Enabling highly reversible Zn anode via an interfacial preferentially adsorbed additive containing nucleophilic groups
Abstract
The cyclability and reversibility of aqueous zinc-ion batteries (AZIBs) are severely hampered by the safety concerns arising from the Zn dendrite growth. Therefore, a stable anode with inhibited dendrites and side reactions is crucial for AZIBs. Herein, we utilized methyl acetoacetate (MA) as an additive to prevent dendrite growth and enable highly reversible Zn anodes. Benefiting from the nucleophilic groups (carbonyl groups) in MA, MA molecules can preferentially adsorb on the anode/electrolyte interface (AEI), forming a molecular protective layer. Such MA layers can not only regulate the migration and deposition of zinc ions, but also inhibit side reactions induced by the decomposition of free H2O molecules at AEI. Therefore, the symmetric cell with the addition of MA achieves a long-term cycling stability of 1,500 h at 2 mA cm-2 with a capacity of 2 mAh cm-2. In addition, the Zn//NVO full cell using MA-contained electrolyte demonstrates a high specific capacity (138.4 mAh g-1) with an outstanding capacity retention (92.8% after 600 cycles) at 1 A g-1. This work provides a principle for the use of ester-based additives with nucleophilic groups to suppress Zn dendrite growth for highly durable zinc metal anodes.
Keywords
INTRODUCTION
Currently, constructing a cleaner and more efficient society requires greater utilization of renewable energy sources to replace fossil fuels[1-3]. Due to the unstable nature of renewable energy, there is an urgent need to develop large-scale electrochemical energy storage systems (ESS) to cope with grid fluctuations[4-7]. Considering the strict requirements for safety and economy, aqueous zinc-ion batteries (AZIBs) are considered the most promising alternatives for grid energy storage. The Zn anode possesses core advantages including environmental friendliness, low cost, high energy density (820 mAh g-1), and appropriate redox potential (-0.76 V vs. the standard hydrogen electrode)[8-11]. However, the cycle lifespan of the zinc metal anode is seriously insufficient to satisfy the demands of commercial application, which is owing to the numerous complex reactions occurring at the interface of zinc metal anode in aqueous electrolytes[12,13]. It is well known that during electrodeposition, the Zn anode undergoes uneven initial nucleation of zinc. Under the “tip effect”, zinc dendrites grow rapidly, eventually piercing the separator and causing a sudden short circuit[14-16]. In addition, the Zn anode is in a thermodynamically unstable state in aqueous solutions, where active water molecules in the electrolyte can compete with Zn2+ cations to capture electrons, leading to a hydrogen evolution reaction (HER)[17-19]. The occurrence of HER is accompanied by a local increase in pH at the anode surface, which in turn leads to surface hydroxide sulfate passivation. Dendrite growth and parasitic side reactions resonate with each other, severely limiting the practicality of AZIBs as a feasible and effective ESS.
The deposition morphology and uniformity of zinc are determined by the deposition/dissolution behavior of Zn[20,21]. Altering the chemical environment at the surface of zinc metal and regulating the distribution and transport of Zn2+ at the anode/electrolyte interface (AEI) are key to continuously optimizing the morphological evolution of the zinc metal anode[22-24]. Unlike traditional methods of constructing an artificial solid electrolyte interface (SEI) on zinc metal to regulate the deposition kinetics of Zn2+, the use of electrolyte optimization strategies has been proven to be a simple and direct approach to control the deposition behavior of Zn2+[25,26]. Some organic compounds containing polar groups, such as alcohols, ketones, and ethers, can optimize the deposition behavior of zinc and suppress side reactions by altering the solvation structure of zinc ions or reconstructing the hydrogen bonding network in the electrolyte[27,28]. Recently, ester-based organic molecules, due to their strong adsorption capacity for zinc, have been used to regulate the interfacial chemistry of the zinc metal anode[29]. For example, Wang et al. used the interaction of trimethyl phosphate (TMP) molecules to increase the electron density of water protons, thereby suppressing water activity and enabling symmetrical cells to operate for up to 1,600 h[3]. Liu et al. constructed a mixture solvent using propylene carbonate (PC) and aqueous solution, effectively reducing the free water molecules in the Zn2+-solvent cations, enabling the full cells to exhibit a high specific capacity of 183 mAh g-1 over 300 cycles[30]. However, current research on ester components mostly involves adding them as co-solvents to aqueous electrolytes, which significantly increases the cost of the electrolyte and decreases the ionic conductivity. Therefore, exploring the use of ester molecules in trace addition, and elucidating the underlying mechanisms by which ester molecules regulate the zinc deposition process is crucial for the application of ester organic compounds in AZIBs.
In this study, we present methyl acetoacetate (MA), a water-soluble ester, as a novel additive for AZIBs to improve the stability and reversibility of zinc electrodes. The calculated and experimental results indicate that the MA molecule possesses two oxygen atoms with high charge density, which can preferentially adsorb at AEI, effectively controlling the nucleation and diffusion processes of zinc ions. This molecular adsorption layer also promotes the growth of the preferred Zn (002) crystal facet and limits the generation of zinc dendrites. Furthermore, the adsorbed MA molecules create an electrical double layer (EDL) with reduced water content at AEI. This unique water-poor EDL prevents direct contact between zinc foils and active H2O, restraining the Zn corrosion reaction [Scheme 1]. As a consequence, modulated Zn deposition behaviors by MA additives greatly prolong the cycling lifespan of symmetric cells over 1,500 h (2 mA cm-2 with a capacity of 2 mAh cm-2) and the Zn//Cu cell achieves stable cycling for 1,400 cycles at 2 mA cm-2. Moreover, at 1 A g-1, the Zn//NVO full cell using MA exhibits an improved reversible specific capacity retention of 92.8% after 600 cycles. This research offers a guideline of utilizing ester-based additives to enable highly reversible Zn anodes for high-performance AZIBs.
MATERIALS AND METHODS
Electrolyte preparation
The process of making an electrolyte includes dissolving ZnSO4·7H2O (99.995%, Aladdin) in deionized (DI) water to prepare a solution of 2 M ZnSO4. To produce an electrolyte with MA, MA of 99% purity from Aladdin was incorporated into the 2 M ZnSO4 solution. Additionally, Zn(CF3SO3)2 (98.0%, Aladdin) was utilized for the full cells.
Synthesis of cathode Na5V12O32 (NVO) powders
First, 1.819 g V2O5 and 0.400 g NaOH were dissolved into 40 mL DI water and the mixed solution was stirred continuously for 30 min. Following that, the mixture was introduced into a 50 mL pressure vessel and maintained at a temperature of 180 °C for a duration of 48 h. The precursors were collected after repeatedly washing and centrifuging, and dried at 80 °C under vacuum conditions for 12 h. The precursors were heated in air for 2 h at 300 °C to finally obtain Na5V12O32 (NVO) powder.
Material characterization
Scanning electron microscopy (SEM) images of sample morphology were analyzed via TESCAN. The morphology of the surface of the anodes after cycling was examined using laser confocal microscopy (KEYENCE VK-X150). Raman microscopy (Thermo Scientific DXR3) using 532 nm lasers, while the Smartlab SE instrument was used for X-ray diffraction (XRD) analysis with a step size of 0.02° to check the crystal structure. X-ray photoelectron spectroscopy (XPS) tests were conducted to study the valence state of each element (Thermo Scientific K-Alpha). The contact angles of various electrolytes on the electrode surface are measured using a contact angle tester (DINGSHENG JY-82C).
Electrochemical characterization
The assembly of the cells was conducted in CR2025 coin-type cells under ambient air conditions. The glass fiber separator (Whatman, GF-D) was employed. The electrolyte for both asymmetric and symmetric cells was a standard 2 M aqueous solution of ZnSO4. The full cell utilized a 2 M Zn(CF3SO3)2 electrolyte. The NEWARE battery-testing system (MIHW-200-160CH-B, Shenzhen, China) was used to perform extended cycling and rate capability tests at an ambient temperature of 25 °C. Electrochemical characterization, including impedance spectroscopy [electrochemical impedance spectroscopy (EIS)] spanning from 100 kHz to 0.1 Hz and cyclic voltammetry (CV), was conducted with an electrochemical workstation (Ivium, Netherlands). For cathode fabrication, N-methylpyrrolidone (NMP) served as the solvent. The cathode material, polyvinylidene fluoride and carbon black were mixed in a weight ratio of 7:2:1 using NMP as a solvent to create a slurry. This mixture was evenly coated onto a 20 µm thick titanium steel foil. Following the coating process, the foil underwent drying at 80 °C for a period of 12 h.
Simulation method
The electrostatic potential (ESP) and binding energy were calculated using the Gaussian 09 software. The geometries of the molecules were optimized employing the B3LYP hybrid functional in conjunction with the 6-31G+ basis sets. The ESP diagrams were generated using Multiwfn 3.8 software. Atomic models and ESP diagrams were visualized with VMD19 software, utilizing an iso-surface value of 0.03 for clarity. In addition, the DFT-D3 dispersion correction methods were implemented to accurately represent the subtle interactions from cations to anions.
The computational tool VASP was utilized to determine the charge distribution and the density of states (DOS) for the studied systems, adhering to the principles of density functional theory (DFT). The generalized gradient approximation (GGA), specifically the Perdew-Burke-Ernzerhof (PBE) functional, was applied to calculate the exchange-correlation energy. A plane-wave basis set with an energy cutoff of 450 eV was implemented to achieve accurate self-consistent charge density calculations. The convergence criterion for atomic forces was set at 0.01 eV Å-1, which is crucial for determining the equilibrium geometries. The surface slab model was meticulously constructed, incorporating a generous vacuum space of
where Ea or Eb is the energy of Zn bulk and adsorbent, and Etotal is the total energy of the system.
RESULTS AND DISCUSSION
The molecular structure of MA with two nucleophilic carbonyl groups is shown in Figure 1A. The surface ESP is utilized to investigate the contribution of MA molecules to electrochemical processes. As displayed in Figure 1B, the results evidence that electronegative carbonyl oxygen atoms in MA molecules exhibit more negative ESP value than other atoms, suggesting that zinc metal anode and positively charged Zn2+ ions interact more strongly with nucleophilic carbonyl groups on MA. This interaction favors the adsorption of MA onto the zinc foil surface in the electrolyte, thus modulating the distribution, transmission, and deposition dynamics of Zn2+ at the AEI. To explore the adsorption behavior of MA on zinc anode, the Eads of MA molecules, Zn atoms and H2O molecules on the Zn (002) crystal plane was investigated via DFT calculations [Figure 1C], and the corresponding optimized structures are presented in Figure 1D. MA molecules demonstrate an Eads of -3.56 eV on the Zn (002) plane, which is much lower than that of Zn atoms (-2.79 eV) and H2O molecules (-3.02 eV), proving the domain occupation of MA at AEI, which manipulates the kinetics of Zn2+ ions and reduces the proportion of adsorbed water molecule. In addition, the elemental composition and functional groups on the electrode reveal the adsorption of MA in XPS characterization and Raman spectrum. Supplementary Figure 1 shows the full survey spectra of the zinc foils soaked in different electrolytes for five days. In the high-resolution C 1s spectra [Figure 1E], C-C, C-O and C=O species can be detected for the zinc metal anode soaked in the solution with MA. Besides, the O 1s spectra in Figure 1F show a higher proportion of characteristic peaks of C=O and C-O bonds, which verify the presence of MA molecules on the anode surface. As depicted in the Raman spectra [Figure 1G], the characteristic peaks of MA can be observed for the zinc foil soaked in MA-contained electrolyte, where the
Figure 1. (A) Molecular structure and (B) electrostatic potential mapping of MA. (C) The adsorption energy of the zinc atom, water molecule and MA molecule on Zn (002) crystal plane. The corresponding structures of the (D) MA molecule, Zn atom and water molecule adsorbed on zinc. (E) C 1s, (F) N 1s XPS spectrum, and (G) Raman spectra of zinc foils immersed in electrolyte with/without MA for 5 days.
Because an electrolyte with 10 mM MA can maximize the cycling life of symmetric cells, this concentration of MA additives was selected as the optimal condition for the optimized electrolyte
Figure 2. (A) Contact angle measurements of different electrolytes on zinc metal foil. (B) Nucleation overpotential at 1 mA cm-2 using different electrolytes. (C) CV curves of symmetric cells in different electrolytes. CE of asymmetric cells in different electrolytes at (D)
We believe that the nucleophilic carbonyl groups in MA act as an excellent hydrogen bond acceptor. Consequently, when MA molecules are adsorbed onto the Zn electrode surface, they can establish a
Figure 3. (A-C) FTIR spectra of different electrolytes. (D) LSV curves using NaSO4 solutions with/without MA. (E) Optical images, corresponding SEM images and (F) XRD patterns of zinc electrodes after 50 cycles using various electrolytes. (G) Electrochemical performance of the symmetric cells using different electrolytes when enduring alternate cycling and resting process.
Benefiting from the significant role of MA in stabilizing the zinc metal electrode, the enhanced electrochemical performance of cells using MA-contained electrolyte can be expected. When cycled at
Figure 4. (A) Current-voltage profiles of the Zn//Zn symmetrical batteries with different electrolytes at 5 mA cm-2 with
The enhanced electrochemical reversibility means an outstanding improvement in the stability of zinc electrodes, and also proves that the growth process of zinc dendrites is inhibited. The macroscale images of zinc metal deposition in different electrolytes are depicted in Figure 5A-F, showcasing a pronounced difference in the deposition morphology. In addition, the 3D confocal laser scanning microscopy (CLSM) images indicate that the Zn anode cycled in the MA-free electrolyte has a rough surface with high average surface roughness. Conversely, the anode cycled in the MA-containing electrolyte shows a markedly smoother and more compact surface, with a significantly lower average surface roughness. Furthermore, the Zn//NVO full batteries were assembled with electrolytes without/with MA to evaluate the practical application potential. Attributed to the enhanced reversibility of the zinc electrode in the MA-contained electrolyte, the Zn//NVO full cell delivers outstanding cycling stability with a high specific capacity of
Figure 5. CLSM images and the corresponding surface roughness curve of the Zn electrodes after fifty cycles in electrolytes (A-C) without and (D-F) with MA addition. Electrochemical performance of Zn//NVO full cells using electrolytes with/without MA: (G) Cycling performance at 1 A g-1; (H) Selected GCD curves; (I) EIS curves; (J) SEM images of the anodes of full batteries after 50 cycles.
CONCLUSIONS
To summarize, we utilized MA with nucleophilic groups as an interface stabilizer to enhance the cycling performance of the Zn anode. Combining the calculation and experiment results, MA molecules preferentially adsorb on the Zn/electrolyte interface, owing to their strong affinity to the zinc metal anode. Moderate electron-withdrawing properties of carbonyl groups allow them to redistribute the charge, thereby modulating the nucleation and growth of Zn2+, which leads to the achievement of a uniform and compact Zn deposition layer[53]. Moreover, the adsorbed MA molecules establish a H2O-poor EDL structure which diminishes side reactions and surface passivation at the AEI due to the decomposition of active water. Consequently, the symmetric cell using MA-contained electrolyte delivers an enhanced stability of
DECLARATIONS
Authors’ contributions
Materials synthesis, electrochemical experiments, data collection and analysis: Li, C.
Data acquisition, visualization, and investigation: Qi, H.; Huang, J.; Chen, D.
Mechanism explanation, data acquisition, and visualization: Cheng, Y.; Xu, M.
Methodology, initial draft writing, and manuscript revision: Jiang, Z.; Yu, H.
Supervision, manuscript revision, and funding acquisition: Huang, Y.; Li, G.; Chen, Y.
Availability of data and materials
The raw data supporting the findings of this study are available within this Article and its Supplementary Materials. Further data is available from the corresponding authors upon reasonable request.
Financial support and sponsorship
This research was financially supported by the National Natural Science Foundation of China (No. 52377222) and the Natural Science Foundation of Hunan Province (No. 2023JJ20064).
Conflicts of interest
All authors declared that there are no conflicts of interest.
Ethical approval and consent to participate
Not applicable.
Consent for publication
Not applicable.
Copyright
© The Author(s) 2025.
Supplementary Materials
REFERENCES
1. Chen, F.; Xu, Z. L. Design and manufacture of high-performance microbatteries: lithium and beyond. Microstructures 2022, 2, 2022012.
2. Hu, Y.; Wang, P.; Li, M.; Liu, Z.; Liang, S.; Fang, G. Challenges and industrial considerations towards stable and high-energy-density aqueous zinc-ion batteries. Energy. Environ. Sci. 2024, 17, 8078-93.
3. Wang, Y.; Wang, Z.; Pang, W. K.; et al. Solvent control of water O-H bonds for highly reversible zinc ion batteries. Nat. Commun. 2023, 14, 2720.
4. Sun, D.; Sun, Z.; Yang, D.; Jiang, X.; Tang, J.; Wang, X. Advances in boron nitride-based materials for electrochemical energy storage and conversion. EcoEnergy 2023, 1, 375-404.
5. Chavhan, M. P.; Kryeziu, A.; Ganguly, S.; Parmentier, J. Monolithic metal-based/porous carbon nanocomposites made from dissolved cellulose for use in electrochemical capacitor. Green. Carbon. 2024, 2, 109-17.
6. Huang, S.; Li, K.; He, Z.; et al. Rolling strategy for highly efficient preparation of phosphating interface enabled the stable lithium anode. J. Alloys. Compd. 2024, 1005, 176193.
7. Yang, H.; Zheng, H.; Yu, H.; et al. Coordinating ionic and electronic conductivity on 3D porous host enabling deep dense lithium deposition toward high-capacity lithium metal anodes. Nanoscale 2022, 14, 13722-30.
8. Cheng, Z.; Wang, K.; Fu, J.; et al. Texture exposure of unconventional (101)Zn facet: enabling dendrite-free Zn Deposition on metallic zinc anodes. Adv. Energy. Mater. 2024, 14, 2304003.
9. Ren, J.; Wu, H.; Yan, W.; Huang, P.; Lai, C. Stable zinc anode by regulating the solvated shell and electrode–electrolyte interface with a sodium tartrate additive. Ind. Chem. Mater. 2024, 2, 328-39.
10. Wei, Z.; Zhang, H.; Li, A.; et al. Construction of in-plane 3D network electrode strategy for promoting zinc ion storage capacity. Energy. Storage. Mater. 2023, 55, 754-62.
11. Bai, Y.; Deng, D.; Wang, J.; et al. Inhibited passivation by bioinspired cell membrane Zn interface for Zn-air batteries with extended temperature adaptability. Adv. Mater. 2024, 36, e2411404.
12. Wang, D.; Li, R.; Dong, J.; et al. Bidentate coordination enables anions-regulated solvation structure for advanced aqueous zinc metal batteries. Angew. Chem. Int. Ed. 2025, 64, e202414117.
13. Zhou, J.; Yu, H.; Qing, P.; et al. Interfacial double-coordination effect reconstructing anode/electrolyte interface for long-term and highly reversible Zn metal anodes. J. Colloid. Interface. Sci. 2025, 678, 772-82.
14. Deng, S.; Xu, B.; Zhao, J.; Fu, H. Advanced design for anti-freezing aqueous zinc-ion batteries. Energy. Storage. Mater. 2024, 70, 103490.
15. Zhou, J.; Zhang, L.; Peng, M.; et al. Diminishing interfacial turbulence by colloid-polymer electrolyte to stabilize zinc ion flux for deep-cycling Zn metal batteries. Adv. Mater. 2022, 34, e2200131.
16. Huang, R.; Zhang, J.; Wang, W.; et al. Dual-anion chemistry synchronously regulating the solvation structure and electric double layer for durable Zn metal anodes. Energy. Environ. Sci. 2024, 17, 3179-90.
17. Liu, W.; Liu, X.; Ning, F.; et al. Fabrication of a heterovalent dual-cation pre-embedded hydrated vanadium oxide cathode for high-performance zinc ion storage. J. Mater. Chem. A. 2024, 12, 11883-94.
18. Ma, G.; Yuan, W.; Li, X.; et al. Organic cations texture zinc metal anodes for deep cycling aqueous zinc batteries. Adv. Mater. 2024, 36, e2408287.
19. Zhang, M.; Li, S.; Tang, R.; et al. Stabilizing Zn/electrolyte interphasial chemistry by a sustained-release drug inspired indium-chelated resin protective layer for high-areal-capacity Zn//V2O5 batteries. Angew. Chem. Int. Ed. 2024, 63, e202405593.
20. Li, J.; Lou, Y.; Zhou, S.; et al. Intrinsically decoupled coordination chemistries enable quasi-eutectic electrolytes with fast kinetics toward enhanced zinc-ion capacitors. Angew. Chem. Int. Ed. 2024, 63, e202406906.
21. Chen, R.; Zhang, W.; Guan, C.; et al. Rational design of an in-situ polymer-inorganic hybrid solid electrolyte interphase for realising stable Zn metal anode under harsh conditions. Angew. Chem. Int. Ed. 2024, 63, e202401987.
22. Shang, Y.; Tong, Z.; Kundu, D. Decoding the zinc depletion-mediated failure in aqueous zinc batteries: on limiting parameters and accurate assessment. ACS. Energy. Lett. 2024, 9, 3084-92.
23. Qu, W.; Wen, C.; Chen, B.; Cai, Y.; Zhang, M. Sulfonate-functionalization in Zn-iodine batteries as one stone kills two birds: iodine limiter and uniform Zn plating guidance layer. Sci. China. Mater. 2024, 67, 2889-97.
24. Yu, H.; He, Z.; Chen, D.; et al. Zwitterionic materials for aqueous Zn-based energy storage devices: current developments and perspective. Energy. Rev. 2025, 4, 100107.
25. Zha, Z.; Sun, T.; Li, D.; Ma, T.; Zhang, W.; Tao, Z. Zwitterion as electrical double layer regulator to in-situ formation of fluorinated interphase towards stable zinc anode. Energy. Storage. Mater. 2024, 64, 103059.
26. Wang, L.; Yu, H.; Chen, D.; et al. Steric hindrance and orientation polarization by a zwitterionic additive to stabilize zinc metal anodes. Carbon. Neutral. 2024, 3, 996-1008.
27. Li, Z.; Shu, Z.; Shen, Z.; et al. Dissolution mechanism for dendrite-free aqueous zinc-ions batteries. Adv. Energy. Mater. 2024, 14, 2400572.
28. Wei, T.; Ren, Y.; Wang, Y.; et al. Addition of dioxane in electrolyte promotes (002)-textured zinc growth and suppressed side reactions in zinc-ion batteries. ACS. Nano. 2023, 17, 3765-75.
29. Li, T. C.; Lin, C.; Luo, M.; et al. Interfacial molecule engineering for reversible Zn electrochemistry. ACS. Energy. Lett. 2023, 8, 3258-68.
30. Liu, D.; Zhang, Y.; Liu, S.; et al. Regulating the electrolyte solvation structure enables ultralong lifespan vanadium-based cathodes with excellent low-temperature performance. Adv. Funct. Mater. 2022, 32, 2111714.
31. Jiang, P.; Du, Q.; Shi, M.; Yang, W.; Liang, X. Stabilizing zinc anodes by a uniform nucleation process with cysteine additive. Small. Methods. 2024, 8, e2300823.
32. Liu, M.; Yuan, W.; Ma, G.; et al. In-Situ integration of a hydrophobic and fast-Zn2+-conductive inorganic interphase to stabilize Zn metal anodes. Angew. Chem. Int. Ed. 2023, 62, e202304444.
33. Zong, W.; Li, J.; Zhang, C.; et al. Dynamical Janus interface design for reversible and fast-charging zinc-iodine battery under extreme operating conditions. J. Am. Chem. Soc. 2024, 146, 21377-88.
34. Zhang, C.; Li, C.; Chen, D.; et al. Zn2+ flux regulator to modulate the interface chemistry toward highly reversible Zn anode. J. Colloid. Interface. Sci. 2025, 682, 232-41.
35. Zhao, R.; Dong, X.; Liang, P.; et al. Prioritizing hetero-metallic interfaces via thermodynamics inertia and kinetics zincophilia metrics for tough Zn-based aqueous batteries. Adv. Mater. 2023, 35, e2209288.
36. Kong, W.; Wan, F.; Lei, Y.; et al. Dynamic detection of decomposition gases in eco-friendly C5F10O gas-insulated power equipment by fiber-enhanced Raman spectroscopy. Anal. Chem. 2024, 96, 15313-21.
37. Zhao, Q.; Liu, W.; Ni, X.; et al. Steering interfacial renovation with highly electronegative Cl modulated trinity effect for exceptional durable zinc anode. Adv. Funct. Mater. 2024, 34, 2404219.
38. Cao, J.; Wu, H.; Zhang, D.; et al. In-situ ultrafast construction of zinc tungstate interface layer for highly reversible zinc anodes. Angew. Chem. Int. Ed. 2024, 63, e202319661.
39. Peng, M.; Tang, X.; Xiao, K.; Hu, T.; Yuan, K.; Chen, Y. Polycation-regulated electrolyte and interfacial electric fields for stable zinc metal batteries. Angew. Chem. Int. Ed. 2023, 62, e202302701.
40. Bu, F.; Gao, Y.; Zhao, W.; et al. Bio-inspired trace hydroxyl-rich electrolyte additives for high-rate and stable Zn-ion batteries at low temperatures. Angew. Chem. Int. Ed. 2024, 63, e202318496.
41. Chang, C.; Hu, S.; Li, T.; et al. A robust gradient solid electrolyte interphase enables fast Zn dissolution and deposition dynamics. Energy. Environ. Sci. 2024, 17, 680-94.
42. Chen, W.; Tan, Y.; Guo, C.; et al. Biomass-derived polymer as a flexible “zincophilic-hydrophobic” solid electrolyte interphase layer to enable practical Zn metal anodes. J. Colloid. Interface. Sci. 2024, 669, 104-16.
43. Ma, X.; Yu, H.; Yan, C.; et al. Nitroxyl radical triggered the construction of a molecular protective layer for achieving durable Zn metal anodes. J. Colloid. Interface. Sci. 2024, 664, 539-48.
44. Yuan, W.; Nie, X.; Wang, Y.; et al. Orientational electrodeposition of highly (002)-textured zinc metal anodes enabled by iodide ions for stable aqueous zinc batteries. ACS. Nano. 2023, 17, 23861-71.
45. Zhu, Q.; Sun, G.; Qiao, S.; et al. Selective shielding of the (002) plane enabling vertically oriented zinc plating for dendrite-free zinc anode. Adv. Mater. 2024, 36, e2308577.
46. Wang, C.; Yang, Y.; Zhang, S.; et al. The impact of surface functional groups on MXene anode protective layer in aqueous zinc-ion batteries: understanding the mechanism. J. Energy. Storage. 2024, 94, 112360.
47. Li, Y.; Yang, X.; He, Y.; et al. A Novel ultrathin multiple-kinetics-enhanced polymer electrolyte editing enabled wide-temperature fast-charging solid-state zinc metal batteries. Adv. Funct. Mater. 2024, 34, 2307736.
48. Yu, H.; Chen, D.; Zhang, L.; et al. Electrolyte engineering for optimizing anode/electrolyte interface towards superior aqueous zinc-ion batteries: a review. Trans. Nonferrous. Met. Soc. China. 2024, 34, 3118-50.
49. Meng, C.; He, W.; Tan, H.; Wu, X.; Liu, H.; Wang, J. A eutectic electrolyte for an ultralong-lived Zn//V2O5 cell: an in situ generated gradient solid-electrolyte interphase. Energy. Environ. Sci. 2023, 16, 3587-99.
50. Li, C.; Liao, T.; Chen, D.; et al. Fabrication of carbon-coated V2O5-x nanoparticles by plasma-enhanced chemical vapor deposition for high-performance aqueous zinc-ion battery composite cathodes. Chin. Chem. Lett. 2025, 36, 110557.
51. Yi, X.; Fu, H.; Rao, A. M.; et al. Safe electrolyte for long-cycling alkali-ion batteries. Nat. Sustain. 2024, 7, 326-37.
52. Qiu, M.; Liang, Y.; Hong, J.; Li, J.; Sun, P.; Mai, W. Entropy-driven hydrated eutectic electrolytes with diverse solvation configurations for all-temperature Zn-ion batteries. Angew. Chem. Int. Ed. 2024, 63, e202407012.
Cite This Article
How to Cite
Download Citation
Export Citation File:
Type of Import
Tips on Downloading Citation
Citation Manager File Format
Type of Import
Direct Import: When the Direct Import option is selected (the default state), a dialogue box will give you the option to Save or Open the downloaded citation data. Choosing Open will either launch your citation manager or give you a choice of applications with which to use the metadata. The Save option saves the file locally for later use.
Indirect Import: When the Indirect Import option is selected, the metadata is displayed and may be copied and pasted as needed.
About This Article
Copyright
Data & Comments
Data
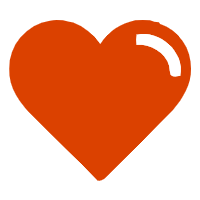
Comments
Comments must be written in English. Spam, offensive content, impersonation, and private information will not be permitted. If any comment is reported and identified as inappropriate content by OAE staff, the comment will be removed without notice. If you have any queries or need any help, please contact us at [email protected].