Charting the course of blood flow: vessel-on-a-chip technologies in thrombosis studies
Abstract
Cardiovascular diseases, primarily driven by thrombosis, remain the leading cause of global mortality. Although traditional cell culture and animal models have provided foundational insights, they often fail to capture the complex pathophysiology of thrombosis, which hinders the development of targeted therapies for cardiovascular diseases. The advent of microfluidics and vascular tissue engineering has propelled the advancement of vessel-on-a-chip technologies, which enable the simulation of the key aspects of Virchow’s Triad: hypercoagulability, alteration in blood flow, and endothelial wall injury. With the ability to replicate patient-specific vascular architectures and hemodynamic conditions, vessel-on-a-chip models offer unprecedented insights into the mechanisms underlying thrombosis formation and progression. This review explores the evolution of microfluidic technologies in thrombosis research, highlighting breakthroughs in endothelialized devices and their roles in emulating conditions such as vessel stenosis, flow reversal, and endothelial damage. The limitations and challenges of the current vessel-on-a-chip systems are addressed, and future perspectives on the potential for personalized medicine and targeted therapies are presented. Vessel-on-a-chip technology holds immense potential for revolutionizing thrombosis research, enabling the development of targeted, patient-specific diagnostic tools and therapeutic strategies. Realizing this potential will require interdisciplinary collaboration and continued innovation in the fields of microfluidics and vascular tissue engineering.
Keywords
INTRODUCTION
Cardiovascular diseases are the leading cause of morbidity worldwide, accounting for 17.9 million deaths annually and representing 32% of all global deaths[1]. Despite their deadly and pervasive nature, they commonly lack deeper awareness of the broader population. The majority of cardiovascular deaths (around 85% of all) are caused by heart attack and stroke, most of which are related to undesired thrombosis caused by excessive platelet aggregation and coagulation[2]. Platelet aggregation is an essential process to prevent blood loss and subsequent vascular repair after vascular injury, and it involves circulating platelets adhering to subendothelial matrix proteins of the vessel wall[3]. However, overactivated platelets at the inflammatory site may trigger an exaggerated aggregation response, leading to cardiovascular diseases.
Traditional in vitro two-dimensional (2D) cell culture and microfluidic chambers, such as parallel-plate flow chambers, have been used to study the molecular mechanisms of thrombosis and anti-thrombotic drug development[4]. For example, flow chamber assays can be applied to study clot formation, growth factors, coagulation factors, cell interplay, and responses during thrombosis[5]. However, these 2D chambers mostly fail to capture complex biochemical and biophysical microenvironments during native thrombosis formation. Alternatively, in vivo animal models have been widely used to advance our understanding of the human circulatory system. However, these models are costly, requiring special provision of care and strict housing, and are under scrutiny owing to ethical considerations[6]. Furthermore, although animal studies have been successful, translational challenges have resulted in many failures in clinical drug trials, highlighting the limitations of currently available diagnostic and blood-thinning treatment options[7]. Thus, the need to establish diagnostic tools and design new treatments for thrombosis has steadily increased over the last several decades[8].
Recent technical improvements in thrombosis studies represent a critical step forward in addressing the global health burden of cardiovascular diseases, offering new opportunities for improving thrombosis diagnosis, anti-thrombotic drug development, and prevention of thrombotic conditions[9]. An emerging technology, in vitro 3D microfluidic models (also known as vessel-on-a-chip) that adhere to the 3R principle (replacement, reduction, and refinement) and can mimic human blood flow and thrombosis formation, is widely used in thrombosis studies[10]. This platform constrains fluids to a micro-scale device and facilitates the analysis of platelet function, coagulation biology, blood rheology, adhesion dynamics, and pharmacology, revolutionizing the study of vascular physiology and its potential for clinical diagnostics[11].
Most microfluidic systems use a variation of photolithography and soft lithography to fabricate microfluidic channels with micrometer resolution, allowing researchers to design the geometry of chips[12-15]. Elastomeric materials, such as polydimethylsiloxane (PDMS), are commonly used for fabrication because of their transparency, biocompatibility, and chemical inertness[16-18]. Simple microfluidic settings can be utilized to investigate shear-dependent adhesion and aggregation of platelets during thrombus formation[19,20]. The success of this wide range of microfluidic systems can also be attributed to manipulable flow geometries that allow fine control over the hemodynamic environment.
In recent years, vessel-on-a-chip models have been widely developed, especially with endothelialized microchannels[21,22], providing the opportunity to study the relationship among the three components of Virchow’s Triad (hemodynamic changes, hypercoagulability, and endothelial injury/dysfunction, as depicted in Figure 1A). More recent attempts to add a biomimetic hydrogel layer underneath the endothelium layer, which mimics the subendothelial matrix layer of native vessels, have gained mechanobiological relevance in these models[23,24]. Owing to these technical advances, there is growing promise in these vessel-on-a-chip models for personalized diagnosis and treatment of cardiovascular diseases. For example, patient-specific thrombotic risk factors or Virchow’s Triad can be recapitulated in microfluidic models[25]. Subsequent thrombosis formation based on the patient-specific thrombosis model can be employed to investigate the efficacy and sensitivity of anti-thrombotic drugs for each patient, greatly improving personalized medicine[26].
Figure 1. Schematic diagram of Virchow’s Triad and structural diagram of human vessels. (A) Three factors dictate vascular thrombosis, also known as the Virchow’s Triad. (B) Structure and composition of the arterial and venous vasculature. ECs: Endothelial cells; SMCs: smooth muscle cells.
This review mainly focuses on developing and applying vessel-on-a-chip microfluidic models in thrombosis research. First, we introduce the anatomical and physiological differences and how the thrombus develops differently among various vessels, especially arteries and veins. Second, we provide an overview of the evolution of microfluidic devices in thrombosis studies, followed by a discussion of the advantages and disadvantages of vessel-on-a-chip technology and their further applications. We emphasize the aspects of shear stress modulation, extracellular matrix (ECM) proteins, and substrate stiffness in endothelial and inflammatory cells.
ANATOMY AND PHYSIOLOGY OF BLOOD VESSELS
Blood vessels are the main components of the human cardiovascular system that circulate blood throughout the body[27]. Based on their structure and function, they are mainly classified as arteries, which carry the blood away from the heart; veins, which bring the bloodstream back to the heart; and capillaries, the smallest vessels connecting arteries and veins, responsible for exchanging metabolic materials between the blood and tissue. Although blood vessels have varying structures, mechanical properties, and specific functions depending on the organs they supply, arteries and veins share the same basic structural layers with varying compositions.
As depicted in Figure 1B, both arteries and veins consist of three concentric layers: the tunica intima, tunica media, and adventitia (tunica externa), each with distinct roles associated with vascular physiology. The innermost layer, the tunica intima, comprises a monolayer of endothelial cells that covers all luminal surfaces[28]. Its predominant role in veins is to serve as a smooth, continuous barrier that minimizes friction and turbulence of blood flow while allowing for the selective perfusion of nutrients and waste from the blood.
The tunica media is composed of circumferentially aligned smooth muscle cells and ECM components, such as type I collagen and elastin fibers, which are typically much thinner in veins than in arteries because of the marked difference in blood pressure in the arterial system (90-100 mmHg) compared to the venous system (5-15 mmHg)[28]. This layer gives vessels characteristic stretchability, allowing them to receive or control blood flow within a dynamic pressure range. Finally, fibroblastic connective tissue constitutes the adventitia, which is the outermost layer of the vessel responsible for structural support.
The characteristic composition of vessels endows them with unique mechanical properties in response to external forces exerted by blood flow, blood pressure, and nearby tissues. Mechanically, three key forces act on blood vessels[28-30]. First, the tensile radial force due to the blood pressure produces an internal circumferential stress (σC). Second, the distending force of blood in the longitudinal direction combined with tethering of the vessel with the surrounding tissue produces an internal longitudinal stress (σL). Most importantly, the flow of blood itself imparts a shear stress on the vessel wall (τW) parallel to the lumen (tangential to the axis flow), given by:
where Q is the volumetric flow rate, µ is the blood viscosity, and ri is the inner radius of the vessel wall. Similarly, wall shear rate (γW) is defined as the rate of change of fluid velocity for perpendicular distance from the vessel wall. Blood pressure creating shear in human blood vessels is crucial to investigate, as it is well understood that altered hemodynamic environments resulting in hemostasis increase the likelihood of platelet aggregation and thrombosis, forming a key pillar of Virchow’s Triad[31]. Veins are particularly susceptible, as they traditionally carry blood under lower shear conditions with an average wall shear rate of 15-200 s-1 compared with arteries at 300-800 s-1[32].
The difference between arteries and veins in different organs lies in the vessel size, wall thickness, and proportion of distinct components in each layer, which gives vessels various mechanical properties. For example, lower wall thickness, particularly in the tunica media, provides veins with different mechanical properties compared to their arterial counterparts owing to decreased elastic tissue and higher amounts of collagen (~0.3×)[33]. Despite the large variation and heterogeneity, few studies have considered vessel anatomy or characterized the mechanical properties of engineered vasculature when studying vascular thrombosis. For example, some popular materials for synthetic vasculature, such as Teflon and Dacron, provide environments that are approximately 50-100 times stiffer (~500-900 MPa) than the in vivo arteries (~10 MPa) and veins (~4-20 MPa). Some common materials used in microfluidics are mismatched or not in the biophysical range[28]. Ideally, biomimetic blood vessels should closely mimic their native counterparts in terms of structure and function while having acceptable mechanical properties.
Given the heterogeneity of blood vessels in terms of anatomy, composition, functions, mechanical variations, and applied force differences, it is essential to consider these comprehensive parameters when designing and attempting to recreate the native environment in an in vitro model.
PATHOPHYSIOLOGY OF THROMBOSIS
Thrombosis is the formation of blood clots within blood vessels, either in arteries or veins, resulting in limited blood flow through the circulatory system, causing serious clinical health issues[2]. Diseases caused by pathological thrombosis include heart attack, stroke, peripheral vascular disease, superficial or deep vein thrombosis (DVT), and pulmonary embolism. Depending on the thrombus location, there are two main types of thrombosis: arterial and venous thrombosis.
Arterial thrombosis involves thrombus formation in an artery and can cause serious cardiovascular diseases depending on the size of the thrombus and the region in which it develops. Cerebral thrombosis may cause stroke, whereas thrombosis in the heart may result in a heart attack. A major cause of arterial thrombosis and embolism is atherosclerosis, in which an unpredictable rupture of the vulnerable fatty plaque initiates subsequent adverse vascular events, including the release of inflammatory cytokines and acute arterial thrombosis, leading to acute coronary syndromes[34]. These vulnerable plaques mainly develop in the branching and bending regions of arteries where altered blood flow is present[35]. The plaques also form arterial stenosis, which further disturbs blood flow and deteriorates the damage induced by hemodynamic changes.
Similarly, blood clot formation in the veins of different regions in the human body is known as venous thrombosis, such as superficial thrombosis in the skin, DVT in the legs, and cerebral venous sinus thrombosis (CVST). Venous thrombosis always forms near the venous valves owing to abrupt and pulsatile hemodynamic conditions[36]. Unlike atherosclerotic arterial thrombosis, which has clear pathophysiological changes in arterial structure and function, the exact causes of venous thrombosis are not fully understood[37-39]. The current understanding of the etiology of thrombosis is an imbalance or disturbance in homeostasis between hemostasis and anti-coagulation via a series of complex pathophysiological mechanisms. Three common risk factors are related to thrombosis and have been described as Virchow’s Triad since the 19th century [Figure 1A]. Virchow’s Triad describes three pathophysiological factors that collaboratively lead to thrombus formation: (1) arterial or venous blood flow stasis; (2) hypercoagulability of the blood; and (3) vessel wall injury[40].
Stasis of arterial or venous blood is usually due to predisposing factors such as pregnancy, immobility, and altered blood hemodynamics caused by pathological conditions, including atherosclerosis, vessel fibrosis, and trauma. Hypercoagulability is a condition in which blood has a highly coagulant predisposition. This is caused by various alterations in coagulation factors, including enhanced levels of pro-coagulant proteins, inflammatory factors, and cytokines, and reduced levels of anti-coagulant factors in the bloodstream. Endothelial damage usually initiates thrombosis, which can be caused by events such as direct disruption of the vessel via catheter placement, trauma, or surgery. These three risk factors (blood stasis, hypercoagulability, and endothelial damage) act together rather than independently. Blood stasis or hemodynamic change is the most common factors in Virchow’s Triad. However, itself alone is insufficient to initiate vessel thrombus formation[41]. The concurrent presence of endothelial damage and hypercoagulability dramatically contributes to a predisposition to thrombus formation[42].
Endothelial health and damage are the key regulators of vessel thrombosis. Endothelial cells play a pivotal role in hemostasis through the expression of a complex of coagulation factors (pro-/anti-coagulants), cell platelet adhesion-related molecules/cytokines, and vessel constriction regulators (vasodilators/vasoconstrictors). Under normal venous conditions, an anti-thrombotic environment is created where platelet adhesion/aggregation and inflammatory activation are hampered[43]. The anti-thrombotic endothelial status is maintained via four key mechanisms[44]: (1) release of heparan/dermatan sulfate that enhances anti-thrombin and heparin cofactor activity; (2) expression of thrombomodulin and activation of protein C, which suppresses thrombosis activation; (3) production of tissue factor (TF) pathway inhibitors (TFPIs), which inhibit the initiation of the coagulation cascade; and (4) endothelial production of fibrinolysis-regulating factors, including tissue (tPA) and urokinase-type plasminogen activator (uPA)[45], which can facilitate the breakdown of coagulated thrombosis. Moreover, the expression of nitric oxide (NO) and interleukin 10 (IL-10) in the endothelium can inhibit leukocyte adhesion and activation[44].
While the majority of thrombosis research focuses on large vessels, such as arteries and veins, thrombosis can also occur in small vessels and capillaries. Microvascular thrombosis is a common complication of various diseases including sepsis, disseminated intravascular coagulation, and thrombotic microangiopathies[46]. The pathophysiology of microvascular thrombosis involves an interplay between endothelial dysfunction, platelet activation, and coagulation cascade activation, leading to the formation of microthrombi that can occlude small vessels and capillaries[47]. The unique hemodynamic conditions and close proximity of endothelial cells to circulating blood constituents in microvessels increase the susceptibility to thrombosis[48]. Studying thrombosis in small vessels and capillaries using microfluidic models can provide valuable insights into the mechanisms underlying microvascular thrombosis and help to develop targeted therapies for these conditions.
EVOLUTION OF MICROFLUIDIC MODELS IN THROMBOSIS RESEARCH
Prior to the invention of microfluidic models, in vitro studies on thrombosis formation were largely limited to the culture of cells on 2D substrates, filters, and flow chambers on a centimeter scale[46]. Most in vitro 2D models have poor biophysiological accuracy. For example, most cells cultured on 2D plastic substrates have a stiffness much greater than that of human tissues or in 3D biosynthetic matrices that exhibit non-biophysiological compositions and porosities. Few displayed constant flow rates over endothelial cells, and even fewer captured the characteristic pulsatile flow stress experienced by the cells in vivo. Microfluidic device technology emerged in the 1980s, which constrains fluids to a small (typically submillimeter scale) scale. It significantly facilitated the analysis of platelet function, coagulation biology, cellular rheology, adhesion dynamics, and pharmacology, revolutionizing the study of vascular physiology and its potential for clinical diagnostics[11].
Techniques for fabricating microfluidic devices have been comprehensively reviewed[10]. Variations in photolithography and soft lithography have been used to fabricate microfluidic channels with micrometer resolution, in which PDMS has been predominantly used because of its transparency and chemical inertness. The success of microfluidics can also be largely attributed to manipulable flow geometries that allow fine control over the hemodynamic environment.
Vessel geometry varies greatly from simple, straight configurations to complex geometries that include stenotic, bifurcated, or branched architectures that better recapitulate flow disturbance-induced thrombosis[47]. Subsequent customizability of physiological and pathophysiological shear environments better emulates mechanical stimuli, including changes to shear stress, shear rate, vorticity, and turbulence at different regions along the microfluidic channel, which are key factors underpinning thrombus formation. In addition, multichannel designs have allowed concurrent investigations of platelet adhesion under various shear rate environments using minimal blood volumes (typically < 100 µL) and reagents[11]. This high-throughput nature and recapitulation of the hemodynamic environment with reduced time and cost factors define the key advantages of microfluidics.
Recently, endothelialized microchannels have been used to model complex interactions between endothelium and blood components[25]. The process of thrombosis initiation and propagation within blood vessels is highly controlled by the blood-endothelium interplay. Introducing perfusable and endothelialized microchannels to microfluidic devices has proven to be an important platform to recapitulate the physiological and pathophysiological responses of the blood-endothelium interface towards flow dynamics that underpin thrombus formation. Table 1 summarizes the most relevant microfluidic models in the field, all of which introduced stenosis geometry and mostly with a sharp angle on their structure. These devices allow us to study real-time platelet clotting by perfusing whole blood on a channel coated with endothelial cells or pro-coagulant adhesion proteins, such as von Willebrand factor (VWF) and collagen. However, most 3D channels are rectangular and do not resemble physiological blood vessels, while diverse stenosis geometries complicate imaging without offering significant experimental benefits beyond the aggregate size. Thus, more detailed studies are needed to determine how platelets react to the vorticity area caused by these geometries, particularly platelet activation.
Existing microfluidic models that facilitate studies of vascular thrombosis under perfusion
Microfluidics chips description | Channel size | Inlet bulk shear rate | Channel functionalization | Perfusion type | Experimental details |
Rectangular cross-section (y-z plane) with a semi-circular stenosis (x-y plane), Figure 2A[48] | 52 µm height, 300 µm width with 20%, 40%, 60%, or 80% semi-circular stenosis | 1,000 s-1, syringe pump-driven flow | Discrete patches of immobilized VWF (20 µg/mL) and fibrinogen (50 µg/mL) | Citrated human WB is pre-labeled with DiOC6 and then recalcified before perfusion | Shear-dependent platelet aggregation with atherosclerosis-like vascular geometries |
Rectangular cross-section (y-z plane) with a trapezoidal stenosis (x-y plane)[49] | 100 µm width with 80% trapezoidal stenosis | 1,800 s-1, syringe pump-driven flow | Non-coated bare channel | Hirudinated WB was pre-labeled with DiOC6 | Characterization of the shear-dependent platelet aggregation with in vitro blood perfusion and CFD simulation |
Rectangular cross-section (y-z plane) with a trapezoidal stenosis (x-y plane)[50] | 100 µL height, 400 µL width with 85% trapezoidal stenosis | 18,800 to 84,700 s-1, syringe pump-driven flow | Non-coated bare channel | Human WB with heparin | Platelet adhesion and aggregation under disturbed blood flow conditions |
Multi rectangular parallel channels with 60° bend section (x-y plane), Figure 2C[53] | 75 µm height, 200 µm width (the bend section corresponds to ~55% stenosis) | 250 to 9,000 s-1, syringe pump-driven flow | Coated with collagen I | Citrated human WB and recalcified before perfusion | Mimic a network of stenosed arteriolar vessels, assessing platelet aggregation and clotting time under pathophysiological flow |
Circular patient-specific venous stenosis channel (y-z plane), Figure 2D[54] | 400 µm diameter with 44% stenosis | 150 s-1, syringe pump-driven flow | Endothelialized: HUVECs seeded on fibronectin (100 µg/mL) | Citrated human WB and recalcified before perfusion | Venous thrombogenesis characterization: fibrin formation and platelet aggregation |
Circular arterial stenosis channel (z axis), Figure 2E[55] | 370 µm diameter with ~68% stenosis | ~1,000 s-1, syringe pump-driven flow | Endothelialized: HUVECs seeded on collagen I (100 µg/mL) | Citrated human WB and recalcified before perfusion | Assess the influence of vessel geometries on platelet aggregation through blood perfusion using vessel designs that simulate both healthy and stenotic conditions |
Rectangular stenosis channel (x-z plane)[57] | Width range: 500 µm to 1,000 µm with 65% and 85% stenosis | ~180 and 460 s-1, syringe pump-driven flow | Coated with VWF (20 µg/mL) and fibrinogen (50 µg/mL) and seeded with HUVECs | Human WB labeled with Rhodamine-6G (with TNFα stimulation for the HUVEC channels) | Shear-dependent leukocyte-endothelium interaction and neutrophil recruitment |
Bifurcating channel with occlusive factor stimulation, Figure 2F[58] | 65 µm height, 500 µm width | 1,000 s-1, vacuum pressure pump-driven flow | Collagen I (100 µg/mL) and tissue factor patch | Citrated human WB and recalcified before perfusion | Monitor occlusion duration by detecting platelet and leukocyte aggregation as well as fibrin formation using eptifibatide, an anti-platelet medication |
Multi-parallel channel with stenotic region (x-y plane)[59] | ranging from 0.6 m length and 0.8 mm width to | 500 to 1,500 s-1, | Non-coated bare channel | Porcine WB with heparin | Measure the occlusion time and volume under various shear rate flow conditions |
Rectangular parallel channel[63] | 50 µm height, 250 µm width for main channel, 50 µm width for side channel | up to ~460 s-1 | Coated with ECs | Citrated human WB and recalcified before perfusion | Assess the concentration effects of FeCl3 on aggregation of platelets and red blood cells |
Straight rectangular channel, Figure 2H[65] | 100 μm height, 400 μm width, 2 cm length | 750 s-1, syringe pump-driven flow | Endothelialized: HUVECs seeded on collagen I (100 µg/mL) | Citrated human WB and recalcified before perfusion | Study thrombus and fibrin formation and platelet adhesion using endothelialized and collagen-coated microfluidic channels in conjunction with anti-platelet drugs |
Based on these advances in microfluidic technologies, new specific applications, such as vessel stenosis and reversed flow, can be employed for more in-depth studies on thrombosis etiology and pathophysiology, as shown in Figure 2. For example, many new models have been developed to replicate the biophysical conditions of narrow vessels. Simple straight channels containing atherosclerotic plaque-like semi-cylindrical barriers provided an 80% stenosis model capable of inducing a shear rate higher than 8,000 s-1, which causes thrombosis in the post-stenosis region [Figure 2A][48]. This further elucidates the importance of endothelial VWF secretion and binding in exacerbating shear-induced platelet activation/aggregation. Other stenotic geometries have been developed, including triangular, square, and trapezoid barriers
Figure 2. Microfluidic models for studying vessel stenosis, reversed flow, and vessel injury. (A) 2D atherosclerotic model developed by Westein et al.[48] Scale bar = 100 µm. (B) Triangular and squared stenotic geometries for computational fluid dynamics (CFD) analysis by Zainal et al.[52] (C) Parallel stenosis channels for hemostasis study by Jain et al.[53] (D) Patient-specific chip for CVST diagnosis by Zhao et al.[54] Scale bar = 400 µm. (E) The arterial thrombosis model by Costa et al.[55] Scale bar = 200 µm. (F) Bifurcated model for the investigation of anti-thrombotic drugs by Berry et al.[58] (G) Characterization of reversed flow vortices by Tovar-Lopez et al.[60]
Most recently, patient-specific geometries have been translated into microfluidic devices from clinical images to enhance rapid diagnostics and drug target identification [Figure 2D and E][54,55]. It is encouraging to witness progress towards personalized thrombosis studies as the vessel-on-a-chip model can accurately reestablish the venous geometry of a CVST patient from the patient’s venography data, where endothelial tissue and whole blood perfusion allows for recapitulating patient-specific Virchow’s Triad for further thrombosis and drug testing.
Microfluidic models have also been used to capture changes in vessel geometry due to bifurcations, sharp curvatures, and localized expansions that can disrupt the unidirectional laminar flow of blood, leading to reversed blood flow patterns known as disturbed flows or vortices. These flows are commonly associated with altered shear stress levels, which may activate inflammatory pathways and disrupt endothelial barrier function, making it prone to thrombosis[56]. Such models have revealed that stable platelet aggregation only occurs in the post-stenotic zone with shear deceleration associated with backflow at distal stenosis[49,51,57]. Berry et al. used an innovative bifurcation model to monitor the effects of anti-thrombotic drugs on occlusion time [Figure 2F][58]; similarly, Li et al. developed a multi-channel system incorporating bifurcation and stenosis to investigate occlusion duration[59]. Despite these advancements, the production of disturbed flows is limited to the expanded region of the device, which, in turn, limits the number of stimulated cells
THE ROLE OF ENDOTHELIALIZED VESSEL-ON-A-CHIP IN THROMBOSIS RESEARCH
Microfluidic technology can simulate aspects of human physiology for thrombosis research that are not achievable using traditional cell culture methods and animal models. However, there is a growing demand for advanced in vitro models that provide more realistic representations of biological processes. Among the various advancements, endothelialized microfluidic devices or vessel-on-a-chip technology can bridge the gap between conventional static models and dynamic complexities of in vivo conditions. In contrast to the existing models, vessel-on-a-chip technology offers a more physiologically relevant platform that meets Virchow’s Triad in that endothelium plays a vital role in regulating hemostasis and preventing thrombosis under normal conditions. By incorporating endothelial cells, these models better mimic the vascular microenvironment and improve the microfluidic platform from the molecular-to-cell level to the cell-to-cell level, allowing for a nuanced understanding of platelet behavior, clot formation, and endothelial responses under flow conditions.
Endothelial cells serve as a barrier to maintain vascular hemostasis and regulate vascular permeability[56]. Vessel-on-a-chip technology provides a way to reveal the connection between vascular walls, coagulation, and the immune system by perfusing blood together with tissue and inflammatory factors. Recently, endothelialized microchannels have been used to model the complex interactions between the endothelium and blood components[25]. The thrombosis initiation and propagation within blood vessels is highly controlled by blood-endothelium interplays. The integration of perfusable, endothelialized microchannels in microfluidic devices is a crucial platform for replicating the responses of the blood-endothelium interface to flow dynamics, which are pivotal in thrombus formation[61]. Table 1 summarizes the most relevant microfluidic models in thrombosis research.
Simulation of vascular injury is crucial for understanding the mechanisms related to thrombus formation and vascular integrity, which can be employed in vessel-on-a-chip models in several distinct ways[62]. Ciciliano et al. used a T-shaped endothelialized microfluidic system that introduced ferric chloride (FeCl3) endothelial damage and platelet activation[63]. In contrast, Herbig and Diamond loaded triangular scaffolds with fibrous matrix materials to mimic the exposed subendothelial matrix around flow stagnation points, which serve as sites of endothelial injury[64]. Models using tumor necrosis factor α (TNFα) or phorbol-12-myristate-13-acetate (PMA) treatment also replicate vessel injury by increasing the expression of intercellular adhesion molecules, such as intercellular adhesion molecule-1 (ICAM-1) and vascular cell adhesion molecule-1 (VCAM-1), and the secretion of VWF and other TFs, resulting in a dose-dependent enhancement of endothelium-platelet binding [Figure 2H][65-67].
Over the past decades, standardized vessel-on-a-chip microfluidic devices with hollow rectangular 3D channels exposed to blood flow have been used to investigate the mechanisms of thrombosis[11,65,68]. These technologies allow researchers to have in situ control of the endothelial environment to study the interaction between the vessel and blood cells under specific conditions in vitro, including the effects of shear stress[69] and vascular injury[70]. Recent studies have shown that 3D printing and dual-layer lithography techniques enable the development of 3D channels with different geometries to mimic vascular stenosis[55,71,72], thus facilitating the investigation of how vessel geometry and flow vorticity in the presence of endothelium would affect thrombosis in pathobiological aspects [Figure 3A]. Costa et al. demonstrated that stenotic vessel-on-a-chip channels could easily trigger thrombosis after 15 min of whole blood perfusion compared with healthy vessel-on-a-chip geometries[55]. Furthermore, Zhang et al. established a microvasculature-on-a-post chip to explore stenosis-induced thrombosis and endothelial behavior and found that the thrombus-like post structure could disrupt endothelial polarization and trigger microvascular thrombosis[73] [Figure 3B]. These studies suggest that there may be a positive correlation between vascular narrowing and thrombus formation, and that stenosis may also affect the integrity and stability of blood vessels, thus triggering thrombosis. However, further research on the mechanisms between endothelium behavior and the process of thrombosis in thrombosis-on-a-chip microfluidics is needed.
Figure 3. Bioengineered endothelialized vessel-on-a-chip for thrombosis research on pathological vascular geometry. (A) Concept of
Vessel-on-a-chip technology aligns with the 3R principle, which reduces the use of animal models, simplifies the vascular architecture, and allows us to design various studies in terms of thrombosis; however, it still exhibits several limitations. First, it lacks the comprehensive anatomical structure of blood vessels, which has a limited representation of microvascular networks. Poventud-Fuentes et al. introduced TFs into a vessel-on-a-chip model to study vascular hemostasis after injury[70] [Figure 3C], which showed a relatively promising wound healing process. However, several injuries failed to achieve full closure in their system for up to 10 min, much longer than the physiological closure process. This suggests that the vessel-on-a-chip system is a good tool for in vitro studies of thrombosis; however, there is a gap between vessel-on-a-chip and physiological conditions regarding the concentration and variety of TFs and coagulation factors. Second, endothelial cells might change their phenotype with an increase in passages in cell culture, and the laboratory cell culture conditions of endothelial cells do not contain variable oxygen tension or a relatively soft extracellular substrate that mimics in vivo conditions[74,75].
CHALLENGES AND OPPORTUNITIES
Despite the advances in microfluidic technologies in thrombosis modeling, there are still limitations in the current microfluidic designs that hamper their wide application to better reconstituted human thrombosis. One fundamental limitation of most microfluidic studies on vascular thrombosis is that most of the geometry of the microfluidic channels is rectangular and cannot replicate the cylindrical geometries of native in vivo vascular vessels. Although this traditional rectangular shape fabricated by 2D silicon wafer-based microfabrication techniques associated with photolithography is easier to fabricate and image by microscopy[65], the flow near the rectangular edges differs from that inside a cylindrical geometry. Therefore, hemodynamic and cellular responses and the analysis of thrombosis near the edge of the channel may not offer useful insights.
Another limitation is that many microfluidic models rely on channels embedded in PDMS, which have mechanical properties far from the native vasculature. Differences in stiffness and strain create variations in flow control and vessel compliance, failing to accurately capture the same biomechanical environments as blood vessels. Moreover, owing to the limited channel size, it is difficult for some scaled microfluidic models to recreate the turbulence of blood flow that mimics the pathophysiological conditions for large and elastic vessels. The short-term duration of current whole-blood perfusion experiments limits studies on long-term thrombosis processes, vascular remodeling, etc.
Notable studies have produced circular cross-sections by combining traditional manufacturing techniques with 3D printing techniques, stereolithography, or gelatin-based soft lithography that have been perfused with blood to study thrombosis[54,55,57]. However, none of them have yielded microfluidic systems in which both their geometries and recapitulation of the physiological environment are truly comparable to native vessels, with the closest being the work of Zhao et al. on patient-specific vessel-on-a-chip systems[54]. All models poorly captured the essential biophysical properties of blood vessels. In addition, endothelium varies among not only individuals but also distinct organs and vascular locations as they are exposed to different shear stresses in different body parts[30]. However, most studies with vessel-on-a-chip models use human umbilical vein endothelial cells (HUVECs), which are not representative of other endothelial cells, such as cells from venous and arterial; thus, organ-specific endothelium should be included to expand the application of vessel-on-a-chip systems. Furthermore, to enhance the replication of blood vessel physiology and deepen our understanding of the interaction among endothelial cells, smooth muscle cells, and thrombosis, it is imperative to co-culture both cell types.
Although 3D bioprinting enables the precise fabrication of intricate tissue constructs and holds promise for developing functional tissues and organs for transplantation, achieving adequate vascularization remains a notable challenge, particularly in printing large or thick tissues[76]. Further development of vessel-on-a-chip technology requires expanding the collaboration of different disciplines, including material science, biomedical engineering, biology, and microscope imaging, to create more comprehensive systems that may enable the study of the interplay between thrombosis and other physiological processes[77]. This will allow us to design personalized chips for patients with thrombosis to improve the diagnostic accuracy and develop more effective treatment strategies. Therefore, establishing a more biomimetic microfluidic model that can more precisely capture the risk factors of thrombosis, biochemical and biophysical environment of vascular vessels, and interplay of these parameters presents a challenging yet critical research frontier that can unlock new diagnostic tools or targets for anti-thrombotic therapeutics.
To this end, a largely separate field of tissue engineering has produced elastomers and hydrogels that more closely mimic the biophysical properties of native vessels and their structural layers. These vascular models are yet to be integrated with microfluidic systems to simulate the flow dynamics and tailored biomechanical loads of a thrombosis model. Furthermore, the application of most existing thrombosis models that have been used to investigate arterial thrombosis compared with the far less researched and understood venous thrombosis. Thus, incorporating vasculature with significantly greater biomimicry into a microfluidic model for the study of venous thrombosis would represent a tremendous step forward and hold potential as a powerful diagnostic tool and model for drug target identification.
Integrating these advanced technologies will pave the way for a new direction of personalized medicine for thrombosis. Personalized microfluidic systems can capture personalized thrombosis profiles, including vessel geometry re-established from patient vessel readouts, blood-endothelium interplays using blood, and engineered endothelium tissues obtained from patients or induced pluripotent stem cells technology, all of which cannot be obtained from animal models and conventional microfluidic systems.
Developing more advanced and biomimetic vessel-on-a-chip models for thrombosis research requires interdisciplinary collaboration among experts from various fields including biomedical engineering, materials science, cell biology, and clinical medicine. Integrating knowledge and techniques from these disciplines will enable the creation of more comprehensive and physiologically relevant models to better recapitulate the complex interplay between thrombosis and other physiological processes. In particular, disruptive soft biosensing technology may offer new opportunities for monitoring thrombosis formation in real-time and in situ if such soft probing technology is integrated into a vessel-on-a-chip system[78,79]. Collaborative efforts will also facilitate the translation of vessel-on-a-chip technology from the laboratory to the clinic, ensuring that the models developed are not only scientifically rigorous but also clinically relevant and applicable.
Integrating endothelialized microfluidics into thrombosis research, particularly through vessel-on-a-chip technology, has catalyzed a significant shift from traditional in vivo and in vitro models. These advanced systems merge the precision of microfabrication with the complexity of endothelial biology, offering nuanced insights into the pathophysiology of thrombosis by mimicking the dynamic interplay between the vascular microenvironment. As a result, vessel-on-a-chip stands at the forefront of personalized medicine, promising for reshaping diagnostics and therapeutic strategies using models that reflect individual patient profiles.
Vessel-on-a-chip technology has significant potential to transform thrombosis research by facilitating the creation of personalized diagnostic tools and treatment strategies. These models mimic native vasculature more accurately than current methods, offering a platform for studying blood perfusion. In the realm of cardiovascular diseases, developing personalized thrombosis models is highly impactful for scientific and medical applications. Past advancements in understanding venous thrombosis mechanisms have driven the discovery of new diagnostic tools and therapies by identifying drug targets. Medically, vessel-on-a-chip technology holds promise for point-of-care applications in the early detection of clotting, stroke, and other thrombosis-related diseases.
This convergence of disciplines is not without its challenges, but the trajectory is clear; vessel-on-a-chip technology paves the way for a future in which the management of thrombotic diseases is as personalized as it is precise, ushering in a new era of targeted and effective healthcare solutions. Realizing this potential will require interdisciplinary collaboration and continued innovation in microfluidics and vascular tissue engineering.
DECLARATIONS
Acknowledgments
We appreciate Yingqi Kaitlyn Zhang for assistance with the table correction and would like to thank Yunduo Charles Zhao and Arian Nasser for their help with the revisions.
Authors’ contributions
Wrote the manuscript and designed the figures: Ren J, Wang Z
Revised the manuscript and reorganized the figures and tables: Ren J
Organized the references: Wang Z
Summarized the microfluidic models used to investigate the mechanisms of vascular thrombosis: Du N
Revised the manuscript: Cheng W
Supervised the whole study and structured and revised the manuscript: Ju LA
Availability of data and materials
Not applicable.
Financial support and sponsorship
This work was supported by the Australian Research Council (ARC) (DP200101970-L.A.J.); the National Health and Medical Research Council (NHMRC) of Australia (APP2003904-L.A.J.); MRFF Cardiovascular Health Mission Grants (MRF2016165-L.A.J. and Z.L.; MRF2023977-L.A.J. T.A., F.P. and Z.L.); NSW Cardiovascular Capacity Building Program (Early-Mid Career Researcher Grant-L.A.J.); Ramaciotti Health Investiment Grants (2020HIG76-L.A.J.); Tour de Cure Pioneering Research Grants (RSP-391-FY2023-L.A.J.); National Heart Foundation Vanguard Grant (106979-L.A.J.); Office of Global and Research Engagement (International Sustainable Development Goal Program-L.A.J.) and Sydney Nano Research Schemes (Grand Challenge-L.A.J. and F.P.). Ju LA is a Snow Fellow (2022SF176) and a National Heart Foundation Future Leader Fellow Level 2 (105863).
Conflicts of interest
All authors declared that there are no conflicts of interest.
Ethical approval and consent to participate
Not applicable.
Consent for publication
Not applicable.
Copyright
© The Author(s) 2024.
REFERENCES
1. World Health Organization. Cardiovascular diseases. Available from: https://www.who.int/news-room/fact-sheets/detail/cardiovascular-diseases-(cvds) [Last accessed on 5 Jun 2023].
2. Ashorobi D, Ameer MA, Fernandez R. Thrombosis. 2019. https://www.ncbi.nlm.nih.gov/books/NBK538430/ [Last accessed on 5 Jun 2023].
3. Kaplan ZS, Jackson SP. The role of platelets in atherothrombosis. Hematology Am Soc Hematol Educ Program 2011;2011:51-61.
4. Zhang Y, Jiang F, Chen Y, Ju LA. Platelet mechanobiology inspired microdevices: from hematological function tests to disease and drug screening. Front Pharmacol 2021;12:779753.
5. Ching T, Toh YC, Hashimoto M, Zhang YS. Bridging the academia-to-industry gap: organ-on-a-chip platforms for safety and toxicology assessment. Trends Pharmacol Sci 2021;42:715-28.
6. Savoji H, Mohammadi MH, Rafatian N, et al. Cardiovascular disease models: a game changing paradigm in drug discovery and screening. Biomaterials 2019;198:3-26.
7. Nguyen N, Thurgood P, Sekar NC, et al. Microfluidic models of the human circulatory system: versatile platforms for exploring mechanobiology and disease modeling. Biophys Rev 2021;13:769-86.
8. Ford ES, Capewell S. Proportion of the decline in cardiovascular mortality disease due to prevention versus treatment: public health versus clinical care. Annu Rev Public Health 2011;32:5-22.
9. Mutch NJ, Walters S, Gardiner EE, et al. Basic science research opportunities in thrombosis and hemostasis: communication from the SSC of the ISTH. J Thromb Haemost 2022;20:1496-506.
10. Sato K, Sato K. Chapter 6 - blood vessels-on-a-chip. In: Principles of human organs-on-chips. Sawston, UK: Woodhead Publishing; 2023, pp. 167-94.
11. Colace TV, Tormoen GW, McCarty OJ, Diamond SL. Microfluidics and coagulation biology. Annu Rev Biomed Eng 2013;15:283-303.
12. Nguyen T, Sarkar T, Tran T, Moinuddin SM, Saha D, Ahsan F. Multilayer soft photolithography fabrication of microfluidic devices using a custom-built wafer-scale PDMS slab aligner and cost-efficient equipment. Micromachines 2022;13:1357.
13. Geisterfer ZM, Oakey J, Gatlin JC. Microfluidic encapsulation of Xenopus laevis cell-free extracts using hydrogel photolithography. STAR Protoc 2020;1:100221.
14. Brower K, White AK, Fordyce PM. Multi-step variable height photolithography for valved multilayer microfluidic devices. J Vis Exp 2017;119:55276.
15. Hattori K, Sugiura S, Kanamori T. Microfluidic perfusion culture. Methods Mol Biol 2014;1104:251-63.
16. Lokai T, Albin B, Qubbaj K, Tiwari AP, Adhikari P, Yang IH. A review on current brain organoid technologies from a biomedical engineering perspective. Exp Neurol 2023;367:114461.
17. Halldorsson S, Lucumi E, Gómez-Sjöberg R, Fleming RMT. Advantages and challenges of microfluidic cell culture in polydimethylsiloxane devices. Biosens Bioelectron 2015;63:218-31.
18. Alhmoud H, Alkhaled M, Kaynak BE, Hanay MS. Leveraging the elastic deformability of polydimethylsiloxane microfluidic channels for efficient intracellular delivery. Lab Chip 2023;23:714-26.
19. Ju L, Chen Y, Zhou F, Lu H, Cruz MA, Zhu C. Von willebrand factor-A1 domain binds platelet glycoprotein Ibα in multiple states with distinctive force-dependent dissociation kinetics. Thromb Res 2015;136:606-12.
20. de Witt SM, Swieringa F, Cavill R, et al. Identification of platelet function defects by multi-parameter assessment of thrombus formation. Nat Commun 2014;5:4257.
21. Ahn J, Yoon MJ, Hong SH, et al. Three-dimensional microengineered vascularised endometrium-on-a-chip. Hum Reprod 2021;36:2720-31.
22. Li X, Mearns SM, Martins-Green M, Liu Y. Procedure for the development of multi-depth circular cross-sectional endothelialized microchannels-on-a-chip. J Vis Exp 2013;80:e50771.
23. Qiu Y, Ahn B, Sakurai Y, et al. Microvasculature-on-a-chip for the long-term study of endothelial barrier dysfunction and microvascular obstruction in disease. Nat Biomed Eng 2018;2:453-63.
24. Ching T, Vasudevan J, Chang SY, et al. Biomimetic vasculatures by 3D-printed porous molds. Small 2022;18:e2203426.
25. Bulboacă AE, Boarescu PM, Melincovici CS, Mihu CM. Microfluidic endothelium-on-a-chip development, from in vivo to in vitro experimental models. Rom J Morphol Embryol 2020;61:15-23.
26. Phan DTT, Wang X, Craver BM, et al. A vascularized and perfused organ-on-a-chip platform for large-scale drug screening applications. Lab Chip 2017;17:511-20.
27. Tucker WD, Arora Y, Mahajan K. Anatomy, blood vessels. Treasure Island, FL: StatPearls Publishing; 2024.
28. Camasão DB, Mantovani D. The mechanical characterization of blood vessels and their substitutes in the continuous quest for physiological-relevant performances. A critical review. Mater Today Bio 2021;10:100106.
31. Pandian NKR, Mannino RG, Lam WA, Jain A. Thrombosis-on-a-chip: prospective impact of microphysiological models of vascular thrombosis. Curr Opin Biomed Eng 2018;5:29-34.
32. Gogia S, Neelamegham S. Role of fluid shear stress in regulating VWF structure, function and related blood disorders. Biorheology 2015;52:319-35.
33. Aird WC. Phenotypic heterogeneity of the endothelium: I. Structure, function, and mechanisms. Circ Res 2007;100:158-73.
34. Zhang S, Liu Y, Cao Y, et al. Targeting the microenvironment of vulnerable atherosclerotic plaques: an emerging diagnosis and therapy strategy for atherosclerosis. Adv Mater 2022;34:e2110660.
35. Chiu JJ, Chien S. Effects of disturbed flow on vascular endothelium: pathophysiological basis and clinical perspectives. Physiol Rev 2011;91:327-87.
36. Bovill EG, van der Vliet A. Venous valvular stasis-associated hypoxia and thrombosis: what is the link? Annu Rev Physiol 2011;73:527-45.
38. Voetsch B, Loscalzo J. Genetic determinants of arterial thrombosis. Arterioscler Thromb Vasc Biol 2004;24:216-29.
39. Bentzon JF, Otsuka F, Virmani R, Falk E. Mechanisms of plaque formation and rupture. Circ Res 2014;114:1852-66.
40. Kumar DR, Hanlin E, Glurich I, Mazza JJ, Yale SH. Virchow’s contribution to the understanding of thrombosis and cellular biology. Clin Med Res 2010;8:168-72.
41. Ashorobi D, Ameer MA, Fernandez R. Thrombosis. Treasure Island, FL: StatPearls Publishing; 2024.
43. Becker BF, Heindl B, Kupatt C, Zahler S. Endothelial function and hemostasis. Z Kardiol 2000;89:160-7.
44. Halcox JP, Deanfield JE. Endothelial cell function testing: how does the method help us in evaluating vascular status? Acta Paediatr Suppl 2004;93:48-54.
45. Wakefield TW, Myers DD, Henke PK. Mechanisms of venous thrombosis and resolution. Arterioscler Thromb Vasc Biol 2008;28:387-91.
46. Wong KH, Chan JM, Kamm RD, Tien J. Microfluidic models of vascular functions. Annu Rev Biomed Eng 2012;14:205-30.
47. Zhang Y, Ramasundara SZ, Preketes-Tardiani RE, Cheng V, Lu H, Ju LA. Emerging microfluidic approaches for platelet mechanobiology and interplay with circulatory systems. Front Cardiovasc Med 2021;8:766513.
48. Westein E, van der Meer AD, Kuijpers MJ, Frimat JP, van den Berg A, Heemskerk JW. Atherosclerotic geometries exacerbate pathological thrombus formation poststenosis in a von Willebrand factor-dependent manner. Proc Natl Acad Sci USA 2013;110:1357-62.
49. Tovar-Lopez FJ, Rosengarten G, Westein E, et al. A microfluidics device to monitor platelet aggregation dynamics in response to strain rate micro-gradients in flowing blood. Lab Chip 2010;10:291-302.
50. Ha H, Lee SJ. Hemodynamic features and platelet aggregation in a stenosed microchannel. Microvasc Res 2013;90:96-105.
51. Jung SY, Yeom E. Microfluidic measurement for blood flow and platelet adhesion around a stenotic channel: effects of tile size on the detection of platelet adhesion in a correlation map. Biomicrofluidics 2017;11:024119.
52. Zainal Abidin NA, Poon EKW, Szydzik C, et al. An extensional strain sensing mechanosome drives adhesion-independent platelet activation at supraphysiological hemodynamic gradients. BMC Biol 2022;20:73.
53. Jain A, Graveline A, Waterhouse A, Vernet A, Flaumenhaft R, Ingber DE. A shear gradient-activated microfluidic device for automated monitoring of whole blood haemostasis and platelet function. Nat Commun 2016;7:10176.
54. Zhao YC, Zhang Y, Wang Z, et al. Novel movable typing for personalized vein-chips in large scale: recapitulate patient-specific virchow’s triad and its contribution to cerebral venous sinus thrombosis. Adv Funct Mater 2023;33:2214179.
55. Costa PF, Albers HJ, Linssen JEA, et al. Mimicking arterial thrombosis in a 3D-printed microfluidic in vitro vascular model based on computed tomography angiography data. Lab Chip 2017;17:2785-92.
56. Gimbrone MA Jr, Topper JN, Nagel T, Anderson KR, Garcia-Cardeña G. Endothelial dysfunction, hemodynamic forces, and atherogenesis. Ann N Y Acad Sci 2000;902:230-9; discussion 239-40.
57. Menon NV, Su C, Pang KT, et al. Recapitulating atherogenic flow disturbances and vascular inflammation in a perfusable 3D stenosis model. Biofabrication 2020;12:045009.
58. Berry J, Peaudecerf FJ, Masters NA, Neeves KB, Goldstein RE, Harper MT. An “occlusive thrombosis-on-a-chip” microfluidic device for investigating the effect of anti-thrombotic drugs. Lab Chip 2021;21:4104-17.
59. Li M, Ku DN, Forest CR. Microfluidic system for simultaneous optical measurement of platelet aggregation at multiple shear rates in whole blood. Lab Chip 2012;12:1355-62.
60. Tovar-Lopez F, Thurgood P, Gilliam C, et al. A microfluidic system for studying the effects of disturbed flow on endothelial cells. Front Bioeng Biotechnol 2019;7:81.
61. Hesh CA, Qiu Y, Lam WA. Vascularized microfluidics and the blood-endothelium interface. Micromachines 2019;11:18.
62. Sakurai Y, Hardy ET, Ahn B, et al. A microengineered vascularized bleeding model that integrates the principal components of hemostasis. Nat Commun 2018;9:509.
63. Ciciliano JC, Sakurai Y, Myers DR, et al. Resolving the multifaceted mechanisms of the ferric chloride thrombosis model using an interdisciplinary microfluidic approach. Blood 2015;126:817-24.
64. Herbig BA, Diamond SL. Thrombi produced in stagnation point flows have a core-shell structure. Cell Mol Bioeng 2017;10:515-21.
65. Jain A, van der Meer AD, Papa AL, et al. Assessment of whole blood thrombosis in a microfluidic device lined by fixed human endothelium. Biomed Microdevices 2016;18:73.
66. Dupuy A, Hagimola L, Mgaieth NSA, et al. Thromboinflammation model-on-a-chip by whole blood microfluidics on fixed human endothelium. Diagnostics 2021;11:203.
67. Zhang X, Bishawi M, Zhang G, et al. Modeling early stage atherosclerosis in a primary human vascular microphysiological system. Nat Commun 2020;11:5426.
68. Zheng Y, Chen J, Craven M, et al. In vitro microvessels for the study of angiogenesis and thrombosis. Proc Natl Acad Sci USA 2012;109:9342-7.
69. Milusev A, Ren J, Despont A, et al. Glycocalyx dynamics and the inflammatory response of genetically modified porcine endothelial cells. Xenotransplantation 2023;30:e12820.
70. Poventud-Fuentes I, Kwon KW, Seo J, et al. A human vascular injury-on-a-chip model of hemostasis. Small 2021;17:e2004889.
71. Yaneva-Sirakova T, Serbezova I, Vassilev D. Functional assessment of intermediate vascular disease. Biomed Res Int 2018;2018:7619092.
72. Li Y, Wang J, Wan W, et al. Engineering a Bi-Conical microchip as vascular stenosis model. Micromachines 2019;10:790.
73. Zhang Y, Aye S, Cheng V, et al. Microvasculature-on-a-post chip that recapitulates prothrombotic vascular geometries and 3D flow disturbance. Adv Mater Inter 2023;10:2300234.
74. Kolodziejczyk AM, Kucinska M, Jakubowska A, et al. Endothelial cell aging detection by means of atomic force spectroscopy. J Mol Recognit 2020;33:e2853.
75. Lai A, Zhou Y, Thurgood P, et al. Endothelial response to the combined biomechanics of vessel stiffness and shear stress is regulated via piezo1. ACS Appl Mater Interfaces 2023;15:59103-16.
76. Matai I, Kaur G, Seyedsalehi A, McClinton A, Laurencin CT. Progress in 3D bioprinting technology for tissue/organ regenerative engineering. Biomaterials 2020;226:119536.
77. Chen L, Yang C, Xiao Y, et al. Millifluidics, microfluidics, and nanofluidics: manipulating fluids at varying length scales. Mater Today Nano 2021;16:100136.
78. Lyu Q, Gong S, Yin J, Dyson JM, Cheng W. Soft wearable healthcare materials and devices. Adv Healthc Mater 2021;10:e2100577.
Cite This Article
Export citation file: BibTeX | EndNote | RIS
OAE Style
Ren J, Wang Z, Du N, Cheng W, Ju LA. Charting the course of blood flow: vessel-on-a-chip technologies in thrombosis studies. Microstructures 2024;4:2024037. http://dx.doi.org/10.20517/microstructures.2023.106
AMA Style
Ren J, Wang Z, Du N, Cheng W, Ju LA. Charting the course of blood flow: vessel-on-a-chip technologies in thrombosis studies. Microstructures. 2024; 4(3): 2024037. http://dx.doi.org/10.20517/microstructures.2023.106
Chicago/Turabian Style
Jianfang Ren, Zhao Wang, Nixon Du, Wenlong Cheng, Lining Arnold Ju. 2024. "Charting the course of blood flow: vessel-on-a-chip technologies in thrombosis studies" Microstructures. 4, no.3: 2024037. http://dx.doi.org/10.20517/microstructures.2023.106
ACS Style
Ren, J.; Wang Z.; Du N.; Cheng W.; Ju LA. Charting the course of blood flow: vessel-on-a-chip technologies in thrombosis studies. Microstructures. 2024, 4, 2024037. http://dx.doi.org/10.20517/microstructures.2023.106
About This Article
Special Issue
Copyright
Data & Comments
Data
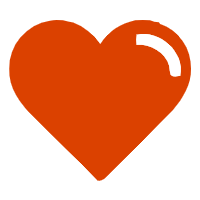
Comments
Comments must be written in English. Spam, offensive content, impersonation, and private information will not be permitted. If any comment is reported and identified as inappropriate content by OAE staff, the comment will be removed without notice. If you have any queries or need any help, please contact us at support@oaepublish.com.