Reducing greenhouse gas emissions in China’s automobile manufacturing with circular economy strategies
Abstract
Circular economy strategies encompass a wide range of approaches and initiatives designed to foster sustainable resource utilization and minimize waste, particularly in the context of complex products such as automobiles. These strategies encompass, among others, material recycling and reuse, energy recovery, efficiency enhancements, and circular-focused product design. To evaluate the potential of different circular economy strategies to reduce greenhouse gas emissions in China’s automobile manufacturing, this research employs the input-output subsystems approach to analyze three distinct scenarios based on the interconnections between various components: the low-carbon transition of the energy structure, closed-loop material recycling, and the transition to shared mobility. The findings reveal that spillover component emissions account for over 98% of the total emissions of the automobile manufacturing sector. The circular economy model, through material recycling, can significantly cut down emissions from these spillover components, thereby aiding the automobile manufacturing industry in meeting its emission reduction targets. Notably, compared to relying solely on the low-carbon transition of the energy structure, the closed-loop material recycling scenario can reduce greenhouse gas emissions by approximately 10% through the recycling of steel and plastics alone. Moreover, the transition to shared mobility has the potential to achieve an additional 4%-18% reduction in greenhouse gas emissions by diminishing the final demand for automotive products.
Keywords
INTRODUCTION
The low-carbon transition of the automotive industry is a critical component of the global effort to achieve carbon neutrality[1]. The transition involves not only the development of electric and hybrid vehicles but also the optimization of the entire production chain, from raw material sourcing to end-of-life vehicle disposal, which brings significant challenges to the automotive industry[2]. As a pillar of the national economy, the automotive industry should balance its efforts to reduce greenhouse gas (GHG) emissions with the need to address a multitude of socio-economic factors. The GHG emission reduction extends beyond mere energy transitions, encompassing the intricate industrial linkages involved in upstream material production[3]. Furthermore, emerging intelligent driving technologies have the potential to fundamentally transform people’s travel patterns[4].
A comprehensive circular economy strategy, which has significant potential for GHG emission reduction, can incorporate the broader context of the economic system with intricated cross-sectoral linkages of the modern industrial system, which requires a systemic view of the socio-economic system[5]. Considering the holistic approach of the circular economy strategy, which spans from reduction initiatives and material recycling to energy recovery, and requires collaborative efforts from all stakeholders across the product’s entire lifecycle, the following pivotal research questions (RQ) emerge as the automobile industry stands at this crucial juncture of technological transition:
RQ1: What are the key circular economy strategies with the potential to significantly decrease greenhouse gas emissions in China’s automobile manufacturing sector?
RQ2: Additionally, to what extent are these strategies effective in realizing the overarching objective of decarbonization?
The aim of this research is to quantitatively assess the potential of different circular economy strategies for GHG emission reduction in the low-carbon transition of the automobile industry, using an input-output subsystem analysis tool to link sector-level efforts to their broad effects on the national economy. The next section will position our research into the existing literature on the decarbonization of the automotive industry. Section "METHODOLOGY AND DATA" presents the methods and data used in the analysis. Section "RESULTS AND DISCUSSIONS" shows the research results, followed by a general discussion. Finally, the implications to various stakeholders for the decarbonization of the automobile industry along the circular value chains conclude the paper.
LITERATURE REVIEW
The global automotive industry is undergoing a pivotal transition toward low-carbon technologies, with the electrification of road transport anticipated to significantly reduce emissions in the forthcoming decades[6]. In 2023, the progressive electrification of Chinese passenger cars alone accounted for about 35% of the global road transport emissions avoided, showing the great potential to unlock cumulative carbon benefits[1]. GHG emissions from material production constitute a significant proportion of the total carbon footprint across the entire lifecycle of an automobile, and this proportion is expected to rise further as energy sources shift toward lower-carbon alternatives[7]. Therefore, the circular economy strategy will play an increasingly important role in the low-carbon development of the automobile industry.
Multiple innovative pathways toward low-carbon transportation
The circular economy, adhering to the 3R principles (Reduce, Reuse, and Recycle), leads to diversified technological pathways for GHG reduction across the industrial chain, which brings great opportunities for technological innovation and new business model development[8].
The current low-carbon transition of the automobile industry focuses on electrification and energy substitution. This shift is a pivotal response to the global challenge of reducing greenhouse gas emissions and mitigating climate change[6]. Electrification involves the transition from traditional internal combustion engines (ICEs) to electric vehicles (EVs), which include battery electric vehicles (BEVs), plug-in hybrid electric vehicles (PHEVs), and fuel cell electric vehicles (FCEVs), whose lifecycle GHG emissions highly rely on the regional energy system[9]. Energy substitution, on the other hand, refers to the replacement of fossil fuels with renewable and sustainable energy sources for the entire transportation and manufacturing sector[10]. This includes the use of biofuels, synthetic fuels, and hydrogen produced from renewable energy, as well as renewable sources of electricity for EVs. By prioritizing electrification and energy substitution, the automotive industry aims to achieve a significant reduction in GHG emissions, improve air quality, and contribute to a more sustainable transportation system[11].
However, the transformation of the energy system alone is not enough[7]. The potential to significantly reduce the lifecycle emissions from the automotive industry through the construction of a closed-loop supply chain has been widely recognized, from lifecycle cost and GHG emission of a vehicle[12], to the recycling of key raw materials such as scrap steel[13], scrap aluminum[14], and scrap plastics[15], as well as battery recycling[16,17]. According to China Automotive Data Co., if the automotive industry could achieve carbon neutrality by 2050, the proportion of GHG emissions during vehicle manufacturing to total lifecycle GHG emissions will rise to 66% by 2060[18], almost double the current status. The potential of a circular economy for GHG emission reduction is of increasing significance in automobile producers’ low-carbon strategy[7], besides securing supply for various critical metals for the surging markets[16].
Furthermore, the development of low-carbon transportation necessitates not only the transition to cleaner vehicle technologies but also a holistic approach that reduces the overall demand for vehicle products through technological innovation, business model transformation, and urban planning reforms[19]. Technological innovation plays a crucial role in enhancing the efficiency and sustainability of transportation systems[20]. Advancements in intelligent transportation systems (ITS), for instance, enable more efficient traffic management, reducing congestion and minimizing the need for additional vehicles[21,22]. Autonomous vehicles and shared mobility services can further optimize vehicle usage, as they allow for more flexible and efficient transportation options that cater to varying passenger demands[23].
In general, the shift toward a circular economy in the transportation sector could be achieved through various pathways, including promoting practices such as vehicle sharing, leasing, and the reuse and recycling of vehicle components[17]. By extending the lifespan of vehicles and maximizing their utilization, the demand for new vehicle production can be curtailed, thereby lowering GHG emissions associated with manufacturing processes[24]. On the other hand, urban planning is equally important in shaping low-carbon transportation systems[25]. Compact, mixed-use urban development could encourage walking, cycling, and the use of public transportation, reducing the reliance on private vehicles[26]. The integration of green infrastructure, such as bike lanes, pedestrian pathways, and public transit networks, further supports sustainable mobility options[27,28]. Additionally, the implementation of congestion pricing, low-emission zones, and incentives for low-carbon transportation choices can effectively reduce vehicle demand and promote environmental sustainability[29,30]. Collectively, these strategies aim to create a transportation system that is more efficient, sustainable, and resilient.
Extensive socio-economic impacts of low-carbon transition through circular economy
The socio-economic ramifications of the technological shifts and business model innovations in the circular economy remain uncharted territory due to the complexity of the economic system[31]. The economic impact of circular economy practices on traditional industrial structures is crucial in addressing the broad impact of technological change in a single industrial sector toward net-zero emission, which could lead to complex feedback effects in economies heavily reliant on linear economic models[32].
Furthermore, the policy and regulatory framework plays a pivotal role in shaping the socio-economic outcomes of technological shifts and business model innovations in emerging technologies that could bring radical change to human society, such as autonomous technology[33]. Governments and regulatory bodies are developing policies to incentivize circular practices, address potential market failures, and ensure a fair playing field for all stakeholders[34]. These measures include extended producer responsibility (EPR) policies[35], subsidies for green innovation[36,37], and tax incentives for sustainable practices[38].
In summary, while the technological advancements and business model innovations inherent in the circular economy present promising pathways for achieving a low-carbon transition in the automotive sector, their socio-economic implications are intricate and multifaceted. To fully leverage the potential of the circular economy, a comprehensive and inclusive approach is imperative, one that considers economic, social, policy, and global dimensions[39]. This approach should prioritize actions that promote both closed-loop recycling within the automobile supply chains[40], and inter-sectoral flows of recyclable materials for general durable products, as these elements are crucial for reducing the lifecycle GHG emission in both the automotive industry and the broader manufacturing landscape[34].
Quantifying the GHG emission reduction potential of circular economy strategies
Existing research on the circular economy within the automotive sector acknowledges the considerable potential of circular practices in mitigating GHG emissions throughout the lifecycle of automotive products. However, it also recognizes the substantial uncertainties surrounding the actualization of this potential, largely driven by the dramatic technological change in this sector[41]. These uncertainties are intricately woven with a myriad of complex factors, encompassing governmental macro-policies, corporate competitive strategies, and individual travel behaviors[42]. As such, quantifying these discrepancies carries immense significance.
Therefore, this paper conducts a quantitative assessment of the potential of various circular economy strategies in reducing GHG emissions during the low-carbon transition of the automobile industry. This assessment links sector-specific efforts to national low-carbon policies. The input-output subsystem method is employed to dissect the underlying driving mechanisms behind GHG emissions in automobile manufacturing within economic systems. This method views a single industrial sector or a cluster of sectors as a subsystem within the broader economy. It has previously been utilized to investigate the overarching carbon emission driving mechanisms in China[43]. By examining the input-output connections between this subsystem and other industry sectors in the economy, it differentiates the resources consumed and pollutants emitted by the subsystem according to distinct driving mechanisms, considering final demands such as consumption, investment, and exports[44].
Compared to traditional research methods, this approach presents two distinct advantages for emissions analysis:
First, by isolating a single industry sector or a group of sectors as a subsystem within the broader economy, it examines the intricate interactions of emissions among various branch sectors within this subsystem, as well as the interrelations across diverse subsystems of the entire economic framework. Additionally, it accounts for external influences by incorporating the import/export sector, thereby enabling a systematic identification of key sectors that significantly impact emissions within the overall economic system[45].
Second, by leveraging the input-output linkages between different industry sectors, this method unveils the direct and indirect emissions generated by the subsystem to fulfill its own final demand. By categorizing various driving mechanisms, it highlights the industrial linkage effects of emissions within the subsystem. This detailed understanding empowers policymakers to devise more tailored and effective policies and measures for emission reduction[44]. Furthermore, based on the insights derived from the input-output subsystem analysis, the potential for GHG emission reduction will be assessed under various scenarios, which can offer implementation to actions for various stakeholders.
METHODOLOGY AND DATA
Subsystem input-output decomposition analysis
This study adopts the input-output subsystem approach proposed by Alcántara et al.[44] and Liu et al.[43]. This method decomposes the total emission of a certain pollutant generated by the j-th industry sector in the economy, driven by final demand, into three stages, ultimately breaking it down into 12 components.
The expression for the first decomposition is as follows:
where
• Ei and Ej represent the emission intensities of the i-th and j-th industry sectors, respectively.
• ljj and lij denote the corresponding elements in the Leontief inverse matrix.
• yj represents the final demand value of the j-th industry sector.
• Ej(ljj -1)yj signifies the emission generated by the j-th industry sector in producing intermediate inputs to meet its own final demand, termed the internal component.
•
• Ejyj represents the emission directly associated with the scale of final demand in the j-th industry sector, known as the scale component.
The expression for the second decomposition is as follows:
where
•
•
•
• Ejyj represents the scale component, which is directly related to the scale of final demand in the j-th industry sector.
The expression for the third decomposition is as follows:
where
• y1j, y2j, and y3j correspond to the consumption expenditure, capital formation, and export values of the
The input-output subsystem approach defines the emissions generated by a subsystem to meet final demand as “total emissions”[44], which consist of two parts: “direct emissions” and “indirect emissions”. Direct emissions refer to those emissions that are directly influenced by the scale of the subsystem’s final demand and are directly related to the scale of the subsystem’s final demand, independent of the production process of intermediate inputs. These correspond to the scale of production within the subsystem. Indirect emissions, on the other hand, refer to the emissions generated indirectly by providing productive inputs to satisfy the subsystem’s final demand. They arise from the production of intermediate inputs required by the subsystem to meet its final demand and correspond to feedback effects and spillover along the supply chains.
Data source
The data used in this paper are from the CEEIO database (China’s Environmentally Extended Input-Output Database), jointly developed by the Industrial Ecology teams at the University of Michigan, Guangdong University of Technology, and Nanchang University. This database is a comprehensive, transparent, and consistent environmentally extended input-output database designed for studying environmental issues in China[46,47]. The research focuses on greenhouse gas emissions, including carbon dioxide, methane, and nitrous oxide.
For industrial categories, the study selects two specific subcategories within the automobile manufacturing industry from the 153-sector table in the 2018 CEEIO data: Automobile Vehicle Manufacturing and Auto Parts and Accessories Manufacturing. The remaining industrial categories are merged according to the classifications in the 96-sector data table, resulting in a new 97-sector industry data table.
The research follows the “proportional import” assumption. Based on the proportion of imported products in each sector’s total domestic use, imported products are separated from the intermediate input matrix and the final use matrix of the competitive input-output table. This transformation converts the original competitive input-output table into a non-competitive one, avoiding exaggerated calculation results when measuring domestic resource consumption and emissions[48].
Scenario analysis
Based on the decomposition results of the input-output subsystems, this paper sets a baseline scenario and three emission reduction scenarios, i.e., the low-carbon transition of energy structure, closed-loop recycling of materials, and sharing mobility transition. The specific parameter settings for each scenario are shown in Table 1. The paper then simulates and estimates the GHG emission reduction potential under different scenarios.
Scenario setting
Scenarios | Change in energy structure | Change in direct input coefficient | Change in final demand |
Baseline (2030) | Renewable energy accounts for 25% | Unchanged | 1. The final consumption growing with an annual growth rate of 2% 2. The capital formation growing with an annual growth rate of 5.89% 3. The export growing with an annual growth rate of 5.60% |
S1: Low-carbon transition of energy structure | Renewable energy rises to 70% | Unchanged | Id |
S2: Closed-loop recycling of materials | Id | Given the increasing rate of recycling materials used in automobile manufacturing, the intermediate input value for the “ferrous metal smelting and rolling processing industry” and the “plastic product industry” consumed by the automobile manufacturing industry is reduced, and a new intermediate flow matrix and direct consumption coefficient matrix are generated | Id |
S3: Sharing mobility transition | Id | Id | All three final demands reduced by 13% to 57% on the basis of the annual growth rate |
Baseline scenario
The Chinese government announced “The goals of carbon peaking and carbon neutrality” to achieve carbon peaking by 2030 and carbon neutrality by 2060 at the 75th United Nations General Assembly. Based on this, the benchmark scenario in this study is set for the year 2030, with specific parameter settings as follows:
1. Changes in energy structure: According to the “China Energy Statistical Yearbook (2021)”[49], in 2018, fossil fuels accounted for 85.5% of China’s total energy consumption, while non-fossil fuels accounted for 14.5%. On October 24, 2021, the State Council issued the “Action Plan for Carbon Peaking Before 2030”, notifying that “by 2030, the share of non-fossil fuels in China’s energy consumption will reach around 25%”[50]. Based on this, this paper sets the share of non-fossil fuels in primary energy consumption in the benchmark scenario at 25% and adjusts the emission intensity across various industrial sectors.
The direct input coefficients remain unchanged from the 2018 non-competitive input-output table for domestic products.
2. Changes in final demand: The benchmark scenario sets parameters for changes in final demand from three perspectives: changes in final consumption, changes in capital formation, and changes in exports. Specifically: (1) Changes in final consumption: the China Association of Automobile Manufacturers predicts that China’s total vehicle sales will maintain an annual growth rate of 1.54% over the next five years (2020-2025), 2% over the next ten years (2020-2030), and 2.18% over the next fifteen years (2020-2035) in their “China Automobile Market Medium and Long-Term Forecast Report (2020-2035)”[51]. Based on this, this paper sets the final consumption parameter in the benchmark scenario to grow steadily at a CAGR of 2% from 2018 onwards; and (2) Changes in capital formation and exports: Using the conversion coefficient at constant prices from 2012, this paper set the annual growth rate of capital formation (5.89%) and exports (5.60%) in the automobile manufacturing industry from 2012 to 2030.
Scenario 1: low-carbon energy transition
The low-carbon transition of the energy structure scenario serves as a reference standard for the three types of emission reduction scenarios, reflecting the overall impact of energy structure optimization and transformation on GHG emission reduction. Specifically, it reflects the impact of a decrease in the proportion of fossil fuels in primary energy consumption and an increase in the proportion of non-fossil fuels.
The “Frontier Report on China’s Energy Development (2021)” predicts that under the “ The goals of carbon peaking and carbon neutrality scenario, the share of fossil fuel consumption in China will decrease to around 31% by 2060[52]. Based on this, this paper sets the share of non-fossil fuels in primary energy consumption in the low-carbon energy transition scenario at 70% and adjusts the emission intensity across various industrial sectors. Other parameters remain consistent with the baseline scenario.
Scenario 2: closed-loop recycling of materials scenario
The material closed-loop reuse scenario reflects the impact of closed-loop recycling of key automotive raw materials, where discarded materials are used to produce new products of the same type, on GHG emission reduction.
Currently, the construction of a closed-loop supply chain in China’s automobile manufacturing industry is in its initial stages, with production primarily using virgin materials. This paper takes steel and plastic as examples. Due to the complexity of non-ferrous metals as a major material consumed in automobile manufacturing and the uncertainty in their usage affected by the transition to new energy vehicles, they are not discussed here. For steel, currently, only 8% of recycled steel meets the quality standards for reuse in automobiles, but with appropriate dismantling processes and intelligent scrap steel recovery and processing flows, it is estimated that 85% of the steel demand in automobile production could be met through recycled steel[53]. For plastics, existing research on dynamic material flow analysis of passenger vehicle plastics in China shows that from 1950 to 2018, only 28% of automotive scrap plastics were recycled, with only half of these (14%) being reused within the industry through component reuse[54]. With improvement in dismantling and recycling of automobiles and the development of remanufacturing, the recovery rate of automotive plastics is expected to reach up to 80%, and based on the estimation that the closed-loop reuse rate is roughly half of the recovery rate, the closed-loop reuse rate of automotive plastics is expected to reach up to 40%.
In summary, this paper first assumes a recycling rate of 8% for the “Iron and Steel Smelting and Rolling Processing Industry (corresponding to steel)” and 14% for the “Plastic Product Manufacturing Industry (corresponding to plastic)” in the baseline scenario. In the closed-loop recycling of materials scenario, the recycling rates are set at 85% for the “Iron and Steel Smelting and Rolling Processing Industry” and 40% for the “Plastic Product Manufacturing Industry”. Subsequently, based on this, the input value of the “Iron and Steel Smelting and Rolling Processing Industry” and “Plastic Product Manufacturing Industry” consumed by the automobile manufacturing sector in the intermediate flow matrix of the baseline scenario is reduced. Finally, using the improved R.A.S. method[55], a new intermediate flow matrix is generated and the direct input coefficient matrix is adjusted. Other parameters remain consistent with the low-carbon energy transition scenario to analyze the further GHG emission reduction potential through materials recycling.
Scenario 3: sharing mobility transition scenario
The scenario of the sharing mobility transition reflects the impact of implementing the sharing mobility business model on GHG emission reduction, through reduction in in-stock vehicles with smart transportation.
Sharing mobility refers to the transportation model where people share vehicles with others through shared rides or carpooling without needing to own the vehicle themselves, paying a corresponding usage fee based on their travel needs. This includes a wide range of innovative models represented by ride-hailing and car-sharing. Sharing mobility relies on providing better mobility services on demand rather than continuously selling new cars. It primarily achieves emission reductions by substituting private car use, increasing vehicle utilization rates, and enhancing urban transportation efficiency[56].
A research report published in 2020 by the International Resource Panel (IRP) of the United Nations Environment Programme (UNEP) points out that by 2050, sharing models, including car-sharing and ride-sharing, have the potential to reduce car ownership in the G7 countries by 13% to 57% without altering consumers’ travel modes[57].
Based on this, in the sharing mobility transition scenario, the final demand is reduced by 13% for the lowest potential, and 57% for the highest potential, to illustrate the impact of this factor on overall GHG emission reduction in scenario 3. Other parameters remain consistent with the scenario of closed-loop material recycling.
RESULTS AND DISCUSSIONS
In this section, we will first present the research findings of the input-output subsystem analysis. Building upon these results, we will delve into the primary mechanisms across sectors in the socio-economic system that influence the GHG emissions of automobile manufacturing. Lastly, through a comparison of various simulation scenarios, we will elucidate the effects of various strategies of circular economy transition within the socio-economic system on GHG emissions in the automotive manufacturing sector.
The components of GHG emissions of automobile manufacturing
Table 2 presents the comprehensive decomposition of GHG emissions within the automobile manufacturing industry. Remarkably, emissions stemming from the spillover component constitute over 98% of the total GHG emissions, indicating an unequivocally dominant presence. This finding not only corroborates previous product-level Life Cycle Assessment (LCA) reports[18], but also highlights an even more pronounced share. This discrepancy can be attributed to the methodology used in environmental extended input-output analysis, which allocates emissions based on product value. Consequently, the high monetary value of automobile products can artificially inflate their emission contributions relative to their actual quantities.
The input-output subsystem decomposition of carbon emission in China’s automobile manufacturing sector
Final demands | Capital formation | Export | In total | |||||
tonne | % | tonne | % | tonne | % | tonne | % | |
Own internal | 105,647.7 | 0.02 | 268,000.5 | 0.06 | 112,908.5 | 0.03 | 486,556.6 | 0.11 |
Feedback | 1,929.9 | 0.00 | 4,470.7 | 0.00 | 3,629.1 | 0.00 | 10,029.7 | 0.00 |
Spillover | 104,265,073.3 | 23.98 | 283,367,244.8 | 65.18 | 41,859,411.0 | 9.63 | 429,491,729.1 | 98.79 |
Scale | 1,170,994.3 | 0.27 | 3,200,198.7 | 0.74 | 404,805.1 | 0.09 | 4,775,998.1 | 1.10 |
On the other hand, emissions from the scale component account for a smaller yet significant proportion, contributing 1.1% to overall GHG emissions. Conversely, emissions from both the internal and feedback components are extremely low, to the point of being virtually negligible. These observations imply that the majority of GHG emissions generated by China’s automobile manufacturing sector to satisfy its own final demand originate predominantly from external industrial sectors outside the immediate system, notably those involved in energy supply and materials production. As such, the automobile manufacturing industry plays a pivotal role in driving GHG emissions from these interconnected sectors.
The detailed decomposition of carbon emissions in China’s automobile manufacturing sector shows that, within the spillover effect, which represents emissions indirectly caused by upstream activities linked to the sector, capital formation emerges as the most important driver of GHG emissions. Specifically, capital formation accounts for 65.18% of total spillover emissions, totaling 283 billion tonnes. This significantly outpaces both exports and final consumption in terms of their contribution to emissions growth, highlighting that upstream industries are far more strongly driven by investment activities than by export demands or final consumer demands. This underscores the crucial role that investment plays in shaping the carbon footprint of the automobile manufacturing sector and its supply chain.
The driving mechanism of GHG emissions through the supply chain
Spillover emissions can serve as a mirror, reflecting the driving forces behind GHG emissions from both within and outside a subsystem. Given that spillover emissions from China’s automobile manufacturing industry constitute a significant portion of total emissions, a deeper dive into these emissions across various associated industrial sectors is imperative to uncover the intricate mechanisms driving GHG emissions within the automotive industry’s supply chain.
To achieve this, our paper performs an industrial linkage analysis of the spillover effects of GHG emissions in China’s automobile manufacturing sector using Sankey diagrams. This analysis aims to pinpoint the key contributors to spillover emissions, capturing the contribution ratios of 96% across all relevant industrial sectors [Figure 1]. The diagram reveals five top industrial sectors, including ferrous metal smelting and rolling, electricity and heat production, chemical products manufacturing (excluding petroleum products), petroleum refining, and glass manufacturing, collectively accounting for over 75% of the total contributions, as the “major contributors”. The remaining sectors are grouped under “other sectors”, within which non-ferrous metal production is notably highlighted due to its significance in the production of new energy vehicles.
Figure 1. Spillover emissions of other industrial sectors for the automobile manufacturing industry [Values in kilotons of CO2 equivalent (kt CO2-eq)].
Prominently, the steel-making sector stands out as the largest contributor, a fact that has been consistently recognized in previous research[13,58]. This sector has been heavily influenced by massive investments over the years, leading to the establishment of a high-carbon emission production model characterized by long production processes. As a result, significant capacities have been built up, primarily relying on carbon-intensive processes.
Looking ahead, there is an urgent need for forces from downstream sectors to catalyze a transition in the upstream steel industry. This transition should prioritize the adoption of low-carbon technologies, particularly shifting toward short-process steel production utilizing scrap steel recycling. Such a shift is crucial not only for reducing the carbon footprint of the automotive industry but also for aligning the upstream steel sector with global efforts to mitigate climate change.
Furthermore, the second largest contributor relates to energy consumption in production processes, highlighting the need for efficiency improvements and the integration of renewable energy sources. The third and fourth contributors, closely linked to plastics production, also underscore the importance of exploring sustainable alternatives and circular economy practices within these sectors.
In general, the spillover emissions analysis conducted in this paper reveals critical insights into the driving forces behind GHG emissions in China’s automobile manufacturing industry and its supply chain. Addressing these emissions will require concerted efforts across multiple industrial sectors, with a particular focus on transforming the upstream material industry through a systematic transition toward a circular economy, driven by downstream eco-design for low-carbon development.
Scenario comparison of different circular economy strategies
Figure 2 presents an illustration of the shifts in GHG emissions within China’s automobile manufacturing sector across various scenarios. A notable challenge emerges in the form of the relentlessly expanding final demand, posing a substantial barrier to the reduction of GHG emissions generated by this industry. When juxtaposed with the baseline scenario in 2018, where emissions stood at 434.76 million tons, it becomes evident that a lack of a more progressive low-carbon transition or the development of a circular economy could lead to a dramatic escalation in overall emissions. Specifically, under a scenario that merely accounts for a low-carbon energy transition without addressing the underlying demand growth, emissions are projected to soar to 734.56 million tons. This finding resonates with prior research[59] that examined the rebound effects of new energy vehicle development from a macroeconomic perspective. The research underscores that, even as the adoption of new energy vehicles increases, the overall lifecycle energy consumption may shift upstream within the supply chain. Consequently, merely transitioning to cleaner energy sources within the automotive sector is not sufficient to mitigate emissions comprehensively. It necessitates a holistic approach that encompasses the entire production chain, including upstream processes, to effectively curb the rise in GHG emissions.
Figure 2. Scenario comparison of GHG emissions by China’s automobile manufacturing with different strategies of circular economy. note: in scenario 3, there are two results: 192.86 million tons, corresponding to 13% for the lowest reduction potential, and 95.32 million tons, corresponding to 57% for the highest reduction potential, according to IRP’s estimation[40], to illustrate the impact of this factor on overall GHG emission reduction in scenario 3.
Secondly, in contrast to the baseline scenario, a progressive low-carbon energy transition stands out as the most impactful measure in reducing GHG emissions from China’s automobile manufacturing sector, with the potential to contribute to approximately 60% of the total emission reduction. Assuming the same level of automobile production output in 2030, this transition could lead to a substantial decrease in total GHG emissions, slashing them by around one-third compared to 2018 levels. The significance of a low-carbon energy transition extends beyond the manufacturing phase; it also plays a pivotal role in reducing emissions during the use stage of automobiles, particularly for electric vehicles. Consequently, our study reinforces the current strategic direction of the automobile industry and national policies that prioritize low-carbon energy transitions[18]. This alignment underscores the critical importance of adopting comprehensive and forward-thinking approaches to mitigate the environmental impact of the automobile sector. In essence, a robust commitment to low-carbon energy transitions is not just a desirable goal for one sector, but a necessity for achieving significant GHG emission reductions through systemic transformation toward low-carbon energy infrastructure.
Thirdly, our research results show significant potential in the circular economy to further reduce GHG emissions induced by automobile manufacturing. In the scenario of closed-loop material recycling, with only improving the recycling rate of steel and plastics, the total emission can be reduced up to about 10% compared to the baseline scenario. The input-output table we used was made in 2018 when the market share of electric vehicles was still very low in China. Therefore, the data do not illustrate the non-ferrous metal used in battery production. However, according to the LCA of electric vehicles, battery production accounts for a significant portion of emissions, in which non-ferrous metals play a critical role in the supply chain[16]. The potential of closed-loop material recycling in the context of electrification of automobiles will be even higher than our estimation.
Finally, the potential contribution of shared mobility to GHG emission reductions could range from 4% to 18%, contingent upon the extent to which it reduces vehicle demand. This estimation has considerable uncertainty, primarily because the shifts in mobility patterns driven by autonomous driving technology are currently difficult to predict with precision. Mainstream research suggests that shared mobility will lead to a decrease in car sales, thereby mitigating the associated environmental burdens[56,60,61]. To reflect this possibility, we present a range of potential outcomes based on the United Nations Environment Programme’s (UNEP) estimates regarding the impact of shared mobility on vehicle demand[57]. However, it is important to acknowledge that some research has highlighted the risk of rebound effects in the development of autonomous electric vehicles[62]. These rebound effects could potentially offset some of the environmental benefits anticipated from shared mobility. Ultimately, the true trajectory of these changes will be heavily influenced by future urban planning and infrastructure development, as well as the evolution of new technologies. Therefore, while shared mobility presents a promising avenue for GHG emission reductions, its full impact remains contingent upon a multitude of factors that will shape the future of urban mobility.
Implications for various stakeholders
Based on the aforementioned research findings, the following action recommendations are proposed for various relevant stakeholders:
First, to the national policymakers, it is imperative to champion a progressive low-carbon energy transition. This transition demands a profound transformation of the energy infrastructure, with extensive ramifications for nearly all industrial sectors, including the automobile manufacturing industry. Our estimates reveal that, in comparison to the existing national plan for low-carbon energy development, a minimum of 60% of the overall GHG emissions generated by automobile manufacturing in China can be mitigated. This substantial reduction underscores the significant environmental benefits that can be achieved by adopting a more sustainable energy pathway.
Second, it is pivotal for automobile manufacturers to coordinate the entire supply chain in their GHG mitigation efforts. By boosting the closed-loop recycling rate, they have the potential to dramatically decrease emissions. For instance, merely enhancing the recycling of steel and plastic can reduce 72.1418 million tons of GHG emissions, equivalent to approximately a 10% decrease. To accomplish this, collaboration across the entire supply chain is essential, ensuring that materials are efficiently collected for recovery, reprocessing, and reuse. The implementation of EPR can serve as a potent incentive for automobile producers to forge closed-loop recycling chains with other stakeholders, thereby fostering a more circular economy. On the other hand, the low-carbon commitments among automotive producers have played a pivotal role in driving the improvement of the circular supply chain. By prioritizing environmental sustainability and efficiency, automotive firms are incentivized to adopt practices that enhance material recycling, reduce waste, and optimize resource utilization. This not only contributes to mitigating greenhouse gas emissions but also strengthens their competitive edge in the market, as consumers and regulators increasingly value environmentally responsible business practices. Consequently, the push for low-carbon competition encourages automakers to invest in and refine their circular supply chains, fostering a more sustainable and resilient industry.
Furthermore, for the urban and regional authorities, the advent of shared mobility presents a promising avenue for further GHG emission reductions. By diminishing the demand for automotive products while still fulfilling travel requirements, shared mobility can contribute to an additional 4% reduction in emissions. However, harnessing the full potential of shared mobility necessitates the development of innovative business models and urban design strategies. This endeavor requires a multidisciplinary approach, bringing together policymakers, industry leaders, urban planners, and other stakeholders to cultivate a supportive ecosystem that enables shared mobility to flourish. By fostering collaboration and innovation across these diverse domains, we can pave the way for a more sustainable and environmentally friendly future in the automobile sector.
Last but not least, this research emphasizes the pivotal role of educational innovation in fostering a generation of design and business students who can adopt a broader, more holistic view of the automotive industry’s transition toward low-carbon products. Central to this endeavor is the integration of circular design principles with low-carbon design methodologies, creating a synergy that promises transformative impact. The economic and strategic dimensions of low-carbon design and circular economy practices need to be integrated to respond to the financial incentives, policy landscapes, and market trends that drive low-carbon development in the automotive sector. Crucially, it is essential to foster interdisciplinary collaboration between design and business students by promoting a deeper appreciation of the interconnectedness of design, business, and sustainability.
CONCLUSION
China’s automobile manufacturing industry possesses substantial potential for mitigating GHG emissions through the adoption of circular economy strategies. Our analysis unveils that emissions from spillover components, impacting the upstream raw material supply chain, constitute over 98% of the sector’s total emissions. This highlights the crucial need to consider the broader economic system in formulating decarbonization strategies.
The study illustrates that while transitioning to a low-carbon energy structure can significantly cut GHG emissions by approximately 60%, there remains considerable scope for further reductions through circular economy practices. Specifically, boosting the closed-loop recycling rates of materials like steel and plastics can yield an additional 10% reduction in GHG emissions. Furthermore, the development of shared mobility services presents an opportunity to decrease demand for automotive products, leading to an extra 4% to 18% reduction in emissions, contingent on the development of new mobility models.
Nonetheless, this paper acknowledges certain limitations. Firstly, the input-output methodology employs value quantities instead of physical quantities, introducing a bias compared to product lifecycle assessments grounded in material flows. This discrepancy may affect the accuracy and pertinence of our findings, especially in assessing environmental impacts. Secondly, there is a notable time lag in compiling environmental input-output tables, failing to capture the swift evolution of China’s electric vehicle market. As a result, our analysis does not fully mirror the latest advancements and dynamics within the industry. Future research is expected to address these limitations, offering a more comprehensive and up-to-date understanding of the subject.
To achieve substantial GHG emission reductions in the automobile industry, it is imperative to embrace a holistic approach that integrates energy transition, material recycling, and mobility innovation. All stakeholders must collaborate to effectively develop and implement these strategies, balancing both economic and environmental considerations, in pursuit of carbon neutrality.
DECLARATIONS
Acknowledgement
We would like to thank Prof. Xianlai Zeng and Prof. Ming Xu from Tsinghua University, and
Authors’ contributions
Conceptualization, methodology, investigation, resources, writing - original draft, writing - review & editing, formal analysis, supervision, project administration, funding acquisition: Tong X
Conceptualization, methodology, software, formal analysis, investigation, data curation, visualization: Gao J
Conceptualization, methodology, software, formal analysis, visualization: Wang T
Conceptualization, investigation, resources: Shi X
Availability of data and materials
The data are available from the corresponding author upon reasonable request. Regarding carbon emissions by automobile manufacturing and other industries, the data are originally from the Chinese environmentally extended input-output (CEEIO) database developed by Prof. Ming Xu at the University of Michigan in collaboration with Prof. Sai Liang at Guangdong University of Technology and Prof. Xi Tian at Nanchang University[28,29].
Financial support and sponsorship
This research falls under one of the projects of the Erasmus Initiative: Dynamics of Inclusive Prosperity, a joint project funded by the National Natural Science Foundation of China (NSFC) and the Dutch Research Council (NWO): “Towards Inclusive Circular Economy: Transnational Network for Wise-waste Cities (IWWCs)” (NSFC project number: 72061137071; NWO project number: 482.19.608). The fieldwork and data acquisition were supported by the National Key R&D Program of China on Solid Waste Management [grant number 2018YFC1900101].
Conflicts of interest
All authors declared that there are no conflicts of interest. Shi X is affiliated with China Automotive Technology & Research Center Co., Ltd.
Ethical approval and consent to participate
Not applicable.
Consent for publication
Not applicable.
Copyright
© The Author(s) 2024.
REFERENCES
1. IEA. Outlook for emissions reductions; 2024. Available from: https://www.iea.org/reports/global-ev-outlook-2024/outlook-for-emissions-reductions [Last accessed on 26 Dec 2024].
2. Montemayor HM, Chanda RH. Automotive industry's circularity applications and industry 4.0. Environ Chall 2023;12:100725.
3. Shahzad K, Iqbal Cheema I. Low-carbon technologies in automotive industry and decarbonizing transport. J Power Sources 2024;591:233888.
4. Zhong S, Liu A, Jiang Y, et al. Energy and environmental impacts of shared autonomous vehicles under different pricing strategies. NPJ Urban Sustain 2023;3:92.
5. Geissdoerfer M, Savaget P, Bocken NMP, Hultink EJ. The circular economy - a new sustainability paradigm? J Clean Prod 2017;143:757-68.
6. Intergovernmental Panel on Climate Change (IPCC). Climate change 2022 - mitigation of climate change: working group III contribution to the sixth assessment report of the Intergovernmental panel on climate change. Cambridge University Press; 2022.
7. He Z, Sun L, Hijioka Y, Nakajima K, Fujii M. Systematic review of circular economy strategy outcomes in the automobile industry. Resour Conserv Recycl 2023;198:107203.
8. Ellen MacArthur Foundation. The circular economy opportunity for urban and industrial innovation in China; 2018. Available from: https://www.ellenmacarthurfoundation.org/urban-and-industrial-innovation-in-china [Last accessed on 26 Dec 2024]
9. da Costa VBF, Bitencourt L, Dias BH, Soares T, de Andrade JVB, Bonatto BD. Life cycle assessment comparison of electric and internal combustion vehicles: a review on the main challenges and opportunities. Renew Sustain Energy Rev 2025;208:114988.
10. Ajanovic A, Haas R. Renewable energy systems implementation in road transport: prospects and impediments. Renew Energy Environ Sustain 2021;6:39.
11. Yuan M, Thellufsen JZ, Lund H, Liang Y. The electrification of transportation in energy transition. Energy 2021;236:121564.
12. Buberger J, Kersten A, Kuder M, Eckerle R, Weyh T, Thiringer T. Total CO2-equivalent life-cycle emissions from commercially available passenger cars. Renew Sustain Energy Rev 2022;159:112158.
13. Shen J, Zhang Q, Tian S. Decarbonization pathways analysis and recommendations in the green steel supply chain of a typical steel end user-automotive industry. Appl Energy 2025;377:124711.
14. Billy RG, Müller DB. Aluminium use in passenger cars poses systemic challenges for recycling and GHG emissions. Resour Conserv Recycl 2023;190:106827.
15. Ravina M, Bianco I, Ruffino B, Minardi M, Panepinto D, Zanetti M. Hard-to-recycle plastics in the automotive sector: economic, environmental and technical analyses of possible actions. J Clean Prod 2023;394:136227.
16. Baars J, Domenech T, Bleischwitz R, Melin HE, Heidrich O. Circular economy strategies for electric vehicle batteries reduce reliance on raw materials. Nat Sustain 2021;4:71-9.
17. Gao Z, Xie H, Yang X, et al. Electric vehicle lifecycle carbon emission reduction: a review. Carbon Neutral 2023;2:528-50.
18. Automotive Data of China (Tianjin) Co., L. For carbon neutrality low carbon development strategies and transformation pathways of automobile industry. Beijing: China Machine Press; 2022. Available from: https://www.dedao.cn/ebook/detail?id=rEQKv6PKN7rEo2Gxg96ZjApyMvQVlw5jb9r0Xb14PJzDkYaReqd8n5LOmB8d7egB [Last accessed on 26 Dec 2024]
19. de Blas I, Mediavilla M, Capellán-Pérez I, Duce C. The limits of transport decarbonization under the current growth paradigm. Energy Strategy Rev 2020;32:100543.
20. Elassy M, Al-Hattab M, Takruri M, Badawi S. Intelligent transportation systems for sustainable smart cities. Transp Eng 2024;16:100252.
21. Cao Y, Derrible S, Le Pira M, Du H. Advanced transport systems: the future is sustainable and technology-enabled. Sci Rep 2024;14:9429.
22. Aderibigbe OO, Gumbo T. Smart cities and their impact on urban transportation systems and development. In: Emerging technologies for smart cities. Cham: Springer; 2024, pp. 105-29.
23. Narayanan S, Chaniotakis E, Antoniou C. Shared autonomous vehicle services: a comprehensive review. Transp Res Part C 2020;111:255-93.
24. Kagawa S, Nansai K, Kondo Y, et al. Role of motor vehicle lifetime extension in climate change policy. Environ Sci Technol 2011;45:1184-91.
25. Santos AS, de Abreu VHS, de Assis TF, Ribeiro SK, Ribeiro GM. An overview on costs of shifting to sustainable road transport: a challenge for cities worldwide. In: Environmental footprints and eco-design of products and processes. Singapore: Springer; 2021. pp. 93-121.
26. Nieuwenhuijsen MJ. Urban and transport planning pathways to carbon neutral, liveable and healthy cities; A review of the current evidence. Environ Int 2020;140:105661.
27. Moslem S, Campisi T, Al-Rashid MA, Simic V, Esztergár-Kiss D, Pilla F. Greening urban mobility: assessing environmental and functional characteristics of bicycle infrastructure in the post-pandemic Era. Habitat Int 2024;153:103200.
28. Mahajan S, Argota Sánchez-Vaquerizo J. Global comparison of urban bike-sharing accessibility across 40 cities. Sci Rep 2024;14:20493.
29. Veitch E, Rhodes E. A cross-country comparative analysis of congestion pricing systems: lessons for decarbonizing transportation. Case Stud Transp Policy 2024;15:101128.
30. Gonzalez JN, Gomez J, Vassallo JM. Are low emission zones and on-street parking management effective in reducing parking demand for most polluting vehicles and promoting greener ones? Transp Res Part A 2023;176:103813.
31. Boonman H, Verstraten P, van der Weijde AH. Macroeconomic and environmental impacts of circular economy innovation policy. Sustain Prod Consump 2023;35:216-28.
32. Solaymani S. CO2 emissions patterns in 7 top carbon emitter economies: the case of transport sector. Energy 2019;168:989-1001.
33. Firlej M, Taeihagh A. Regulating human control over autonomous systems. Regul Gov 2021;15:1071-91.
34. Chenavaz RY, Dimitrov S. From waste to wealth: Policies to promote the circular economy. J Clean Prod 2024;443:141086.
35. Tong X, Wang T, Li J, Wang X. Extended producer responsibility to reconstruct the circular value chain. Circular Econ 2024;3:100076.
36. Wang Z, Li X, Xue X, Liu Y. More government subsidies, more green innovation? The evidence from Chinese new energy vehicle enterprises. Renew Energy 2022;197:11-21.
37. Xu X, Zhang W, Wang T, Xu Y, Du H. Impact of subsidies on innovations of environmental protection and circular economy in China. J Environ Manag 2021;289:112385.
38. Genc TS. A circular economy with tax policy: using collection channels and returns to mitigate distortions in steel production and recycling. J Clean Prod 2024;451:142120.
39. Mies A, Gold S. Mapping the social dimension of the circular economy. J Clean Prod 2021;321:128960.
40. Wang L, Zhu S, Evans S, Zhang Z, Xia X, Guo Y. Automobile recycling for remanufacturing in China: a systematic review on recycling legislations, models and methods. Sustain Prod Consump 2023;36:369-85.
41. Peng J, Shi X, Tong X. Extended producer responsibility for low carbon transition in automobile industry. Circular Econ 2023;2:100036.
42. Yu Z, Umar M, Rehman SA. Adoption of technological innovation and recycling practices in automobile sector: under the Covid-19 pandemic. Oper Manag Res 2022;15:298-306.
43. Liu M, Yang X, Wen J, et al. Drivers of China’s carbon dioxide emissions: based on the combination model of structural decomposition analysis and input-output subsystem method. Environ Impact Assess Rev 2023;100:107043.
44. Alcántara V, Padilla E. Input-output subsystems and pollution: an application to the service sector and CO2 emissions in Spain. Ecol Econ 2009;68:905-14.
45. Llop M, Tol RS. Decomposition of sectoral greenhouse gas emissions: a subsystem input-output model for the Republic of Ireland. J Environ Plan Manag 2013;56:1316-31.
46. Liang S, Feng T, Qu S, Chiu AS, Jia X, Xu M. Developing the Chinese environmentally extended input-output (CEEIO) database. J Ind Ecol 2017;21:953-65.
47. Tian X, Liu Y, Xu M, Liang S, Liu Y. Chinese environmentally extended input-output database for 2017 and 2018. Sci Data 2021;8:256.
48. Sun YF, Yu S, Zhang YJ, Su B. How do imports change the energy consumption of China? An analysis of its role in intermediate inputs and final demands. Energy 2023;270:126947.
49. Department of Energy Statistics; National Bureau of Statistics. China energy statistical yearbook 2021. Beijing: China Statistics Press; 2022. Available from: https://cnki.nbsti.net/CSYDMirror/Trade/yearbook/single/N2022060061?z=Z024https://cnki.nbsti.net/CSYDMirror/Trade/yearbook/single/N2022060061?z=Z024 [Last accessed on 26 Dec 2024]
50. State Council of China. Action plan for carbon peak before 2030. Available from: https://www.gov.cn/zhengce/content/2021-10/26/content_5644984.htm [Last accessed on 26 Dec 2024]
51. China Association of Automobile Manufacturers. China market medium and long term forecast report (2020-2035). Beijing: China Association of Automobile Manufacturers; 2020. Available from: https://news.qq.com/rain/a/20201215A0G5K100 [Last accessed on 26 Dec 2024]
52. Shi D. Report on the frontiers of China's energy development (2021). Beijing: Social Sciences Academic Press; 2022. Available from: https://www.pishu.com.cn/skwx_ps/bookdetail?SiteID=14&ID=13720050 [Last accessed on 26 Dec 2024]
53. Material Economics. The circular economy-a powerful force for climate mitigation. 2023. Available from: https://materialeconomics.com/publications/the-circular-economy-a-powerful-force-for-climate-mitigation-1 [Last accessed on 26 Dec 2024]
54. Jiang XB, Jiang J, Chen DJ, Zhou WJ, Zhu B. Dynamic material flow analysis of Chinese passenger car plastics. China Environ Sci 2020;40:4106-14. Available from: http://www.zghjkx.com.cn/EN/abstract/abstract17142.shtml [Last accessed on 26 Dec 2024]
55. Wang Y, Zhang ZY. The design and application of based on the input-output system RAS method. Stat Educ 2008;11:16-20. Available from: https://www.docin.com/p-758567425.html [Last accessed on 28 Dec 2024]
56. Morfeldt J, Johansson DJA. Impacts of shared mobility on vehicle lifetimes and on the carbon footprint of electric vehicles. Nat Commun 2022;13:6400.
57. UNEP. Resource efficiency and climate change: material efficiency strategies for a low-carbon future; 2020. Available from: https://www.unep.org/resources/report/resource-efficiency-and-climate-change-material-efficiency-strategies-low-carbon [Last accessed on 26 Dec 2024].
58. Yao S, Zhu H, Zhang S, Chang H, Wang H. Green steel: the future path towards sustainable automotive manufacturing. Resour Conserv Recyc 2024;200:107319.
59. Vivanco D, Freire-González J, Kemp R, van der Voet E. The remarkable environmental rebound effect of electric cars: a microeconomic approach. Environ Sci Technol 2014;48:12063-72.
60. Heineke K, Laverty N, Möller T, Ziegler F. The future of mobility. McKinsey; 2023. Available from: https://www.mckinsey.com/industries/automotive-and-assembly/our-insights/the-future-of-mobility-mobility-evolves [Last accessed on 28 Dec 2024]
61. Greenblatt JB, Shaheen S. Automated vehicles, on-demand mobility, and environmental impacts. Curr Sustain Renew Energy Rep 2015;2:74-81.
Cite This Article
How to Cite
Download Citation
Export Citation File:
Type of Import
Tips on Downloading Citation
Citation Manager File Format
Type of Import
Direct Import: When the Direct Import option is selected (the default state), a dialogue box will give you the option to Save or Open the downloaded citation data. Choosing Open will either launch your citation manager or give you a choice of applications with which to use the metadata. The Save option saves the file locally for later use.
Indirect Import: When the Indirect Import option is selected, the metadata is displayed and may be copied and pasted as needed.
About This Article
Special Issue
Copyright
Data & Comments
Data
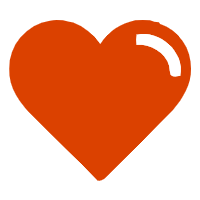
Comments
Comments must be written in English. Spam, offensive content, impersonation, and private information will not be permitted. If any comment is reported and identified as inappropriate content by OAE staff, the comment will be removed without notice. If you have any queries or need any help, please contact us at [email protected].