxides, with various structural regulation techniques significantly affecting their electrical, optical, and catalytic properties. Therefore, it is imperative to consider these microscopic characteristics when designing new materials. The use of advanced digital technologies such as computational simulations and machine learning tools can help discover or understand novel materials or structures. This approach not only accelerates the design process of new materials but also aids in elucidating complex physical and chemical mechanisms. With the recognition of these technologies by the 2024 Nobel Prizes in Physics and Chemistry, we believe this will be a significant trend in future materials science development. Indeed, we are pleased to observe ongoing efforts in this direction concerning 2D Mo-based oxides[79,90].
It is worth noting that numerous studies have focused on the properties and applications of Mo-based oxides at low dimensional scales, especially monolayer Mo-based oxides[91]. Thanks to research into exotic properties of monolayer Mo-based oxides, such as surface plasmon resonance effects, twistronics, and chirality, will facilitate the development of new electronic and optoelectronic devices[92]. In addition, MoO3-x is beginning to show promise in emerging fields such as catalysis and sensors, indicating broad prospects for defect-rich Mo-based oxides[93,94].
Lastly, the methods for synthesizing Mo-based oxides and the underlying mechanisms for their regulation remain incompletely understood. The efficient preparation of high-quality 2D Mo-based oxides with specific properties remains an open question that requires further experimental and theoretical investigation. These works are also pertinent to the study of other 2D metal oxides. Research in 2D materials continues to offer many exciting opportunities for those engaged in scientific endeavors. As part of this field, Mo-based oxides will play a crucial role and usher in new developments.
DECLARATIONS
Authors’ contributions
Conceived and designed the study, conducted literature search and organized references: Zhao, Z.; Zhou, T.; Xiong, L.
Prepared the samples and collected the data, performed data analysis and wrote the main draft of the paper: Zhao, Z.; Zhou, T.; Lei, W.; Chen, K.; Tian, K.; Xiong, G.
Provided technical and material support, advised on scientific discussion and manuscript revision: Xiong, L., Kuang, S.; Yu, Y.; Huang, L.
All authors participated in the writing of the manuscript.
Availability of data and materials
Not applicable.
Financial support and sponsorship
This work was partially supported by the “Key Project of Science and Technology Research Program of Hubei Provincial Department of Education” [F2023008], the “Youth Project of Natural Science Foundation of Hubei Province” [Grant No. 2023AFB224], and the “Science Foundation of Wuhan Institute of Technology” [Grant No. 22QD29].
Conflicts of interest
Prof. Huang, L. is Guest Editor of the Special Issue Structural Regulation and Application of Two-Dimensional Metal Oxide of the journal Microstructures; Prof. Yu, Y. and Prof. Xiong, L. are Assistant Guest Editors of the Special Issue of the journal Microstructures. They were not involved in any steps of editorial processing, notably including reviewer selection, manuscript handling, or decision-making, while the other authors have declared that they have no conflicts of interest.
Ethical approval and consent to participate
Not applicable.
Consent for publication
Not applicable.
Copyright
© The Author(s) 2025.
REFERENCES
1. Gusain, R.; Gupta, K.; Joshi, P.; Khatri, O. P. Adsorptive removal and photocatalytic degradation of organic pollutants using metal oxides and their composites: a comprehensive review. Adv. Colloid. Interface. Sci. 2019, 272, 102009.
2. Mei, J.; Liao, T.; Kou, L.; Sun, Z. Two-dimensional metal oxide nanomaterials for next-generation rechargeable batteries. Adv. Mater. 2017, 29, 1700176.
3. Zhu, Y.; Peng, L.; Fang, Z.; Yan, C.; Zhang, X.; Yu, G. Structural engineering of 2D nanomaterials for energy storage and catalysis. Adv. Mater. 2018, 30, e1706347.
4. Garland, B. M.; Fairley, N.; Strandwitz, N. C.; Thorpe, R.; Bargiela, P.; Baltrusaitis, J. A study of in situ reduction of MoO3 to MoO2 by X-ray photoelectron spectroscopy. Appl. Surf. Sci. 2022, 598, 153827.
5. de Castro, I. A.; Datta, R. S.; Ou, J. Z.; et al. Molybdenum oxides - from fundamentals to functionality. Adv. Mater. 2017, 29, 1701619.
6. Zou, B.; Wang, X.; Zhou, Y.; et al. Optical effect modulation in polarized raman spectroscopy of transparent layered α-MoO3. Small 2023, 19, e2206932.
7. Ye, M.; Qiang, B.; Zhu, S.; et al. Nano-optical engineering of anisotropic phonon resonances in a hyperbolic α-MoO3 metamaterial. Adv. Opt. Mater. 2022, 10, 2102096.
8. Tao, S.; Hou, T.; Zeng, Y.; et al. Anisotropic Fermat’s principle for controlling hyperbolic van der Waals polaritons. Photon. Res. 2022, 10, B14.
9. Wang, Y.; Guo, X.; You, S.; et al. Giant quartic-phonon decay in PVD-grown α-MoO3 flakes. Nano. Res. 2023, 16, 1115-22.
10. Zheng, M.; Liu, P.; Yan, P.; et al. Heterogeneous CNF/MoO3 nanofluidic membranes with tunable surface plasmon resonances for solar-osmotic energy conversion. Mater. Horiz. 2024, 11, 3375-85.
11. Kong, W.; Liu, W.; Zheng, X.; Xu, Q. Sunlight driven reversible and tunable plasmon resonance in 2D amorphous molybdenum oxide. Adv. Opt. Mater. 2024, 12, 2301821.
12. Hu, G.; Ou, Q.; Si, G.; et al. Topological polaritons and photonic magic angles in twisted α-MoO3 bilayers. Nature 2020, 582, 209-13.
13. Wang, S. Y.; Li, D. K.; Zha, M. J.; Yan, X. Q.; Liu, Z.; Tian, J. Tunable optical activity in twisted anisotropic two-dimensional materials. ACS. Nano. 2023, 17, 16230-8.
14. Duan, J.; Álvarez-Pérez, G.; Lanza, C.; et al. Multiple and spectrally robust photonic magic angles in reconfigurable α-MoO3 trilayers. Nat. Mater. 2023, 22, 867-72.
15. Liu, H.; Yu, K.; Zhang, K.; Ai, Q.; Xie, M.; Wu, X. Effect of substrate on the near-field radiative heat transfer between α-MoO3 films. Int. J. Heat. Mass. Transf. 2023, 210, 124206.
16. Li, L.; Wu, X.; Liu, H.; Shi, K.; Liu, Y.; Yu, K. Near-field radiative modulator based on α-MoO3 films. Int. J. Heat. Mass. Transfer. 2023, 216, 124603.
17. Ren, H.; Sun, S.; Cui, J.; Li, X. Synthesis, functional modifications, and diversified applications of molybdenum oxides micro-/nanocrystals: a review. Cryst. Growth. Des. 2018, 18, 6326-69.
18. Scanlon, D. O.; Watson, G. W.; Payne, D. J.; Atkinson, G. R.; Egdell, R. G.; Law, D. S. L. Theoretical and experimental study of the electronic structures of MoO3 and MoO2. J. Phys. Chem. C. 2010, 114, 4636-45.
19. Huang, C.; Zhang, W.; Zheng, W. The debut and spreading the landscape for excellent vacancies-promoted electrochemical energy storage of nano-architected molybdenum oxides. Mater. Today. Energy. 2022, 30, 101154.
20. Xie, H.; Li, Z.; Cheng, L.; et al. Recent advances in the fabrication of 2D metal oxides. iScience 2022, 25, 103598.
21. Kuriakose, S.; Kayani, A. B. A.; Monshipouri, M.; et al. Customized two-dimensional nanostructured MoO3 inks for spectrally selective UV chromic patches. ACS. Appl. Nano. Mater. 2022, 5, 18553-60.
22. Wei, Z.; Gasparyan, M.; Liu, L.; et al. Microwave-exfoliated 2D oligo-layer MoO3-x nanosheets with outstanding molecular adsorptivity and room-temperature gas sensitivity on ppb level. Chem. Eng. J. 2023, 454, 140076.
23. Meng, X.; Yu, J.; Shi, W.; et al. SERS detection of trace carcinogenic aromatic amines based on amorphous MoO3 monolayers. Angew. Chem. Int. Ed. 2024, 63, e202407597.
24. Wu, T.; Zhang, F.; Wang, J.; Liu, X.; Tian, Y.; Chu, K. Electrochemical reduction of nitrite to ammonia on amorphous MoO3 nanosheets. Dalton. Trans. 2024, 53, 877-81.
25. Ou, Y.; Zhou, Y.; Guo, Y.; et al. 2D/2D Dy2O3 nanosheet/MoO3 nanoflake heterostructures for humidity-independent and sensitive ammonia detection. ACS. Sens. 2023, 8, 4253-63.
26. Pradeep Kumar, P.; Singh, V. Enhanced dual gas sensing performance of MoS2/MoO3 nanostructures for NH3 and NO2 detection. Ceram. Int. 2024, 50, 21978-88.
27. Wang, Z. Y.; Yuan, B.; Zhang, F. G.; et al. Photocatalytic nitrogen fixation coupled with the generation of value-added chemicals from N2 and cellulose over MoO3 nanosheets. Inorg. Chem. 2024, 63, 9715-9.
28. Ding, W.; Li, X.; Su, S.; et al. In-situ construction of Schottky junctions with synergistic interaction of oxygen vacancies in Mo@MoO3 nanosheets for efficient N2 photoreduction. Appl. Surf. Sci. 2023, 633, 157594.
29. Xiao, R.; Wang, T.; Feng, S.; et al. Porous MoO3 nanosheets for conductometric gas sensors to detect diisopropylamine. Sens. Actuators. B. Chem. 2023, 382, 133472.
30. Madani, S.; Tesfamichael, T.; Motta, N.; Wang, H. Simulation of perovskite solar cells using molybdenum oxide thin films as interfacial layer for enhancing device performance. Sustain. Mater. Technol. 2022, 32, e00426.
31. Du, Y.; Li, G.; Peterson, E. W.; et al. Iso-oriented monolayer α-MoO3(010) films epitaxially grown on SrTiO3(001). Nanoscale 2016, 8, 3119-24.
32. Bisht, P.; Kumar, A.; Jensen, I. T.; Ahmad, M.; Belle, B. D.; Mehta, B. Enhanced gas sensing response for 2D α-MoO3 layers: thickness-dependent changes in defect concentration, surface oxygen adsorption, and metal-metal oxide contact. Sens. Actuators. B. Chem. 2021, 341, 129953.
33. Zhao, X.; Chu, Q.; Guo, S.; et al. Controllable hot electron transfer in the Ag/MoO3 layer by layer system: thickness-dependent MoO3 layer. Spectrochim. Acta. A. Mol. Biomol. Spectrosc. 2023, 286, 121995.
34. Rahman, M.; Chetri, S.; Pemmaraju, D. B.; Murty, U. S.; Deshpande, U. P.; Kakati, M. An expanded plasma jet assisted technique for very high-rate synthesis of 2D α-MoO3 nanomaterials, with surface oxygen vacancies and robust induced ferromagnetism. Vacuum 2024, 225, 113237.
35. Hong, Y.; Lan, S.; Pan, B.; et al. Thinner 2D α-MoO3 makes setting up memristors easier. J. Materiomics. 2024, 10, 1279-89.
36. Deng, L.; Zhang, Q.; Li, W.; et al. KCl acts as a flux to assist the growth of sub-millimeter-scale metallic 2D non-layered molybdenum dioxide. Rare. Met. 2025, 44, 404-16.
37. Xu, H.; Karbalaei, A. M.; Wang, S.; et al. Tunability of near infrared opto-synaptic properties of thin MoO3 films fabricated by atomic layer deposition. Appl. Surf. Sci. 2022, 593, 153399.
38. Kim, Y. W.; Park, J.; Park, J. H.; et al. Self-isolating electrode deposition process using the area-selective MoO2 and MoO3 atomic layer deposition technique. Appl. Mater. Today. 2024, 37, 102160.
39. Lee, D. J.; Kumar, G. M.; Kim, Y.; et al. Hybrid CsPbBr3 quantum dots decorated two dimensional MoO3 nanosheets photodetectors with enhanced performance. J. Mater. Res. Technol. 2022, 18, 4946-55.
40. Kundu, M.; Mondal, D.; Mondal, I.; et al. A rational preparation strategy of phase tuned MoO3 nanostructures for high-performance all-solid asymmetric supercapacitor. J. Energy. Chem. 2023, 87, 192-206.
41. Zhu, Y.; Tan, Y.; Li, H. MoO3 nanoplates preparation via self-sacrifice C3N4 for supercapacitors in an acid electrolyte. J. Energy. Storage. 2023, 60, 106657.
42. Alam, M. H.; Chowdhury, S.; Roy, A.; et al. Wafer-scalable single-layer amorphous molybdenum trioxide. ACS. Nano. 2022, 16, 3756-67.
43. Liu, B.; Wu, S.; Lv, Y.; et al. Facile synthesis of oxygen-deficient MoO3-x nanosheets by light radiation for fast electrochromic supercapacitors. Electrochim. Acta. 2023, 464, 142894.
44. Qi, S.; Liu, G.; Zhang, K.; Chen, J.; Zhao, Y.; Lou, Y. Colloidal synthesis of plasmonic ultrathin transition-metal oxide nanosheets. ACS. Sustain. Chem. Eng. 2022, 10, 9565-72.
45. Shkir, M.; Ben, G. T. A.; Alkallas, F. H.; Alfaify, S. High performance of the rare earth (Er, Gd & Pr) doped MoO3 thin films for advanced applications towards ammonia gas sensing. J. Mater. Res. Technol. 2022, 20, 4556-65.
46. Li, T.; Zhu, L.; Lu, L.; et al. Highly sensitive optical fiber plasmonic sensors by integrating hydrogen doped molybdenum oxide. IEEE. Sensors. J. 2022, 22, 7734-42.
47. Li, W.; Xu, H.; Wang, A.; et al. Insitu controllable synthesis of MoO3 nanoflakes and its temperature-dependent dual selectivity for detection of ethanol and isopropanol. Sens. Actuators. B. Chem. 2024, 408, 135548.
48. Kriegel, I.; Jiang, C.; Rodríguez-Fernández, J.; et al. Tuning the excitonic and plasmonic properties of copper chalcogenide nanocrystals. J. Am. Chem. Soc. 2012, 134, 1583-90.
49. Li, C.; Jang, H.; Kim, M. G.; Hou, L.; Liu, X.; Cho, J. Ru-incorporated oxygen-vacancy-enriched MoO2 electrocatalysts for hydrogen evolution reaction. Appl. Catal. B. Environ. 2022, 307, 121204.
50. Luo, Z.; Miao, R.; Huan, T. D.; et al. Mesoporous MoO3-x material as an efficient electrocatalyst for hydrogen evolution reactions. Adv. Energy. Mater. 2016, 6, 1600528.
51. Cheng, H.; Kamegawa, T.; Mori, K.; Yamashita, H. Surfactant-free nonaqueous synthesis of plasmonic molybdenum oxide nanosheets with enhanced catalytic activity for hydrogen generation from ammonia borane under visible light. Angew. Chem. Int. Ed. 2014, 53, 2910-4.
52. Sun, Y.; Cui, J.; Wang, C.; Fu, S.; Sun, S.; Wang, X. Controllable synthesis of defect-enriched MoO3 for enhanced H2S sensing through hydrothermal methods: experiments and DFT calculations. J. Alloys. Compd. 2023, 968, 172035.
53. Sun, J.; Qin, S.; Zhao, Z.; Zhang, Z.; Meng, X. Rapid carbothermal shocking fabrication of iron-incorporated molybdenum oxide with heterogeneous spin states for enhanced overall water/seawater splitting. Mater. Horiz. 2024, 11, 1199-211.
54. Xu, L.; Zhou, W.; Chao, S.; et al. Advanced oxygen-vacancy Ce-doped MoO3 ultrathin nanoflakes anode materials used as asymmetric supercapacitors with ultrahigh energy density. Adv. Energy. Mater. 2022, 12, 2200101.
55. Lin, Y.; Zhang, Y.; Magomedov, A.; et al. 18.73% efficient and stable inverted organic photovoltaics featuring a hybrid hole-extraction layer. Mater. Horiz. 2023, 10, 1292-300.
56. Wang, H.; Guan, N.; Feng, Z.; Xiang, W.; Zhao, H.; Zhang, X. Constructing defect engineered 2D/2D MoO3/g-C3N4 Z-scheme heterojunction for enhanced photocatalytic activity. J. Alloys. Compd. 2022, 926, 166964.
57. Peng, J.; Shen, J.; Yu, X.; Tang, H.; Zulfiqar; Liu, Q. Construction of LSPR-enhanced 0D/2D CdS/MoO3-x S-scheme heterojunctions for visible-light-driven photocatalytic H2 evolution. Chin. J. Catal. 2021, 42, 87-96.
58. Wei, Y.; Zhang, Q.; Zhou, Y.; et al. Noble-metal-free plasmonic MoO3-x-based S-scheme heterojunction for photocatalytic dehydrogenation of benzyl alcohol to storable H2 fuel and benzaldehyde. Chin. J. Catal. 2022, 43, 2665-77.
59. Wang, H.; Liu, Q.; Xu, M.; et al. Dual-plasma enhanced 2D/2D/2D g-C3N4/Pd/MoO3-x S-scheme heterojunction for high-selectivity photocatalytic CO2 reduction. Appl. Surf. Sci. 2023, 640, 158420.
60. Yoon, A.; Kim, J. H.; Yoon, J.; Lee, Y.; Lee, Z. van der Waals epitaxial formation of atomic layered α-MoO3 on MoS2 by oxidation. ACS. Appl. Mater. Interfaces. 2020, 12, 22029-36.
61. Krishnan, A.; Kamaraj, M.; Nayak, P. K.; Ramaprabhu, S. Surface functionalized 2D MoO3-hBN heterostructure as friction modifiers in boundary lubrication regime. Surf. Interfaces. 2024, 51, 104527.
62. Wang, Q.; Tian, H.; Zhang, Z.; et al. Keggin-type polycationic AlO4Al12(OH)24(H2O)127+ intercalated MoO3 composites for methyl orange adsorption. Chin. Chem. Lett. 2022, 33, 2617-20.
63. Tan, Y.; He, J.; Wang, B.; Li, C. C.; Wang, T. Tuning the layer structure of molybdenum trioxide towards high-performance aqueous zinc-ion batteries. Chin. Chem. Lett. 2023, 34, 107410.
64. Gao, Z.; Tan, R.; Pan, Z.; et al. Boosting ammonia synthesis over MoO2 by Li intercalation. Green. Chem. 2022, 24, 7584-91.
65. Reed, B. W.; Chen, E.; Koski, K. J. Tunable chemochromism and elastic properties in intercalated MoO3: Au-, Cr-, Fe-, Ge-, Mn-, and Ni-MoO3. ACS. Nano. 2024, 18, 12845-52.
66. Hu, T.; Xue, B.; Meng, F.; et al. Preparation of 2D Polyaniline/MoO3-x superlattice nanosheets via intercalation-induced morphological transformation for efficient chemodynamic therapy. Adv. Healthc. Mater. 2023, 12, e2202911.
67. Xie, S.; Cheng, X.; Hu, C.; et al. Pressure effect on structure transition and optical anisotropy in MoO3. Appl. Phys. Lett. 2022, 120, 131901.
68. Wang, S.; Wang, Y.; Liu, T.; Wang, L.; Huang, Y.; Lu, Y. Irreversible pressure effect on phase transitions and bandgap narrowing of layered MoO3. Mater. Today. Adv. 2024, 21, 100476.
69. Chia, X.; Pumera, M. Characteristics and performance of two-dimensional materials for electrocatalysis. Nat. Catal. 2018, 1, 909-21.
70. Wen, K.; Huang, L.; Qu, L.; et al. g-C3N4/MoO3 composite with optimized crystal face: a synergistic adsorption-catalysis for boosting cathode performance of lithium-sulfur batteries. J. Colloid. Interface. Sci. 2023, 649, 890-9.
71. Hsu, F. H.; Hsu, S. Y.; Subramani, R.; et al. The ion behavior and storage mechanism of 2D MoO3 layer structure in an air-stable hydrated eutectic electrolyte for aluminum-ion energy storage. J. Energy. Storage. 2024, 84, 110693.
72. Hao, L.; Huang, H.; Zhang, Y.; Ma, T. Oxygen vacant semiconductor photocatalysts. Adv. Funct. Mater. 2021, 31, 2100919.
73. Ashraf, G. A.; Rasool, R. T.; Rasool, R. U.; et al. Photocatalytic stimulation of peroxymonosulfate by novel MoO3@ZrO2 with Z-scheme heterojunction for diclofenac sodium degradation. J. Water. Process. Eng. 2023, 51, 103435.
74. Wang, J.; Tao, J.; Dong, X.; et al. Hydrothermal oxygen uncoupling of high-concentration biogas slurry over Cu-α-Fe2O3·α-MoO3 catalyst. J. Environ. Manag. 2022, 320, 115827.
75. Zhao, X.; Zhou, W.; Xu, L.; et al. Oxygen-vacancy Ce-MoO3 nanosheets loaded Pt nanoparticles for super-efficient photoelectrocatalytic oxidation of methanol. Appl. Surf. Sci. 2024, 655, 159576.
76. Zhu, Y.; Lin, Q.; Zhong, Y.; Tahini, H. A.; Shao, Z.; Wang, H. Metal oxide-based materials as an emerging family of hydrogen evolution electrocatalysts. Energy. Environ. Sci. 2020, 13, 3361-92.
77. Wang, X.; Liu, G.; Zhang, D.; et al. N-doped carbon sheets supported P-Fe3O4-MoO2 for freshwater and seawater electrolysis. J. Colloid. Interface. Sci. 2023, 652, 1217-27.
78. Li, J.; Cheng, Y.; Zhang, J.; Fu, J.; Yan, W.; Xu, Q. Confining Pd nanoparticles and atomically dispersed Pd into defective MoO3 nanosheet for enhancing electro- and photocatalytic hydrogen evolution performances. ACS. Appl. Mater. Interfaces. 2019, 11, 27798-804.
79. Deka, H.; Sunaniya, A. K.; Agarwal, P. Simulation studies on MoS2 (n)/a-Si:H (i)/c-Si (p)/MoO3 heterojunction solar cells using one sided short diode approximation. Solar. Energy. 2023, 263, 111943.
80. Li, J.; Kang, Q.; Wang, Y.; et al. Low oxygen content MoOx and SiOx tunnel layer based heterocontacts for efficient and stable crystalline silicon solar cells approaching 22% efficiency. Adv. Funct. Mater. 2024, 34, 2310619.
81. Suzuki, I.; Lin, Z.; Nogami, T.; et al. High open-circuit voltage in single-crystalline n -type SnS/MoO3 photovoltaics. APL. Mater. 2023, 11, 031116.
82. Wang, J.; Zheng, Z.; Zu, Y.; et al. A tandem organic photovoltaic cell with 19.6% efficiency enabled by light distribution control. Adv. Mater. 2021, 33, e2102787.
83. Liu, Z.; Zhang, H.; Sun, L. Enhanced performance of polymer solar cells by ultraviolet-ozone treatment of MoOx films with non-thermal annealing treatment of MoOx films. Surf. Interfaces. 2024, 51, 104701.
84. Jiang, W.; Su, M.; Zheng, Y.; Fei, T. Efficient electron transfer through interfacial water molecules across two-dimensional MoO3 for humidity sensing. ACS. Appl. Mater. Interfaces. 2024, 16, 7406-14.
85. Zhang, W.; Li, H.; Hopmann, E.; Elezzabi, A. Y. Nanostructured inorganic electrochromic materials for light applications. Nanophotonics 2020, 10, 825-50.
86. Gao, G.; Tao, X.; He, Y.; et al. Electrochromic composites films composed of MoO3 doped by tungsten atoms with remarkable response speed and color rendering efficiency via electrochemical deposition. Appl. Surf. Sci. 2023, 640, 158346.
87. Kowalczyk, D. A.; Rogala, M.; Szałowski, K.; et al. Two-dimensional crystals as a buffer layer for high work function applications: the case of monolayer MoO3. ACS. Appl. Mater. Interfaces. 2022, 14, 44506-15.
88. Yang, Z.; Guo, C.; Qin, L.; et al. Enhanced organic thin-film transistor stability by preventing MoO3 diffusion with metal/MoO3/organic multilayered interface source-drain contact. ACS. Appl. Mater. Interfaces. 2023, 15, 1704-17.
89. Shan, X.; Liu, P.; Wang, F.; et al. Dual-conductivity mechanism investigation of 2D α-MoO3-based multi-level memristor. Sci. China. Mater. 2023, 66, 4773-81.
90. Ma, Y.; Lang, J. 2D SnO/MoO3 van der Waals heterojunction with tunable electronic behavior for multifunctional applications: DFT calculations. App. Surf. Sci. 2023, 611, 155719.
91. Surnev, S.; Netzer, F. P. Tungsten and molybdenum oxide nanostructures: two-dimensional layers and nanoclusters. J. Phys. Condens. Matter. 2022, 34, 233001.
92. Petronijevic, E.; Dereshgi, S. A.; Larciprete, M. C.; Centini, M.; Sibilia, C.; Aydin, K. Extrinsic chirality and circular dichroism at visible frequencies enabled by birefringent α-MoO3 nanoscale-thick films: implications for chiro-optical control. ACS. Appl. Nano. Mater. 2022, 5, 5609-16.
93. Kundu, M.; Mondal, D.; Bose, N.; Basu, R.; Das, S. 2D MoO3/PVDF-HFP nanocomposites for flexible piezoelectric nanogenerator and wireless mechanosensor applications. ACS. Appl. Nano. Mater. 2024, 7, 1804-14.
Cite This Article

How to Cite
Download Citation
Export Citation File:
Type of Import
Tips on Downloading Citation
Citation Manager File Format
Type of Import
Direct Import: When the Direct Import option is selected (the default state), a dialogue box will give you the option to Save or Open the downloaded citation data. Choosing Open will either launch your citation manager or give you a choice of applications with which to use the metadata. The Save option saves the file locally for later use.
Indirect Import: When the Indirect Import option is selected, the metadata is displayed and may be copied and pasted as needed.
About This Article
Copyright
Data & Comments
Data
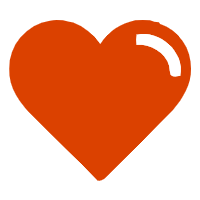
Comments
Comments must be written in English. Spam, offensive content, impersonation, and private information will not be permitted. If any comment is reported and identified as inappropriate content by OAE staff, the comment will be removed without notice. If you have any queries or need any help, please contact us at [email protected].