Synthesis and regulation strategies for enhancing the electrochemical performance of sodium-ion battery anode materials
Abstract
Rechargeable sodium-ion batteries (SIBs) have attracted increasing research interest because of their inherent advantages such as a similar working principle to lithium-ion batteries (LIBs) and plentiful, even-distributed, and inexpensive Na resources. However, Na ions possess a larger ionic radius (1.07 Å) compared to that of Li ions (0.76 Å), which brings key challenges such as irreversible capacity loss, sluggish kinetics, a considerable volume expansion, and low initial coulombic efficiency (ICE) of SIBs, especially for anodes. Despite these challenges, there have been ongoing efforts to develop various synthesis and regulation strategies to enhance the electrochemical performance of SIB anode materials and they are summarized here. In this review, the significance of developing SIBs, the types of SIB anode materials, and the key issues SIB anode materials face are discussed first. Then various developed synthesis and regulation strategies such as compositional, structural, and interfacial regulations based on different synthesis methods to enhance the electrochemical performance of SIB anode materials are summarized. Finally, in conclusions and outlooks, the present status of SIB anode materials is concluded and the future development directions are proposed.
Keywords
INTRODUCTION
Since the second industrial revolution, mankind has entered the electrical era and the use of electricity has entered a period of vigorous development. However, the excessive depletion of fossil fuels has resulted in resource and environmental issues. Thus, people have turned their attention to renewable energy such as solar and wind energy. The development and use of renewable energy have become a pivotal strategy in promoting the transformation of energy towards clean and low-carbon ways, as well as in ensuring energy security. However, these kinds of energy strongly depend on the natural environment and their supply is not stable, which has a great influence on the power supply if they are directly integrated into the power grid. Driven by this background and low-carbon and environmentally friendly production and lifestyle, new energy storage techniques represented by electrochemical energy storage, especially rechargeable batteries, are expected to become the core solution for large-scale grid-connection of renewable energy and smooth power grid fluctuations. Among various rechargeable batteries, lithium-ion batteries (LIBs) have an expansive range of applications within the energy market due to their elevated energy density and extended cycling lifespan. This is particularly the case in the field of power batteries; LIBs have a continuously expanding leading advantage[1-3]. Nevertheless, the limited and uneven distribution of Li resources within the earth’s crust leads to relatively elevated costs, which poses great challenges to the ever-growing demand of the energy market[4,5]. Especially since 2021, the price of lithium carbonate has continued to run at a high level, making it challenging to support large-scale energy storage and the expanding electric vehicle market. Therefore, it is urgently and highly desired to develop supplement or alternative energy storage techniques of LIBs to relieve energy demand. Sodium-ion batteries (SIBs)[6-8], organic batteries[9,10], aqueous batteries[11,12], and other resource-rich, environmentally friendly, and sustainable rechargeable battery techniques therefore have attracted increasing research interest.
SIBs have shown appealing potential because of their advantages such as a similar working principle with LIBs, abundant, even-distributed, and low-cost sodium resources, and higher safety and superior electrochemical performance at low temperatures compared to LIBs. Currently, the energy density of the SIBs is about 100-150 Wh·kg-1 (the ternary LIBs is approximately 250 Wh·kg-1), and the cycle life of SIBs is around 65% of that of the LIBs. Although currently the energy density and cycle life of SIBs still lag behind those of LIBs, the resource, cost, safety, and low-temperature fast-charging advantages make SIBs a promising candidate to supplement or replace the applications of LIBs in some fields, such as energy storage power stations, electric vehicles, and start-stop batteries. As shown in Figure 1A, the structure and operational principles of SIBs are analogous to those of LIBs, mainly including a cathode, an anode, an electrolyte, and a separator. The cathode materials[13] of SIBs mainly include layered metal oxide[14], Prussian blue and its analogs[15], polyanion compounds[16], and oxalate-based[17], which have made a great development. However, as one of the fundamental components of SIBs, the electrochemical performance of anodes still requires improvement to match the performance of cathodes and thus facilitates the commercialization of SIBs[18].
Figure 1. (A) Schematic diagram of the operational principle of SIBs; (B) Number of publications on SIB anodes in the past decade; (C) Different types of SIB anode materials. Quoted with permission from Huang et al.[6]; (D) Reaction mechanism schematics of intercalation. Quoted with permission from Qiao et al.[7]; (E) The mechanism of Na storage in HC. Quoted with permission from Chen et al.[19]; (F and G) Reaction mechanism schematics of conversion and alloying. Quoted with permission from Qiao et al.[7]. SIBs: Sodium-ion batteries; HC: hard carbon.
Anode materials account for about 14% of the total cost of SIBs and their performance influences the overall performance of a full battery significantly. As can be observed in Figure 1B, based on the data obtained from the Web of Science, the publication numbers of SIB anodes have increased dramatically since 2014 due to their important role in energy development. The ideal SIB anode materials should satisfy the following criteria: (1) To obtain high volume-specific capacity and mass-specific capacity, an elemental mass of the anode materials should be light and density should be small and the material should be able to store as much Na+ as possible; (2) The potential should be approximately equivalent to that of sodium metal to enhance the operating voltage of the full battery; (3) The electrode should be stable in the electrolyte; (4) It is essential that the materials display optimal electronic and ionic conductivity; (5) The materials employed are sustainable, cost-effective, and environmentally friendly[8,16,18]. Currently, as shown in Figure 1C, there are three main types of anode materials based on their Na+ storage mechanisms: intercalation, conversion, and alloying.
The intercalation type anode materials primarily consist of carbonaceous materials[19-21] and titanium-based oxides[22,23], in which the Na ions de/intercalate into the lattice of the materials for storage with negligible structural changes illustrated in Figure 1D. The stable structure of intercalation-type anode materials during the electrochemical de/sodiation process leads to good cycling stability. Carbonaceous materials mainly consist of graphite carbon and non-graphite carbon. Graphite carbon has a stable lamellar structure and excellent electrical conductivity. It has been successfully introduced to the market as an anode material for LIBs. However, it is challenging for Na ions to intercalate into the layers of graphite due to their bigger ionic radius compared to Li ions. Non-graphite carbon has amorphous structures, thus not having obvious characteristic graphite diffraction peaks. The interlayer spacing is larger, the presence of defects is more pronounced, and the microcrystalline structure is more disordered in comparison to graphite carbon, which is favorable for the storage of Na-ion. According to the degree of graphitization, non-graphite carbon can be classified into soft carbon (SC) and hard carbon (HC)[19,20].
SCs are carbon materials that have undergone a high degree of graphitization after high temperature (over 2,800 °C) treatment while HC usually refers to carbon materials that are resistant to graphitization even under high-temperature treatment. SC includes carbon microspheres, needle coke, petroleum coke, etc. Compared to HC, SC exhibits a more ordered structure, a smaller interlayer spacing, a lower specific surface area, and a less microporous structure while these features are not conducive to sodium ion storage and diffusion. It has been reported that all SC materials suffer from insufficient sodium storage capacity (approximately 200 mAh·g-1) and a relatively high potential for sodium storage (exceeding 0.5 V vs. Na+/Na). These factors impose significant limitations on the energy density of the Na-ion full-cell systems[1,4].
HC includes resin carbon, organic polymer pyrolytic carbon, carbon black, etc. It has a short-range ordered and long-range disordered structure, shows a graphite microcrystalline structure, and contains a high density of pores and defects. Based on these structural features, the main sodium storage active sites of HC include surface open pores, defect sites on graphite-like layers, interlayer spacing between graphite-like layers, and close cavities. Accordingly, there are four proposed sodium ion storage mechanisms: the “three-stage” model, “adsorption-filling” model, “insertion-adsorption” model, and the “adsorption-intercalation” model as illustrated in Figure 1E, for details please see the references[7,19,21]. The defects, disordered structure, and large layer spacing of HC synergistically improve its specific capacity. Additionally, HC has a low sodium storage potential (0.1 V vs. Na+/Na) and is not soluble in electrolytes. Together with the features of broad resources, low cost, and environmental sustainability, HC becomes the most appealing and promising anode material of SIBs[20], whereas the significant quantity of defects of HC leads to irreversible Na+ sites and thus has a relatively low initial coulombic efficiency (ICE) and an unsatisfied cycling life. Moreover, the disordered structure leads to relatively low conductivity and thus has an unsatisfied rate capability. Titanium-based oxides, which include TiO2 and Na2Ti3O7 (NTO), have become a crucial component of SIB anodes due to their structural stability, redox reaction potential platform stability (0.75 V vs. Na+/Na), inexpensiveness, and environmental friendliness. However, inadequate electrical conductivity leads to tardy ion mobility and poor rate capability. Additionally, the titanium-based oxide anode materials have a low ICE as well[22,23].
The conversion-type materials mainly include transition metal sulfides (TMSs) and transition metal oxides (TMOs), which store Na+ through conversion reactions. During the conversion reaction process, sodium ions replace transition metals and react with sulfur or oxygen in transition metal compounds to generate elemental transition metals and sodium sulfides or sodium oxides [Figure 1F]. The conversion reaction process involves the chemical transformation of one or more atoms, which is a phase transition reaction that involves the disruption and subsequent formation of chemical bonds, culminating in the creation of novel compounds. Due to the redox reactions involving multi-electron transfer during de/sodiation, conversion-type anode materials possess a high theoretical specific capacity ranging from 200-
However, the sodium storage process causes serious and irreversible volume expansion (200%-400%) [Figure 1G], resulting in pulverization of the electrode and rapid attenuation of specific capacity[28-30]. The alloying-type materials therefore have poor cycling stability.
To solve the discussed problems of SIB anodes, it is essential to regulate the electrode materials and electrolytes to achieve enhanced electrochemical performance. The main regulation strategies include[31-40]: composition regulation, such as heteroatom doping, composite construction, and formation of heterostructure; structural regulation, such as nanostructure design, morphology control, and defect introduction; interfacial regulation, such as the regulation of electrode materials and electrolyte salts, solvents and additives to form a stable solid electrolyte interphase (SEI). In the following sections, various regulation strategies and their corresponding enhanced electrochemical performances of each type of SIB anode material will be introduced in detail with selected examples. It should be noted that most references in this review will focus on half-cells and it will be clarified when the data refer to the few full-cell cases. We aim to introduce versatile strategies for the optimization of sodium ion storage performance of anode materials.
COMPOSITIONAL REGULATIONS
Composition regulation mainly includes heteroatom doping, composite construction, and formation of heterostructure. Heteroatom doping refers to the introduction of heteroatoms or ions into a material to alter its microstructure, change its electronic state, and improve its electronic conductivity to improve its specific capacity and rate capability used as an anode material for SIBs[31,32,34]. In addition to heteroatom doping, incorporating porous carbon materials into anode materials to construct composite materials with improved ionic and electronic conductivity is also an effective strategy for obtaining high-performance anode materials. Furthermore, introducing a second or third component into anode materials to form heterogeneous structures can validly enhance the electrochemical performance of the anode materials due to the synergistic effect of all components[31-40]. The electrochemical performance of various SIB anode materials after compositional regulations is shown in Table 1.
The electrochemical performance of various SIB anode materials after compositional regulations
Material | Mechanism | Regulation strategy | ICE (%) | Current density (mA·g-1) | Cycles | Capacity retention (mAh·g-1) | Ref. |
PO-SC-S | Intercalation | Composition | 80 | 750 | 2,000 | 249 | [43] |
NPUCS | Intercalation | Composition | 75 | 5,000 | 2,000 | 110 | [44] |
NPDC (PDC-700) | Intercalation | Composition | 44.4 | 2,000 | 2,000 | 197 | [46] |
NS-TiO2 | Intercalation | Composition | 45.2 | 3,350 | 2,400 | 157.4 | [48] |
H-TiO2@CFC | Intercalation | Composition | 77.4 | 3,350 | 600 | 171.3 | [50] |
CC-ZnS/CNT | Conversion | Composition | 82 | 5,000 | 500 | 314 | [57] |
Ni3S2-N-rGO 700 °C | Conversion | Composition | 68 | 2,000 | 400 | 377 | [59] |
Fe7Ni3S11/CN | Conversion | Composition | 90 | 2,000 | 900 | 477 | [61] |
Sb2O3@Sb | Alloying | Composition | 67.9 | 200 | 200 | 658 | [63] |
NP-SnSb | Alloying | Composition | 60 | 200 | 240 | 388.1 | [67] |
HC
Pei et al. devised a novel protocol for in-situ texture creation utilizing sulfuric acid polymerized sucrose and trithiocyanuric acid as starting materials to fabricate N and S double-doped porous HC (SNC-P) material with increased layer spacing, appropriate doping and defect concentrations, thin carbon shells, and pore classification[41]. This material exhibited a notable reversible specific capacity of 430 and 277 mAh·g-1 at 0.05 and 5.0 A·g-1, along with exceptional cyclic stability for Na+ storage. Jin et al. synthesized N and S co-doped micro/nanoparticles as an anode material for SIB through a one-step approach involving thiourea and sodium citrate grounded on the pseudo-capacitance mechanism[42]. Upon fine-tuning, the specific capacity of NSC2 (thiourea to sodium citrate mass ratio of 2:1) was recorded as 280 and 223 mAh·g-1 at 0.05 and
Figure 2. (A) Schematic illustration of the heteroatom configuration screening strategy for the synthesis of PO-SC-S as an anode material of SIBs and its corresponding (B) Rate performance of full cells coupled with an O3- or P2/O3-type cathode with C-rates from 0.1 to 20 C. Quoted with permission from Xie et al.[43]; (C) Synthesis scheme of NPUCS and NUCS and their corresponding (D) Rate capability. Quoted with permission from Wu et al.[44]; (E) Schematic illustration of the synthesis process of UPCs; (F) Rate capability of UPC-6. Quoted with permission from Yan et al.[47]. PO-SC-S: The pristine SC was co-doped with P/O and subsequently carbonized on two occasions at 800 °C; SIBs: sodium-ion batteries; NPUCS: N and P co-doped ultramicropores HC spheres; NUCS: N-doped ultra-micropores carbon spheres; UPCs: UPC-6: phosphorus-doped carbon with molar ratios n (PCl3) : n (C6H12) = 6:1; SC: soft carbon.
Mehmood et al. proposed a highly nanoporous (NP) carbon material doped with nitrogen created through the ionic carbonization of the Zn-imidazolium framework[45]. This material demonstrated a consistent cycling capacity of 496 and 280 mAh·g-1 at 0.03 and 5.0 A·g-1. Tao et al. produced mesoporous HC materials with N and P co-doping that were synthesized from soybean roots (NPDC)[46]. The material has abundant active sites, increased layer spacing, and a modified electronic structure. The pyrolysis of NPDC (NPDC-700) at 700 °C presented excellent rate capability (150 mAh·g-1 at 5.0 A·g-1) and exceptional cycle stability (197 mA·g-1 at 2.0 A·g-1 after 2,000 cycles). Yan et al. introduced an innovative synthesis technique for the fabrication of ultrahigh P-doped carbon (UPC) as an extraordinary-performance anode material for SIB[47]. By utilizing PCl3 and cyclohexane (C6H12) as the sources of P and C, in-situ P-doping was achieved during carbonization under N2 flow shown in Figure 2E, resulting in a phosphorus content up to 30 wt%. Moreover, the phosphorus incorporated in the carbon structure was mainly replaced by protrusions of P bonded to three C atoms [P-(C3)], which can remarkably enhance the interlamellar spacing and improve the sorptive ability of Na+. UPC-6 delivered a reversible capacity of 510.4 mAh·g-1 at 0.1 A·g-1 and an optimal rate capacity of 397.1 mAh·g-1 at 10 A·g-1 shown in Figure 2F.
Titanium-based oxides
Fan et al. used the N and S double doping method to achieve highly doped anatase-type titanium (NS-TiO2) dioxide hollow spheres illustrated in Figure 3A[48]. The N doping reduced the band gap width of TiO2 while the S doping promoted the spread of Na+ in TiO2. Therefore, TiO2 co-doped with N and S exhibited significant electron conductance and accelerated Na+ transfer kinetics. The tested material exhibited excellent rate performance and specific capacities of 249.3 and 156.4 mAh·g-1 at 0.1675 and 5.025 A·g-1, respectively. Additionally, it showed remarkable cycle stability with capacity retention of 90.5% at 3.35 A·g-1 for 2,400 cycles illustrated in Figure 3B.
Figure 3. (A) Synthesis routes for NS-TiO2; (B) Long-term cycle performance of TiO2 and NS-TiO2. Quoted with permission from Fan et al.[48]; (C) Synthesis routes for different TiO2 spheres, and their corresponding (D) Initial charge/discharge profiles and (E) Long-term cycling performance. Quoted with permission from Xu et al.[54]; (F) Synthetic routes for C-NTO and C-NTC microspheres; (G) Cycling stability and (H) Rate performance of C-NTO. Quoted with permission from Meng et al.[55]; TiO2-HS: TiO2 hollow spheres formed by smaller nanosheets; TiO2-YB: TiO2 yolk-like spheres with bigger nanosheets; TiO2-S: amorphous TiO2 solid spheres; TTIP: tetra-n-butyl titanate; NS-TiO2: N and S double doping method to achieve highly doped anatase-type titanium; NTO: Na2Ti3O7; NTC: titanate/titanium.
Studies have shown that incorporating porous carbon materials into TiO2 to construct TiO2@C composite material is conducive to enhancing the electronic/ionic conductivity of the composite material and is the most common and effective method to increase the electrochemical performance of titanium-based oxide anode materials. Luo et al. developed an anode material composed of TiO2 nanoparticles anchored on the shear carbon nanotube backbone (TiO2/SCNT), which demonstrated distinguished rate capability and cycling stability[49]. The TiO2/SCNT electrode showed a specific capacity of 267 and 136 mAh·g-1 at 1.0 and 10 C and preserved 87% of the initial capacity for 1,000 cycles. Wang et al. outlined a technique for preparing carbon fiber clothing-supported rutile TiO2 hollow cube array composites (H-TiO2@CFC)[50]. This compound material served as a self-sustaining and binder-free anode for Na+ storage, providing a reversible specific capacity of 287.3 and 103.3 mAh·g-1 at 0.1 and 50.0 C due to rapid ion dispersion and remarkable reactivity with Na+.
The kinetics of Na+ spread and its storage capacity within TiO2 can be improved through the combination of heteroatom doping and composite material constructed with carbon. Lv et al. synthesized N/F co-doped TiO2/carbon microspheres (NF-TiO2/C) through a simple hydrothermal approach and high-temperature doping reaction[51]. The co-doping of N and F increased the content of oxygen vacancy (VO), narrowed the band gap between TiO2 and C, and improved the conductivity of NF-TiO2/C. Additionally, NF-TiO2/C showed significant Na+ bond energy and a low Na+ diffusion barrier. Hence, NF-TiO2/C provided a marked contact density (1.51 g·cm-3), remarkable capacity (~242 mAh·g-1 at 1.0 C), excellent rate performance (190 and 125.9 mAh·g-1 at 10 and 100 C, respectively), high area capacity (~4.8 mAh·cm-2) and very lasting cycle performance (~80.2% at 10 C after 10,000 cycles). Wang et al. synthesized coadjacent micro-sheets composed of carbon nanotubes (CNTs) and S-doped TiO2 (CNT/S-TiO2) through ultrasonic technology followed by dead burn[52]. The use of single-wall CNTs (SWCNT) overcame the instability of electrode structure during the preparation of thick electrodes. CNT/S-TiO2 showed an outstanding rate performance (0.3 to 15 C). Furthermore, the material exhibited long-cycle stability of enduring 1,000 cycles under a load of 1.96 mg·cm-2.
Introducing different components into titanium-based materials to form heterogeneous structures can significantly enhance the conductivity of TiO2. Guan et al. devised heterogeneous structures consisting of two-dimensional (2D) nanosheets TiO2@TiOF2 by hydrothermal method[53]. Among them,
Conversion-type materials
Compositing TMSs and TMOs with carbon materials to construct composites can effectively prevent the aggregation of metal nanoparticles, improve the interconnection between the nanoparticles, and enhance the electrical conductivity of the electrode materials, which leads to improved specific capacity, rate capability, and cycling stability. The formation of bimetallic or trimetallic composites can take advantage of the synergistic effect of heterogeneous metals and achieve the enhancement of the electrochemical performance of electrode materials.
Zhao et al. prepared ultrafine TMO nanoparticles encapsulated in nitrogen-doped carbon nanofibers (uf-TMOs@N-CNFs), in which polyvinylpyrrolidone (PVP) was utilized as a highly effective dispersant for inhibiting metal agglomeration and controlling the size of TMOs[56]. The uf-Fe3O4@N-CNFs offered a capacity of 234.5 mAh·g-1 at 1.0 A·g-1 with a contribution of 78.69% from pseudo-capacitor control. They also exhibited remarkable rate performance and durable cycle stability while retaining a capacity of
Figure 4. (A) Schematic illustration of synthesis procedure of the CC-ZnS/CNT and its corresponding (B) HRTEM image, (C) Cycling performance at 0.1 A·g-1, (D) Rate capability compared with pure ZnS and ZnS/CNT, and (E) Long-term cycling performance at
Aminu Muhammad et al. successfully synthesized N-doped rGO (N-rGO) wrapped Ni3S2 nanocrystalline compound (Ni3S2-N-rGO-700 °C) through a simple phase conversion engineering process[59]. The strong coupling effect during annealing on the structural phase transition led to the formation of Ni3S2 nanocrystals and N-doped decreased graphene oxide nanocrystals, which significantly influenced the Na+ storage properties of Ni3S2-N-rGO-700 °C nanocarbons. The Ni3S2-N-rGO-700 °C compound demonstrated a notably reversible capacity of 377 mAh·g-1 at 2.0 A·g-1. Furthermore, the system demonstrated a remarkably high level of coulombic efficiency, exceeding 100% over more than 400 cycles. Wang et al. developed N-doped hollow carbon nanospheres with uniformly embedded MoSe2/Cr2Se3 heterojunction (MoSe2/
Chen et al. employed a one-step hydrothermal process followed by annealing to synthesize Fe7Ni3S11/CN[61]. The resulting spherical solid solution Fe7Ni3S11/CN had a hollow structure with ample active sites for Na+ storage. Additionally, the Fe7Ni3S11/CN nanoparticles were constrained by external CN, which promoted structural stability. The reversible specific capacity at 0.2 A·g-1 was 567 mAh·g-1. When exposed at 6.0 A·g-1, Fe7Ni3S11/CN maintained a capacity of 320 mAh·g-1 over 5,000 cycles.
Alloying-type materials
Nanocomposites can mitigate volume expansion/shrinking of antimony-based materials during the de/sodiation process and improve their performance as an anode for SIB. Wang et al. proposed an innovative N-doped 3D porous carbon and studied the design and fabrication of network-confined nano-FeSb alloy composites (3DFeSb@NC) with robust Fe–N–C bonds[62]. This distinctive structure fully mitigated the volume change of Sb throughout the cycle, shortened the ion/electron proliferation distance, and enhanced the electrical conductivity. The 3D FeSb@NC anode had an excellent charge/discharge capability of
Figure 5. (A) Fabrication process of the mesoporous Sb2O3@Sb nanocomposite and its corresponding (B) SEM image, (C) TEM image, (D) Cycling performance, (E) Rate performance. Quoted with permission from Ma et al.[63]; (F) The one-step dealloying process for NP-SnSb alloy and its corresponding (G) SEM image; (H) Cycling performance (as benchmarked with P-Sn); (I) Rate performance at various specific currents. Quoted with permission from Ma et al.[67]. SEM: Scanning electron microscope; TEM: transmission electron microscopy; NP-SnSb: bimetallic nanoporous SnSb; P-Sn: porous Sn.
Edison et al. incorporated Fe into SnSb by rapid solidification technology to produce a novel ternary nanocrystalline composite, which effectively reduced the stress resulting from the conversion reaction, thereby enhancing the cyclic stability[64]. The SIB anode material provided a specific capacity of
STRUCTURAL REGULATIONS
Structural regulation, such as nanostructure design, morphology control, and defect introduction can create more active sites, shorten the distance of electron/ion transfer, promote ion diffusion and electron transfer, and relieve the strain of the volume extension in electrochemical de/sodiation and thus improve the electrochemical performance. Here, it should be noted that defect introduction is different from doping. It refers to the formation of non-ideal structures or states within a material, including lattice defects, vacancies, and edge defects to provide more storage sites/adsorption sites or to change the atomic structure and charge distribution of the material to promote ion diffusion and charge transfer and therefore to enhance the sodium ion storage performance[41-43]. The electrochemical performance of various SIB anode materials after structural regulations is shown in Table 2.
The electrochemical performance of various SIB anode materials after structural regulations
Material | Mechanism | Regulation strategy | ICE (%) | Current density (mA·g-1) | Cycles | Capacity retention (mAh·g-1) | Ref. |
CPOP-1400 °C | Intercalation | Structural | 88.6 | 30 | 200 | 281.4 | [70] |
0.04 m-MnHC | Intercalation | Structural | 92.0 | 20 | 100 | 340 | [71] |
HC-21-1440 | Intercalation | Structural | 84 | 60 | 40 | 410 | [73] |
TiO2(A/B)-MS | Intercalation | Structural | 91 | 2,500 | 1,000 | 130 | [79] |
2D UNs⊂MT@C | Intercalation | Structural | 47.1 | 6,700 | 5,000 | 190 | [82] |
MoO2/β-MoO3-x | Conversion | Structural | 75.9 | 2,000 | 500 | 660 | [86] |
HCM-Fe3O4@void@N-C | Conversion | Structural | 62.2 | 160 | 800 | 522 | [90] |
SFS/C | Conversion | Structural | 82.4 | 5,000 | 1,000 | 534.8 | [91] |
Sb@3D Cu NWAs | Alloying | Structural | 64.3 | 330 | 200 | 561.1 | [94] |
Bulk Bi | Alloying | Structural | 97 | 7,700 | 3,500 | 379 | [97] |
Carbonaceous materials
The hydrothermal pretreatment process can facilitate the stabilization of the morphology and structure of the carbon material, thereby enhancing the performance of the battery. This is achieved by introducing a greater number of defects and nanopores, which provide additional active sites for the storage of sodium ions[69]. Lu et al. employed a low-cost, high-carbon yield bitumen as a precursor through a pre-oxidation strategy, enabling the bitumen to undergo effective structural transformations from ordered to disordered during the subsequent carbonation process[70]. The structural advantages of disordered carbon help to achieve excellent Na+ storage performance, resulting in a reversible capacity of 300.6 mAh·g-1 at 0.03 A·g-1. Zhao et al. synthesized an uncommon-performance HC anode with low defects and long-sequence graphite domains of 0.04 m-MnHC by the Mn2+ ion-assisted catalytic process, which catalyzed the growth of graphite sheets into long-sequence graphene layers[71]. The SIB anode material had a high ICE (92.05%) and capacity (336.8 mAh·g-1 at 0.02 A·g-1). Wang et al. prepared carbon materials from hazelnut shells by washing with hydrochloric acid to remove impurities and alter microstructure to prepare a HC SIB anode material with a reversible capacity of 342 mAh·g-1 at 0.02 A·g-1 and an ICE of 91%[72].
Meng et al. used the solvothermal method (ST) to form a precursor in liquid phenol-formaldehyde resin (PF) and ethanol (EtOH) with a volume ratio of 2:1, which was carbonized at 1,400 °C, and the synthesized sample was named HC-21-1400[73]. It showed a high reversible capacity of about 410 mAh·g-1 and a high ICE of 84% at 0.03 A·g-1. The capacity retention was 96% after 40 cycles. Kamiyama et al. synthesized the high-capacity HC material HC600-1500 (F50:50) by MgO template technology [Figure 6A][74]. After MgO was removed by pickling, nanoscale pores were formed and these pores were the main active sites for electrochemical sodium storage [Figure 6B and C]. Increasing the pretreatment temperature led to the growth of MgO template particles, resulting in a larger size and number of open micropores. As shown in Figure 6D, HC600-1500 (F50:50) obtained by pickling and post-heating at 1,500 °C showed a high reversible capacity of 478 mAh·g-1. It had good capacity retention and excellent ionization rate performance at
Figure 6. (A) Schematic illustration for the two mixing procedures for preparation of the mixtures of Mg Glu and Glc; TEM images and SAED patterns of (B) HC600-1500 (F0:100) and (C) HC600-1500 (F50:50); (D) Rate performance and (E) Cycle performance of HC600-1500 (F50:50). Quoted with permission from Kamiyama et al.[74]; (F) SEM image and (G) HRTEM and SAED images of CC-1600; (H) Cyclic capability of CCs. Quoted with permission from Li et al.[75]; Mg Glu: Magnesium gluconate; Glc: glucose; HC600-1500 (F0:100) and HC600-1500 (F50:50): HC by heating the Mg Glu/Glc ratio of 0:100 mol and 50:50 mol, respectively, with preheat-treatment temperature at 600 °C and post-heat-treatment temperatures at 1,500 °C; HC400-1500 (F50:50) and HC550-1500 (F50:50): HC by heating the Mg Glu/Glc ratio of 50:50 mol and preheat-treatment temperature at 400 °C and 550 °C, respectively, with post-heat-treatment temperatures at 1,500 °C; CC-Pre-800: carbonisation process at 800 °C for a period of 2 h, followed by a washing procedure with a dilute hydrochloric acid solution (30% concentration); CC-1200, CC-1400, and CC-1600: cork-derived HC pyrolyzed at 1,200, 1,400, and 1,600 °C, respectively, under argon flow for 2 h; TEM: transmission electron microscopy; SAED: selected area electron diffraction; SEM: scanning electron microscope; HRTEM: high-resolution transmission electron microscopy.
Titanium-based oxides
Anatase-type TiO2 is characterized by an open bilateral TiO6 octahedral crystal structure, which facilitates the insertion and removal of Na+. However, weak electronic conductivity and tardy ion diffusion severely limit the remarkable-rate performance of TiO2. Various strategies have been investigated to modify TiO2 to enhance Na storage capacity, for instance, the implementation of VO and the designing of nanoscale structures. Ma et al. prepared VOs-TiO2-x nanosheets with folds, which increased the conductivity and storage capacity of Na by adding Ti3+ and abundant VO[77]. At 20.0 A·g-1 the capacity can reach 91.0 mAh·g-1 with extremely long cycle stability. Han et al. proposed a two-solvent enhanced pressure strategy to prepare N-doped TiO2/C nanocomposites by using tetrabutyl-n-titanate tetramer as titanium dioxide and reducing its size with mutual solvent ethanol and doped dimethylformamide as pressors[78]. The material had an uncommon specific capacity of 370.4 mAh·g-1 at 0.05 A·g-1, a superior rate capability of 111.7 mAh·g-1 at
Figure 7. (A) Synthesis process of the TiO2(A/B)-MS material and its corresponding (B) HRTEM image; (C) Discharge capacities at various C rates; (D) Discharge capacities for long-term cycling. Quoted with permission from Hwang et al.[79]; (E) Schematic illustration of the synthesis process of titania-based nanoarchitectures and their corresponding SEM images and (F) Cycling performance at 0.2 C; (G) Long-term cycling performance of 2D UNs⊂TC at 50 C. Quoted with permission from Xia et al.[82]. aTiO2/HDA: The titania oligomers by hydrogen-bonding to condense into a compact amorphous inorganic-organic shell; aTiO2/HDA@mcTiO2: a hybrid shell consisting of an amorphous inorganic-organic inner shell tightly confined by a mesoporous crystalline outer shell; mcTiO2: the HDA was partly removed, and a highly crystalline mesoporous shell; 2D UNs⊂TC: hierarchical nano configurations with the stepwise varying morphologies of 2D UNs vertically encapsulated in TiO2/carbon hollow nanospheres; MT@C: mesoporous hollow nanospheres consisting of graphitic carbon-coated TiO2 nanocrystals; HRTEM: high-resolution transmission electron microscopy; SEM: scanning electron microscope.
Lan et al. proposed a TiO2 mesoporous structure with a precisely controlled mesoporous framework[81]. The meticulously crafted mesoscopic TiO2 model attained a maximum specific capacity of 240 mAh·g-1. Under the high mass loading of 9.47 mg·cm-2, the area capacity of 2.1 mAh·cm-2 was further achieved. Xia et al. constructed 2D ultrathin nanosheets (UNs) vertically aligned inside of graphitic carbon-coated mesoporous TiO2 hollow nanospheres (2D UNs⊂MT@C) shown in Figure 7E[82]. It consisted of regularly alternating monomolecular layers of TiO2 and carbon, which facilitated rapid electron and Na+ transportation and abundant 2D heterogeneous interface active sites. The structure demonstrated excellent mechanical stability and structural integrity, ensuring more than 500 cycles at 0.2 C and a capacity decay rate of 0.04% of the battery shown in Figure 7F. At 50 C, the long-term cycle is up to 20,000 times shown in Figure 7G. Liu et al. used strong 1D TiO2 nanowires as a substrate to link these nanosheets together and constructed a bundle layered structure [TiO2(B)-BH] with a capacity of 131 mAh·g-1 at 0.5 C and a distinguished rate of 10 C[83]. A reversible capacity of up to 500 cycles can still be maintained at 85 mAh·g-1.
Conversion-type materials
Yang et al. synthesized nickel cobaltite@nitrogen-doped holey graphene (NCO@N-HG) and Fe3O4@N-HG composites by loading nickel-cobaltate and magnetite nanoparticles in a 3D nitrogenous porous graphene framework, respectively[84]. The thin graphene sheet buffered volume changes and the unique large pore structure combined with the existence of nanoholes in the graphene sheet facilitated ion transport during the processes of charge/discharge, contributing to the observed superb specific capacity and good cycling performance of 510 mAh·g-1 over 100 cycles at 0.1 A·g-1. The ball milling technology[85] was employed to adjust the lattice strain of NiS nanocomposites. The restructured anode had a superb capacity, great rate performance (495.7 and 383.2 mAh·g-1 at 0.2 and 15.0 A·g-1), and cycle performance (16,000 cycles at
Zhang et al. constructed MO@NCF (MO = Co3O4, Mn3O4, Fe3O4, NCF: nitrogen-enriched 3D carbon nanofiber networks) by coordinating nanofibrous carboxymethyl chitosan (CMCh) hydrogel with metal ions to create a steady metal-polysaccharide skeleton, then a multistage porous structure was formed through the pyrolysis process[88]. The Co3O4@NCF exhibited an impressive Na+ storage capacity of
Cao et al. successfully fabricated fine hierarchical heterostructures composed of antimonous sulfide Sb2S3@FeS2 (SFS) hollow nanorods embedded in an N-doped carbon matrix shown in Figure 8A and B[91]. The well-designed architecture facilitated the rapid diffusion of Na ions and enhanced charge transfer at heterogeneous interfaces. Simultaneously, this structure greatly accelerated electron/ion transport and inhibited volume expansion over a long cycle. Thus, the composite demonstrated outstanding rate performance of 537.9 mAh·g-1 at 10.0 A·g-1 and exceptional cyclic stability of 85.7% capacity retention after 1,000 cycles at 5.0 A·g-1 shown in Figure 8C. Liu et al. investigated a general strategy for successfully preparing a variety of high entropy oxide-polyoxometalate (HEO-POM) self-assembled nanowires using POM clusters shown in Figure 8D, where the types of metal oxides and POM clusters can be flexibly adjusted[92]. A series of experiments and molecular dynamics simulations showed that the formation of HEO-POM sub-1 nm nanowires (SNWs) followed the oriented attachment mechanism; namely, the POM clusters acted as linkers for multiple metal oxides under the action of van der Waals interactions. CoZnCuNiFeZrCeOx-phosphomolybdic acid (PMA) SNWs can maintain a high capacity retention of about 92% even after 5,000 cycles at 10 C shown in Figure 8E.
Figure 8. (A) Schematic illustration of the fabrication process of tSFS/C composite and its corresponding (B) TEM image; (C) Cycle performance of SFS/C, SFS, and Sb2S3 at 5 A g-1. Quoted with permission from Cao et al.[91]; (D) The snapshot of a nanowire composed of CoZnCuNiFeZrCeOx-PMA building blocks with HAADF-STEM images and AC-TEM images; (E) Cycling stability of CoZnCuNiFeZrCeOx-PMA SNWs. Quoted with permission from Liu et al.[92]. SFS/C: Sb2S3@FeS2/N-doped graphene; TEM: transmission electron microscopy; PMA: phosphomolybdic acid; HAADF: high-angle annular dark field; STEM: scanning transmission electron microscopy; AC-TEM: aberration-corrected transmission electron microscopy; SNWs: sub-1 nm nanowires.
Alloying-type materials
Yang et al. designed an optimized Bi@Void@C nanosphere (Bi@Void@C-2) with a yolk-shell structure, which exhibited a conspicuous cycle life of 198 mAh·g-1 after 10,000 cycles at 20.0 A·g-1[93]. Theoretical calculations and experimental studies showed that the external layer of the thin carbon shell was of significant importance in improving electronic conductivity and preventing the aggregation of Bi nanoparticles. Fan et al. designed a distinctive 3D collector assembled from an array of 1D nanowires (1D NWAs) anchored to 3D porous copper foam for constructing core-shell Cu@Sb nanowires[94]. The resulting 3D hierarchical anode with interconnected 3D micron-sized holes and abundant interwire spaces effectively relieved the structural stress during cycling, which ensured the structural integrity and helped to achieve a uniform ion/electron distribution over the entire surface as shown in Figure 9A. The electrode exhibited a high specific capacity of 605.3 mAh·g-1 at 0.33 A·g-1 [Figure 9B], and a supernormal capacity retention of 84.8% even at a prominent specific current of 3.3 A·g-1 [Figure 9C].
Figure 9. (A) SEM images of Sb@3D Cu NWAs from (a and b) a top view, (c) a side view, and (d and e) an enlarged top view. Energy dispersive X-ray spectroscopy maps for elements (f) Cu and (g) Sb; (B) Cyclability curves; (C) Rate performance. Quoted with permission from Fan et al.[94]; (D) Structural evolution of bulk Bi flakes. Secondary-electron images recorded at low magnification; (E) Changes in specific capacity of Na-Bi half-cells measured at various C-rates using an electrolyte containing DME; (F) Changes in the specific capacity of the Na-Bi half-cell with DME and EC/DEC solvent measured at 20.0 C as a function of the battery cycle. Quoted with permission from Kim et al.[97]. SEM: Scanning electron microscope; Sb@3D Cu NWAs: a thin film of Sb is electrochemically deposited on 3D Cu NWAs; DME: dimethoxy-ethane; EC: ethylene carbonate; DEC: diethyl carbonate.
Carbon fiber has excellent electrical conductivity, a significant specific surface area, and superb electrochemical stability. Through electrostatic spinning and reduction roasting, an independent carbon fiber film was obtained. Owing to the superb specific surface area and porosity, the one-dimensional structure provided more active centers and greatly alleviated the volume expansion. Dang et al. developed a distinctive pod-like independent film[95]. This film incorporated SbSn particles into an integrated carbon matrix. In addition, the following materials are included: hollow carbon spheres and N-doped carbon fibers (B-SbSn/NCFs), which not only improved the electrical conductivity and flexibility but also provided sufficient space to accommodate volume changes. Moreover, the B-SbSn/NCFs self-supporting film can serve as a direct anode without the need for polymer binders and conductors, which improves the energy density and reaction kinetics. The membrane electrode demonstrated a specific capacity of 486.9 mAh·g-1 with a coulombic efficiency approaching 100% after 400 cycles at 0.1 A·g-1.
Song et al. prepared dispersed in situ 3D cross-linked carbon nanofiber SnSb@CNF[96]. The 3D cross-linked nanostructures ensured more Na storage active sites, stable structure, and increased electrical conductivity. Thus, the SnSb@CNF/CNT anode exhibited excellent cycle stability (161 mAh·g-1 in 1,000 cycles at
INTERFACIAL REGULATIONS
In a typical SIB system, ICE is utilized to evaluate the reversible migration of Na+ during the initial charge/discharge process, manifested as the reversible capacity in the first cycle, which is one of the key parameters of SIBs. For a half-cell, the sodium metal can supply excessive Na+ for sodiation/desodiation, making ICE not crucial. However, in a full-cell, insufficient ICE may result in limited consumption of Na at the cathode, resulting in a decrease in energy density. Therefore, improving the ICE of the anode contributes to promoting the advancement and practical implementation of SIBs significantly. The low ICE is a common problem that exists in all types of SIB anode materials, which is believed to be owe to the irreversible capture of Na+ and the formation of SEI layers. The structure and morphology of anode materials, such as defects, pores, and crystalline phases, can significantly influence the ICE. Strategies such as modification of the electrode material (doping, surface coating, microstructure, and porosity adjustment to reduce the side reactions of the electrode with the electrolyte) and optimization of the electrolyte composition (salts, solvents, and additives) are beneficial for the construction of an improved SEI and thus improve the ICE[29,31,34,35]. The electrochemical performance of various SIB anode materials after interfacial regulations is shown in Table 3.
The electrochemical performance of various SIB anode materials after interfacial regulation
Material | Mechanism | Regulation strategy | ICE (%) | Current density (mA·g-1) | Cycles | Capacity retention (mAh·g-1) | Ref. |
pHC | Intercalation | Interfacial | 99.2 | 50 | 300 | 108.9 | [99] |
H-NTO | Intercalation | Interfacial | 49 | 100 | 3,000 | 120 | [104] |
Li-TiO2NTS | Intercalation | Interfacial | 87 | 1,000 | 250 | 132 | [106] |
Sn-MnO@C | Conversion | Interfacial | 91.5 | 1,000 | 500 | 214 | [107] |
Sn@NC | Alloying | Interfacial | 78.4 | 1,000 | 400 | 474 | [108] |
Carbonaceous materials
HC has a substantial specific surface area and numerous defect sites and more sodium ions need to be sacrificed to form SEI films, which ultimately generates an increase in irreversible specific capacity and a decrease in coulombic efficiency. The ICE of graphite anode is more than 90% while that of HC anode is usually only 60% to 80%.
Fang et al. employed sodium diphenyl ketone (Na-DK) as a reducing agent for the bifunctional preparation of HC[99]. The chemically synthesized sodium facilitated the formation of a robust sodium fluoride (NaF)-rich SEI layer in the electrolyte by pre-doping HC. Figure 10A analyzes the optimal structure of adsorbed and non-adsorbed Na+, revealing the interaction of Na+, dibenzoyl (DK), and HC. As illustrated in Figure 10B, the presodiated HC (pHC) anode exhibited a high reversible capacity of 251.5 mAh·g-1 at
Figure 10. (A) Optimized structures of Na+ adsorbed on graphene layer of 60 carbon atoms containing five/seven-membered rings; (B) Rate capability of HC and pHC; (C) Cycle performance of full cells at 0.5 A·g-1 of pHC//NVP and HC//NVP. Quoted with permission from Fang et al.[99]; (D) Schematic illustration of the evolution of SEI films in the DME-based and DME-0.5%VC electrolytes upon prolonged cycling on HCs; TEM images of HC anodes after 2,000 cycles in the (E) DME-based and (F) DME-0.5%VC electrolytes; (G) Long-term cycling performance. Quoted with permission from Bai et al.[100]. DME-VC-SEI: SEI films in DME and vinylene carbonate electrolytes; HC: hard carbon; pHC: presodiated HC; NVP: Na3V2(PO4)3; SEI: solid electrolyte interphase; DME: dimethoxy-ethane; TEM: transmission electron microscopy.
Jin et al. presented an advanced electrolyte containing sodium difluorosulfonyl imide (NaFSI)-triethyl phosphate (TEP), which exhibited great stability with high voltage cathodes and was able to improve the cycling of Na+ batteries[101]. The Na||NaNi0.68Mn0.22Co0.10O2 (NaNMC) battery demonstrated a capacity retention of 89% after 500 cycles with a cut-off voltage of 4.2V (vs. Na+/Na). Jin et al. introduced NaFSI-TEP/1,1,2,2-tetrafluoroethyl-2,2,3,3-tetrafluoropropyl ether (TTE) (molar ratio 1:1.5:2), a non-combustible electrolyte for SIBs, which stabilized the interface at the layered O3-NaCu1/9Ni2/9Fe1/3Mn1/3O2 (Na-CNFM) cathode and HC anode simultaneously for consistent cyclic performance[102]. The capacity retention rate of Na||HC battery after 500 cycles was 94.8% (247.9 mAh·g-1). The high-quality interface established on both the cathode and anode ensured HC’s exceptional cycle stability.
Titanium-based oxides
Li et al. combined 16 nm anatase TiO2 with diglyme-based electrolyte and the reversible capacity reached 257.9 and over 100 mAh·g-1 at 0.1 and 2.0 A·g-1[103]. Both of them were significantly better than the ethylene carbonate (EC)/diethyl carbonate (DEC)-based electrolytes. It had been shown that the ether-based electrolyte promoted the Na+-induced TiO2 structure transformation, and the charge transfer kinetics at the SEI/electrode interface played a crucial role in rate cycling performance. Meng et al. reported hollow NTO microspheres (H-NTO) with oxygen defects prepared from 2D ultra-thin nanosheets[104]. These microspheres exhibited a unique chemical bonding NTO/C(N) interface shown in Figure 11A. When the temperature reached 60 °C, the specific capacity of the H-NTO electrode was 59 mAh·g-1 at 20 A·g-1 demonstrated in Figure 11B. By optimizing the electrolyte, the H-NTO electrode can be stably cycled at
Figure 11. (A) Synthetic route for H-NTO microspheres and its corresponding (B) Rate performance at different temperatures; (C) Cycle performance. Quoted with permission from Meng et al.[104]; (D) Synthesis of Li+ pre-inserted SEI layer; (E) Rate performance; (F) Cycling performance at 1.0 A·g-1. Quoted with permission from Cha et al.[106]. A-TNT: Anatase TiO2 NTs (A-TNT); Li-TiO2 NTs: Li+ inserted anatase TiO2 NTs; Li-TiO2 NTs-De: Li+ inserted/de-inserted anatase TiO2 NTs; H-NTO: hollow Na2Ti3O7; SEI: solid electrolyte interphase.
Xu et al. showed that anatase TiO2 underwent amorphous crystallization during the first alkalization process and in the subsequent cycles[105]. The amorphous phase showed pseudo-capacitor sodium storage behavior. For the first time, it was found that the ether-based electrolyte was able to form a thin (≈ 2.5 nm) and stable SEI layer while the conventional carbonate-based electrolyte could form a thick (≈ 10 nm) and growing SEI layer. The elevated minimum molecular orbital energy of the ether solvent/ionic complexes was the underlying cause of this discrepancy. In the ether-based electrolyte, after 500 cycles, a specific capacity of 192 mAh·g-1 can be achieved at 0.1 A·g-1, which significantly exceeds the capacity of the carbonate electrolyte. Cha et al. obtained significantly enhanced SEI layers on TiO2 nanotube (TiO2 NTS) arrays, which improved the performance of sodium battery systems[106]. Pre-insertion of Li+ into TiO2NTS can make SEI meet the conditions of Na+ replacement shown in Figure 11D. SIBs constructed using pre-embedded lithium nanotubes had superb rate capability (132 mAh·g-1 at 1.0 A·g-1) shown in Figure 11E and superb Na+ cycle stability (capacity retention of 99.9% after 250 cycles at 1.0 A·g-1) shown in Figure 11F.
Conversion-type and alloying-type materials
Li et al. developed a new novel type of alloying/conversion-based anode in which the metal oxide (e.g., MnO) micro dumbbell skeleton was designed to stabilize large capacity alloyed (e.g., Sn) nanoparticles[107]. In combination with electrolyte engineering methods, the Sn-MnO@C anode exhibited outstanding Na+ storage performance, including an excellent specific capacity of 370 mAh·g-1, a good rate capacity of more than 10 A·g-1, and a favorable cycling life of more than 500 cycles. Yang et al. performed surface engineering on tin nanorods by using N-doped carbon layers (Sn@NC) and interface engineering strategy shown in Figure 12A[108]. The uniform surface modification effectively enhanced electron and Na+ transport kinetics and interacted with the ether-based electrolyte to form a strong organic-inorganic SEI shown in Figure 12B. Moreover, Sn@NC anodes provided ultra-long cycle stability of more than 10,000 cycles shown in Figure 12C.
Figure 12. (A) Schematic of synthesis of Sn@NC composite and its (B) TEM image; (C) Long cycling performance of composite anodes at 5.0 A·g-1 in the DEGDME-based electrolyte. Quoted with permission from Yang et al.[108]; (D) Composition/nanostructure of SEIs probed by cryo-TEM and XPS. (a-f) HAADF STEM and TEM images of SEIs in (a, c, and e) NaBF4/EC/DMC and (b, d and f)
Chu et al. employed an ultra-low concentration electrolyte strategy that included a significantly high amount of sodium salts of tetra phenyl-borate ion (BPH4-) and a DME solvent to stabilize micron tin and bismuth anodes[109]. In the 0.1 M NaBPh4-DME electrolyte, sodium had a faster migration rate attributed to the significant steric hindrance of BPH4- anion, which made it difficult to approach the electrode/electrolyte interface and thus the side reactions at the electrode/electrolyte interface were reduced. With electrolyte optimization, the specific capacity of 600 mAh·g-1 on the Sn anode can be reached at 0.5 A·g-1. Huang et al. explored the nanostructure and nanomechanical SEI derived from carbonate-and ether-based materials on micron-sized tin particles[110]. The structure/composition and properties of SEI derived from EC/DMC and diethylene glycol dimethyl ether were elucidated. The SEI derived from diethylene glycol dimethyl ether exhibited high elasticity and ionic conductivity, which can be attributed to the unique structure of the amorphous particles dispersed in polymer-like substances shown in Figure 12D. Compared to the NaBF4/(EC/DMC) electrolytic liquid phase, the diethylene glycol electrolyte provided significantly improved coulomb efficiency and cycle stability shown in Figure 12E-G.
Wu et al. demonstrated the rapid production of ultrafine Bi nanoparticles embedded in a 3D carbon framework (Bi@3D-CF) by a Joule heating method with a synthesis time of just 30 s[111]. The Bi@3D-CF exhibited excellent Na storage performance in 1,2-DME based electrolyte with a capacity of 349 mAh·g-1 after 12,000 cycles at 5.0 A·g-1, and 311 mAh·g-1 at 100 A·g-1. The outstanding performance was attributed to the formation of unique 3D porous structures during cycling and the gradient organic/inorganic SEI.
CONCLUSION AND OUTLOOKS
In this review, we have summarized various synthesis and regulation strategies, such as composition regulation (heteroatom doping, composite construction, and formation of heterostructure), structural regulation (nanostructure design, morphology control, and defect introduction), and interfacial regulation (the regulation of electrode materials and electrolyte salts, solvents, and additives to form a stable SEI) to improve the electrochemical performance of intercalation-type, conversion-type, and alloying-type SIB anode materials with selected examples. The underlying mechanism is to provide more storage/active cites, reduce the strain stress, improve the electronic/ionic conductivity of the electrode materials, and suppress/reduce the electrolyte decomposition by composition and structure modulation to improve their specific capacity, cycling stability, and rate capability. Here are the conclusions and outlooks:
(1) Heteroatom doping, composite construction, and defect introduction are the most critical strategies for intercalation-type SIB anode materials to improve the specific capacity and rate capability. Formation of heterostructure, nanostructure design, and morphology control are the most vital strategies for conversion-type and alloying-type SIB anode materials to improve cycling stability and rate capability. Interfacial regulation strategies are highly desired for each type of SIB anode material because low ICE is the common challenge that all three types of anode materials have to face. By comparing the ideal electrochemical performance of SIB anode materials afore-mentioned in the introduction with the obtained electrochemical performance of various anode materials listed in Tables 1-3, it is evident that despite the implementation of diverse synthesis and regulation strategies, the above-mentioned various SIB anode materials only partially meet the application requirements. Among them, HC is the most appealing anode material for the commercialization of SIBs as it shows the best sodium ion storage performance and is widely available, affordable, and environmentally friendly. Especially, the specific capacity of HC has reached 300 mAh·g-1 and the electrical conductivity can also be improved through N, O, S, and other heteroatom doping. However, the overall performance is still not satisfactory; the specific capacity and rate capability need to be further improved, especially the long-term cycle stability and the low ICE needs to be improved as well. Titanium-based oxides have excellent cycling stability while their specific capacities and ICE still need to be further improved. The conversion type material has a theoretical specific capacity ranging from 800 to
(2) Beyond the mainstream intercalation, conversion, and alloying-type anode materials, metal-organic frameworks (MOFs)[112-115], covalent organic frameworks (COFs)[116,117], and organic materials[118-120] have become one of the important classes of materials for SIB anodes in recent years. MOFs are generated by the self-assembly of metal nodes and organic ligands via coordination bonds. They possess high specific surface areas and tunable pore structures, which enable MOFs to provide abundant active sites and favorable ion diffusion channels, thus improving the specific capacity and rate capability used as SIB anodes. COFs are crystalline porous materials formed by connecting organic units through covalent bonds. They exhibit resource sustainability, structural designability, and excellent chemical stability, which enable COFs to provide designable and stable structures, thus improving the selectivity and cycling stability used as SIB anodes. Similar to COFs, resource sustainability and structural designability allow organic materials to optimize their sodium-ion storage performance accordingly and flexibly. Thus, the sustainability, versatility, and customizability of MOFs, COFs, and organics allow them to show great prospects for the development of next-generation high-performance SIB anode materials. With ongoing advancements in materials science and electrochemical engineering, the performance of these materials is expected to be further enhanced, promoting the SIB technique toward commercialization.
(3) In this review, we mainly discussed the improvement of the sodium ion storage performance of anodes from the materials design aspect. Nevertheless, for plenty of sodiation/desodiation processes, the related structure-performance relationships and the underlying reaction mechanisms are still unclear. This can be effectively solved by the application of in situ/operando characterization techniques such as Raman/infrared (IR) spectroscopy, X-ray diffraction (XRD), and transmission electron microscopy (TEM) to monitor the reaction processes. The obtained information can elucidate the structure-performance relationships and the underlying reactions, guiding the rational design of SIB anode materials with superior performance.
DECLARATIONS
Authors’ contributions
Wrote the draft manuscript: Lin, Y. T.; Lin, B. L.; Niu, B. T.; Lin, X. M.
Revised the manuscript: Chen, X. P.; Guo, H. X.; Lin, X. M.
Guided this work: Lin, X. M.
Availability of data and materials
Not applicable.
Financial support and sponsorship
We are grateful to the National Natural Science Foundation of China (22272069) and the Natural Science Foundation of Fujian Province (2021J01988 and 2022J05173).
Conflicts of interest
All authors declared that there are no conflicts of interest.
Ethical approval and consent to participate
Not applicable.
Consent for publication
Not applicable.
Copyright
© The Author(s) 2025.
REFERENCES
1. Li, M.; Lu, J.; Chen, Z.; Amine, K. 30 years of lithium-ion batteries. Adv. Mater. 2018, 30, e1800561.
2. Weiss, M.; Ruess, R.; Kasnatscheew, J.; et al. Fast charging of lithium-ion batteries: a review of materials aspects. Adv. Energy. Mater. 2021, 11, 2101126.
3. Xu, J.; Cai, X.; Cai, S.; et al. High-energy lithium-ion batteries: recent progress and a promising future in applications. Energy. Environ. Maters. 2023, 6, e12450.
4. Nayak, P. K.; Yang, L.; Brehm, W.; Adelhelm, P. From lithium-ion to sodium-ion batteries: advantages, challenges, and surprises. Angew. Chem. Int. Ed. Engl. 2018, 57, 102-20.
5. Chayambuka, K.; Mulder, G.; Danilov, D. L.; Notten, P. H. L. From Li-ion batteries toward Na-ion chemistries: challenges and opportunities. Adv. Energy. Mater. 2020, 10, 2001310.
6. Huang, Y.; Zhao, L.; Li, L.; Xie, M.; Wu, F.; Chen, R. Electrolytes and electrolyte/electrode interfaces in sodium-ion batteries: from scientific research to practical application. Adv. Mater. 2019, 31, e1808393.
7. Qiao, S.; Zhou, Q.; Ma, M.; Liu, H. K.; Dou, S. X.; Chong, S. Advanced anode materials for rechargeable sodium-ion batteries. ACS. Nano. 2023, 17, 11220-52.
8. Chen, J.; Adit, G.; Li, L.; Zhang, Y.; Chua, D. H. C.; Lee, P. S. Optimization strategies toward functional sodium-ion batteries. Energy. Environ. Mater. 2023, 6, e12633.
9. Chen, X.; Yin, X.; Aslam, J.; Sun, W.; Wang, Y. Recent progress and design principles for rechargeable lithium organic batteries. Electrochem. Energy. Rev. 2022, 5, 135.
10. Wu, X.; Feng, X.; Yuan, J.; et al. Thiophene functionalized porphyrin complexes as novel bipolar organic cathodes with high energy density and long cycle life. Energy. Storage. Mater. 2022, 46, 252-8.
11. Yang, G.; Zhu, Y.; Zhao, Q.; et al. Advanced organic electrode materials for aqueous rechargeable batteries. Sci. China. Chem. 2024, 67, 137-64.
12. Zhang, X.; Xing, P.; Madanu, T. L.; Li, J.; Shu, J.; Su, B. L. Aqueous batteries: from laboratory to market. Natl. Sci. Rev. 2023, 10, nwad235.
13. Zhang, H.; Gao, Y.; Liu, X.; et al. Long-cycle-life cathode materials for sodium-ion batteries toward large-scale energy storage systems. Adv. Energy. Mater. 2023, 13, 2300149.
14. Yao, H.; Zheng, L.; Xin, S.; Guo, Y. Air-stability of sodium-based layered-oxide cathode materials. Sci. China. Chem. 2022, 65, 1076-87.
15. Ma, Y.; Hu, Y.; Pramudya, Y.; et al. Resolving the role of configurational entropy in improving cycling performance of multicomponent hexacyanoferrate cathodes for sodium-ion batteries. Adv. Funct. Mater. 2022, 32, 2202372.
16. Hao, Z.; Shi, X.; Yang, Z.; et al. The distance between phosphate-based polyanionic compounds and their practical application for sodium-ion batteries. Adv. Mater. 2024, 36, e2305135.
17. Pramanik, A.; Manche, A. G.; Sougrati, M. T.; Chadwick, A. V.; Lightfoot, P.; Armstrong, A. R. K2Fe(C2O4)2: an oxalate cathode for Li/Na-ion batteries exhibiting a combination of multielectron cation and anion redox. Chem. Mater. 2023, 35, 2600-11.
18. Lin, X.; Yang, X.; Chen, H.; et al. In situ characterizations of advanced electrode materials for sodium-ion batteries toward high electrochemical performances. J. Energy. Chem. 2023, 76, 146-64.
19. Chen, X.; Liu, C.; Fang, Y.; et al. Understanding of the sodium storage mechanism in hard carbon anodes. Carbon. Energy. 2022, 4, 1133-50.
20. Zhao, L.; Hu, Z.; Lai, W.; et al. Hard carbon anodes: fundamental understanding and commercial perspectives for Na-ion batteries beyond Li-ion and K-ion counterparts. Adv. Energy. Mater. 2021, 11, 2002704.
21. Li, Y.; Lu, Y.; Adelhelm, P.; Titirici, M. M.; Hu, Y. S. Intercalation chemistry of graphite: alkali metal ions and beyond. Chem. Soc. Rev. 2019, 48, 4655-87.
22. Peng, P.; Wu, Y.; Li, X.; et al. Toward superior lithium/sodium storage performance: design and construction of novel TiO2-based anode materials. Rare. Met. 2021, 40, 3049-75.
23. Dong, J.; Jiang, Y.; Wang, R.; Wei, Q.; An, Q.; Zhang, X. Review and prospects on the low-voltage Na2Ti3O7 anode materials for sodium-ion batteries. J. Energy. Chem. 2024, 88, 446-60.
24. Yang, Z.; Zhang, J.; Kintner-Meyer, M. C.; et al. Electrochemical energy storage for green grid. Chem. Rev. 2011, 111, 3577-613.
25. Lin, X.; Chen, J.; Fan, J.; et al. Synthesis and operando sodiation mechanistic study of nitrogen-doped porous carbon coated bimetallic sulfide hollow nanocubes as advanced sodium ion battery anode. Adv. Energy. Mater. 2019, 9, 1902312.
26. Konkena, B.; Kalapu, C.; Kaur, H.; et al. Cobalt oxide 2D nanosheets formed at a polarized liquid|liquid interface toward high-performance Li-ion and Na-ion battery anodes. ACS. Appl. Mater. Interfaces. 2023, 15, 58320-32.
27. Yang, X. T.; Huang, T. Y.; Wang, Y. H.; et al. Understanding the origin of the improved sodium ion storage performance of the transition metal oxide@carbon nanocomposite anodes. J. Chem. Phys. 2023, 158, 174708.
28. Wu, Y.; Yao, Y.; Wang, L.; Yu, Y. Recent progress on modification strategies of alloy-based anode materials for alkali-ion batteries. Chem. Res. Chin. Univ. 2021, 37, 200-9.
29. Wu, X.; Lan, X.; Hu, R.; Yao, Y.; Yu, Y.; Zhu, M. Tin-based anode materials for stable sodium storage: progress and perspective. Adv. Mater. 2022, 34, e2106895.
30. Tan, M.; Han, S.; Li, Z.; Cui, H.; Lei, D.; Wang, C. Compact Sn/C composite realizes long-life sodium-ion batteries. Nano. Res. 2023, 16, 3804-13.
31. Huang, H.; Xu, R.; Feng, Y.; et al. Sodium/potassium-ion batteries: boosting the rate capability and cycle life by combining morphology, defect and structure engineering. Adv. Mater. 2020, 32, e1904320.
32. Guo, S.; Yi, J.; Sun, Y.; Zhou, H. Recent advances in titanium-based electrode materials for stationary sodium-ion batteries. Energy. Environ. Sci. 2016, 9, 2978-3006.
33. Lao, M.; Zhang, Y.; Luo, W.; Yan, Q.; Sun, W.; Dou, S. X. Alloy-based anode materials toward advanced sodium-ion batteries. Adv. Mater. 2017, 29, 1700622.
34. Zhang, M.; Li, Y.; Wu, F.; Bai, Y.; Wu, C. Boost sodium-ion batteries to commercialization: strategies to enhance initial coulombic efficiency of hard carbon anode. Nano. Energy. 2021, 82, 105738.
35. He, H.; Sun, D.; Tang, Y.; Wang, H.; Shao, M. Understanding and improving the initial Coulombic efficiency of high-capacity anode materials for practical sodium ion batteries. Energy. Storage. Mater. 2019, 23, 233-51.
36. Li, Y.; Chen, M.; Liu, B.; Zhang, Y.; Liang, X.; Xia, X. Heteroatom doping: an effective way to boost sodium ion storage. Adv. Energy. Mater. 2020, 10, 2000927.
37. Zhao, R.; Sun, N.; Xu, B. Recent advances in heterostructured carbon materials as anodes for sodium-ion batteries. Small. Struct. 2021, 2, 2100132.
38. Li, Y.; Wu, F.; Li, Y.; et al. Ether-based electrolytes for sodium ion batteries. Chem. Soc. Rev. 2022, 51, 4484-536.
39. Tian, Z.; Zou, Y.; Liu, G.; et al. Electrolyte solvation structure design for sodium ion batteries. Adv. Sci. 2022, 9, e2201207.
40. Cheng, H.; Sun, Q.; Li, L.; et al. Emerging era of electrolyte solvation structure and interfacial model in batteries. ACS. Energy. Lett. 2022, 7, 490-513.
41. Pei, Z.; Meng, Q.; Wei, L.; Fan, J.; Chen, Y.; Zhi, C. Toward efficient and high rate sodium-ion storage: a new insight from dopant-defect interplay in textured carbon anode materials. Energy. Storage. Mater. 2020, 28, 55-63.
42. Jin, Q.; Wang, K.; Feng, P.; Zhang, Z.; Cheng, S.; Jiang, K. Surface-dominated storage of heteroatoms-doping hard carbon for sodium-ion batteries. Energy. Storage. Mater. 2020, 27, 43-50.
43. Xie, F.; Niu, Y.; Zhang, Q.; et al. Screening heteroatom configurations for reversible sloping capacity promises high-power Na-ion batteries. Angew. Chem. Int. Ed. Engl. 2022, 61, e202116394.
44. Wu, S.; Peng, H.; Xu, J.; et al. Nitrogen/phosphorus co-doped ultramicropores hard carbon spheres for rapid sodium storage. Carbon 2024, 218, 118756.
45. Mehmood, A.; Ali, G.; Koyutürk, B.; Pampel, J.; Chung, K. Y.; Fellinger, T. Nanoporous nitrogen doped carbons with enhanced capacity for sodium ion battery anodes. Energy. Storage. Mater. 2020, 28, 101-11.
46. Tao, S.; Xu, W.; Zheng, J.; et al. Soybean roots-derived N, P Co-doped mesoporous hard carbon for boosting sodium and potassium-ion batteries. Carbon 2021, 178, 233-42.
47. Yan, J.; Li, H.; Wang, K.; et al. Ultrahigh phosphorus doping of carbon for high-rate sodium ion batteries anode. Adv. Energy. Mater. 2021, 11, 2003911.
48. Fan, M.; Lin, Z.; Zhang, P.; et al. Synergistic effect of nitrogen and sulfur dual-doping endows TiO2 with exceptional sodium storage performance. Adv. Energy. Mater. 2021, 11, 2003037.
49. Luo, S.; Yuan, T.; Soule, L.; et al. Enhanced ionic/electronic transport in nano-TiO2/sheared CNT composite electrode for Na+ insertion-based hybrid ion-capacitors. Adv. Funct. Mater. 2020, 30, 1908309.
50. Wang, C.; Zhang, J.; Wang, X.; Lin, C.; Zhao, X. S. Hollow rutile cuboid arrays grown on carbon fiber cloth as a flexible electrode for sodium-ion batteries. Adv. Funct. Mater. 2020, 30, 2002629.
51. Lv, D.; Wang, D.; Wang, N.; et al. Nitrogen and fluorine co-doped TiO2/carbon microspheres for advanced anodes in sodium-ion batteries: high volumetric capacity, superior power density and large areal capacity. J. Energy. Chem. 2022, 68, 104-12.
52. Wang, C.; Yao, Q.; Wang, M.; et al. Highly conductive hierarchical TiO2 micro-sheet enables thick electrodes in sodium storage. Adv. Funct. Mater. 2024, 34, 2301996.
53. Guan, S.; Fan, Q.; Shen, Z.; Zhao, Y.; Sun, Y.; Shi, Z. Heterojunction TiO2@TiOF2 nanosheets as superior anode materials for sodium-ion batteries. J. Mater. Chem. A. 2021, 9, 5720-9.
54. Xu, X.; Chen, B.; Hu, J.; et al. Heterostructured TiO2 spheres with tunable interiors and shells toward improved packing density and pseudocapacitive sodium storage. Adv. Mater. 2019, 31, e1904589.
55. Meng, W.; Dang, Z.; Li, D.; Jiang, L.; Fang, D. Interface and defect engineered titanium-base oxide heterostructures synchronizing high-rate and ultrastable sodium storage. Adv. Energy. Mater. 2022, 12, 2201531.
56. Zhao, Q.; Xia, Z.; Qian, T.; et al. PVP-assisted synthesis of ultrafine transition metal oxides encapsulated in nitrogen-doped carbon nanofibers as robust and flexible anodes for sodium-ion batteries. Carbon 2021, 174, 325-34.
57. Hou, T.; Liu, B.; Sun, X.; et al. Covalent coupling-stabilized transition-metal sulfide/carbon nanotube composites for lithium/sodium-ion batteries. ACS. Nano. 2021, 15, 6735-46.
58. Chen, Y.; Liu, H.; Guo, X.; et al. Bimetallic sulfide SnS2/FeS2 nanosheets as high-performance anode materials for sodium-ion batteries. ACS. Appl. Mater. Interfaces. 2021, 13, 39248-56.
59. Muhammad M, Liu Y, Sheng L, Haruna B, Hu X, Wen Z. Phase engineering of nickel-based sulfides toward robust sodium-ion batteries. J. Colloid. Interface. Sci. 2023, 646, 245-53.
60. Wang, X.; Zhang, X.; Chen, Y.; Dong, J.; Zhao, J. Optimizing electron spin-polarized states of MoSe2/Cr2Se3 heterojunction-embedded carbon nanospheres for superior sodium/potassium-ion battery performances. Small 2024, 20, e2312130.
61. Chen, H.; Tian, P.; Fu, L.; Wan, S.; Liu, Q. Hollow spheres of solid solution Fe7Ni3S11/CN as advanced anode materials for sodium ion batteries. Chem. Eng. J. 2022, 430, 132688.
62. Wang, Z.; Dong, K.; Wang, D.; et al. Constructing N-doped porous carbon confined FeSb alloy nanocomposite with Fe-N-C coordination as a universal anode for advanced Na/K-ion batteries. Chem. Eng. J. 2020, 384, 123327.
63. Ma, W.; Wang, J.; Gao, H.; et al. A mesoporous antimony-based nanocomposite for advanced sodium ion batteries. Energy. Storage. Mater. 2018, 13, 247-56.
64. Edison, E.; Sreejith, S.; Ren, H.; Lim, C. T.; Madhavi, S. Microstructurally engineered nanocrystalline Fe–Sn–Sb anodes: towards stable high energy density sodium-ion batteries. J. Mater. Chem. A. 2019, 7, 14145-52.
65. Chen, L.; He, X.; Chen, H.; Huang, S.; Wei, M. N-doped carbon encapsulating Bi nanoparticles derived from metal–organic frameworks for high-performance sodium-ion batteries. J. Mater. Chem. A. 2021, 9, 22048-55.
66. Gao, H.; Niu, J.; Zhang, C.; Peng, Z.; Zhang, Z. A dealloying synthetic strategy for nanoporous bismuth-antimony anodes for sodium ion batteries. ACS. Nano. 2018, 12, 3568-77.
67. Ma, W.; Yin, K.; Gao, H.; Niu, J.; Peng, Z.; Zhang, Z. Alloying boosting superior sodium storage performance in nanoporous tin-antimony alloy anode for sodium ion batteries. Nano. Energy. 2018, 54, 349-59.
68. Lei, S.; Qiu, M.; Hu, X.; et al. Heteroatomic phosphorus selenides molecules encapsulated in porous carbon as a highly reversible anode for sodium-ion batteries. Mater. Today. Nano. 2023, 22, 100344.
69. Xu, Z.; Wang, J.; Guo, Z.; et al. The role of hydrothermal carbonization in sustainable sodium-ion battery anodes. Adv. Energy. Mater. 2022, 12, 2200208.
70. Lu, Y.; Zhao, C.; Qi, X.; et al. Pre-oxidation-tuned microstructures of carbon anodes derived from pitch for enhancing Na storage performance. Adv. Energy. Mater. 2018, 8, 1800108.
71. Zhao, J.; He, X.; Lai, W.; et al. Catalytic defect-repairing using manganese ions for hard carbon anode with high-capacity and high-initial-coulombic-efficiency in sodium-ion batteries. Adv. Energy. Mater. 2023, 13, 2300444.
72. Wang, J.; Zhao, J.; He, X.; Qiao, Y.; Li, L.; Chou, S. Hard carbon derived from hazelnut shell with facile HCl treatment as high-initial-coulombic-efficiency anode for sodium ion batteries. Sustainable. Mater. Technol. 2022, 33, e00446.
73. Meng, Q.; Lu, Y.; Ding, F.; Zhang, Q.; Chen, L.; Hu, Y. Tuning the closed pore structure of hard carbons with the highest Na storage capacity. ACS. Energy. Lett. 2019, 4, 2608-12.
74. Kamiyama, A.; Kubota, K.; Igarashi, D.; et al. MgO-template synthesis of extremely high capacity hard carbon for Na-ion battery. Angew. Chem. Int. Ed. Engl. 2021, 60, 5114-20.
75. Li, Y.; Lu, Y.; Meng, Q.; et al. Regulating pore structure of hierarchical porous waste cork-derived hard carbon anode for enhanced Na storage performance. Adv. Energy. Mater. 2019, 9, 1902852.
76. Li, Q.; Liu, X.; Tao, Y.; et al. Sieving carbons promise practical anodes with extensible low-potential plateaus for sodium batteries. Natl. Sci. Rev. 2022, 9, nwac084.
77. Ma, L.; Gao, X.; Zhang, W.; et al. Ultrahigh rate capability and ultralong cycling stability of sodium-ion batteries enabled by wrinkled black titania nanosheets with abundant oxygen vacancies. Nano. Energy. 2018, 53, 91-6.
78. Han, M.; Zou, Z.; Liu, J.; et al. Pressure-induced defects and reduced size endow TiO2 with high capacity over 20 000 cycles and excellent fast-charging performance in sodium ion batteries. Small 2024, 20, e2312119.
79. Hwang, J.; Du, H.; Yun, B.; et al. Carbon-free TiO2 microspheres as anode materials for sodium ion batteries. ACS. Energy. Lett. 2019, 4, 494-501.
80. Yang, J.; Huang, M.; Xu, L.; Xia, X.; Peng, C. Self-assembled titanium-deficient undoped anatase TiO2 nanoflowers for ultralong-life and high-rate Li+/Na+ storage. Chem. Eng. J. 2022, 445, 136638.
81. Lan, K.; Liu, L.; Zhang, J. Y.; et al. Precisely designed mesoscopic titania for high-volumetric-density pseudocapacitance. J. Am. Chem. Soc. 2021, 143, 14097-105.
82. Xia, Q.; Liang, Y.; Lin, Z.; et al. Confining ultrathin 2D superlattices in mesoporous hollow spheres renders ultrafast and high-capacity Na-ion storage. Adv. Energy. Mater. 2020, 10, 2001033.
83. Liu, S.; Niu, K.; Chen, S.; et al. TiO2 bunchy hierarchical structure with effective enhancement in sodium storage behaviors. Carbon. Energy. 2022, 4, 645-53.
84. Yang, D.; Xu, B.; Zhao, Q.; Zhao, X. S. Three-dimensional nitrogen-doped holey graphene and transition metal oxide composites for sodium-ion batteries. J. Mater. Chem. A. 2019, 7, 363-71.
85. Khan, R.; Yan, W.; Ahmad, W.; et al. Role of moderate strain engineering in Nickel Sulfide anode for advanced sodium-ion batteries. J. Alloys. Compd. 2023, 963, 171196.
86. Li, R.; Dong, W.; Pan, J.; Huang, F. Micrometer-sized, dual-conductive MoO2/β-MoO3-x mosaics for high volumetric capacity Li/Na-ion batteries. Small. Methods. 2021, 5, e2100765.
87. Wang, B.; Li, F.; Wang, X.; Wang, G.; Wang, H.; Bai, J. Mn3O4 nanotubes encapsulated by porous graphene sheets with enhanced electrochemical properties for lithium/sodium-ion batteries. Chem. Eng. J. 2019, 364, 57-69.
88. Zhang, K.; Xiong, F.; Zhou, J.; Mai, L.; Zhang, L. Universal construction of ultrafine metal oxides coupled in N-enriched 3D carbon nanofibers for high-performance lithium/sodium storage. Nano. Energy. 2020, 67, 104222.
89. Xu, M.; Xia, Q.; Yue, J.; et al. Rambutan-like hybrid hollow spheres of carbon confined Co3O4 nanoparticles as advanced anode materials for sodium-ion batteries. Adv. Funct. Mater. 2019, 29, 1807377.
90. Zhao, Y.; Wang, F.; Wang, C.; et al. Encapsulating highly crystallized mesoporous Fe3O4 in hollow N-doped carbon nanospheres for high-capacity long-life sodium-ion batteries. Nano. Energy. 2019, 56, 426-33.
91. Cao, L.; Gao, X.; Zhang, B.; Ou, X.; Zhang, J.; Luo, W. B. Bimetallic sulfide Sb2S3@FeS2 hollow nanorods as high-performance anode materials for sodium-ion batteries. ACS. Nano. 2020, 14, 3610-20.
92. Liu, J.; Li, Y.; Chen, Z.; et al. Polyoxometalate cluster-incorporated high entropy oxide sub-1 nm nanowires. J. Am. Chem. Soc. 2022, 144, 23191-7.
93. Yang, H.; Chen, L. W.; He, F.; et al. Optimizing the void size of yolk-shell Bi@Void@C nanospheres for high-power-density sodium-ion batteries. Nano. Lett. 2020, 20, 758-67.
94. Fan, X.; Han, J.; Ding, Y.; et al. 3D nanowire arrayed Cu current collector toward homogeneous alloying anode deposition for enhanced sodium storage. Adv. Energy. Mater. 2019, 9, 1900673.
95. Dang, J.; Zhu, R.; Zhang, S.; et al. Bean pod-like SbSn/N-doped carbon fibers toward a binder free, free-standing, and high-performance anode for sodium-ion batteries. Small 2022, 18, e2107869.
96. Song, Z.; Wang, G.; Chen, Y.; Lu, Y.; Wen, Z. In situ three-dimensional cross-linked carbon nanotube-interspersed SnSb@CNF as freestanding anode for long-term cycling sodium-ion batteries. Chem. Eng. J. 2023, 463, 142289.
97. Kim, Y. H.; An, J. H.; Kim, S. Y.; et al. Enabling 100C fast-charging bulk Bi anodes for Na-ion batteries. Adv. Mater. 2022, 34, e2201446.
98. Eaves-Rathert, J.; Moyer-Vanderburgh, K.; Wolfe, K.; Zohair, M.; Pint, C. L. Leveraging impurities in recycled lead anodes for sodium-ion batteries. Energy. Storage. Mater. 2022, 53, 552-8.
99. Fang, H.; Gao, S.; Ren, M.; et al. Dual-function presodiation with sodium diphenyl ketone towards ultra-stable hard carbon anodes for sodium-ion batteries. Angew. Chem. Int. Ed. Engl. 2023, 62, e202214717.
100. Bai, P.; Han, X.; He, Y.; et al. Solid electrolyte interphase manipulation towards highly stable hard carbon anodes for sodium ion batteries. Energy. Storage. Mater. 2020, 25, 324-33.
101. Jin, Y.; Xu, Y.; Xiao, B.; et al. Stabilizing interfacial reactions for stable cycling of high-voltage sodium batteries. Adv. Funct. Mater. 2022, 32, 2204995.
102. Jin, Y.; Xu, Y.; Le, P. M. L.; et al. Highly reversible sodium ion batteries enabled by stable electrolyte-electrode interphases. ACS. Energy. Lett. 2020, 5, 3212-20.
103. Li, K.; Zhang, J.; Lin, D.; et al. Evolution of the electrochemical interface in sodium ion batteries with ether electrolytes. Nat. Commun. 2019, 10, 725.
104. Meng, W.; Dang, Z.; Li, D.; Jiang, L. Long-cycle-life sodium-ion battery fabrication via a unique chemical bonding interface mechanism. Adv. Mater. 2023, 35, e2301376.
105. Xu, Z.; Lim, K.; Park, K.; Yoon, G.; Seong, W. M.; Kang, K. Engineering solid electrolyte interphase for pseudocapacitive anatase TiO2 anodes in sodium-ion batteries. Adv. Funct. Mater. 2018, 28, 1802099.
106. Cha, G.; Mohajernia, S.; Nguyen, N. T.; et al. Li+ pre-insertion leads to formation of solid electrolyte interface on TiO2 nanotubes that enables high-performance anodes for sodium ion batteries. Adv. Energy. Mater. 2020, 10, 1903448.
107. Li, Q.; Cao, Z.; Cheng, H.; et al. Electrolyte boosting microdumbbell-structured alloy/metal oxide anode for fast-charging sodium-ion batteries. ACS. Mater. Lett. 2022, 4, 2469-79.
108. Yang, J.; Guo, X.; Gao, H.; et al. A high-performance alloy-based anode enabled by surface and interface engineering for wide-temperature sodium-ion batteries. Adv. Energy. Mater. 2023, 13, 2300351.
109. Chu, C.; Zhou, L.; Cheng, Y.; et al. Ultralow-concentration (0.1M) electrolyte for stable bulk alloy (Sn, Bi) anode in sodium-ion battery via regulating anions structure. Chem. Eng. J. 2024, 482, 148915.
110. Huang, J.; Guo, X.; Du, X.; et al. Nanostructures of solid electrolyte interphases and their consequences for microsized Sn anodes in sodium ion batteries. Energy. Environ. Sci. 2019, 12, 1550-7.
111. Wu, X.; Li, Z.; Feng, W.; et al. Insights into electrolyte-induced temporal and spatial evolution of an ultrafast-charging Bi-based anode for sodium-ion batteries. Energy. Storage. Mater. 2024, 66, 103219.
112. Zhao, B.; Han, J.; Liu, B.; Zhang, S. L.; Guan, B. Hierarchical metal–organic framework nanoarchitectures for catalysis. Chem. Synth. 2024, 4, 41.
113. Peng, Y.; Tan, Q.; Huang, H.; et al. Customization of functional MOFs by a modular design strategy for target applications. Chem. Synth. 2022, 2, 15.
114. Li, H.; Li, C.; Wang, Y.; et al. Selenium confined in ZIF-8 derived porous carbon@MWCNTs 3D networks: tailoring reaction kinetics for high performance lithium-selenium batteries. Chem. Synth. 2022, 2, 8.
115. Su, Y.; Yuan, G.; Hu, J.; et al. Recent progress in strategies for preparation of metal-organic frameworks and their hybrids with different dimensions. Chem. Synth. 2022, 3, 1.
116. Wang, R.; Zhao, J.; Fang, Q.; Qiu, S. Advancements and applications of three-dimensional covalent organic frameworks. Chem. Synth. 2024, 4, 29.
117. Li, X.; Geng, K.; Fu, S.; Jin, E. Molecular engineering toward large pore-sized covalent organic frameworks. Chem. Synth. 2024, 4, 15.
118. Yin, X.; Sarkar, S.; Shi, S.; et al. Recent progress in advanced organic electrode materials for sodium-ion batteries: synthesis, mechanisms, challenges and perspectives. Adv. Funct. Mater. 2020, 30, 1908445.
119. Lee, J.; Kim, Y.; Park, S.; et al. Sodium-coordinated polymeric phthalocyanines as stable high-capacity organic anodes for sodium-ion batteries. Energy. Environ. Mater. 2023, 6, e12468.
Cite This Article
How to Cite
Download Citation
Export Citation File:
Type of Import
Tips on Downloading Citation
Citation Manager File Format
Type of Import
Direct Import: When the Direct Import option is selected (the default state), a dialogue box will give you the option to Save or Open the downloaded citation data. Choosing Open will either launch your citation manager or give you a choice of applications with which to use the metadata. The Save option saves the file locally for later use.
Indirect Import: When the Indirect Import option is selected, the metadata is displayed and may be copied and pasted as needed.
About This Article
Copyright
Data & Comments
Data
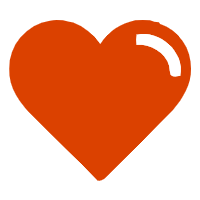
Comments
Comments must be written in English. Spam, offensive content, impersonation, and private information will not be permitted. If any comment is reported and identified as inappropriate content by OAE staff, the comment will be removed without notice. If you have any queries or need any help, please contact us at [email protected].