Efficient adsorptive removal of perfluorooctanoic acid by large surface area biochar modified with KHCO3
Abstract
Biochar is a promising adsorbent for remediating perfluorooctanoic acid (PFOA) in contaminated water. However, the performance of pristine biochar is limited. Considering pore filling is a crucial mechanism for PFOA adsorption on biochar, this study investigated the impact of KHCO3 modification on the pore structure of biochar and its adsorption capacity for PFOA. The characterization and experiment results revealed that both the specific surface area (SSA) and adsorption capacity of biochars were positively correlated with the pyrolysis temperature, and modified corn stalks biochar prepared at 800 °C (CBC-800) exhibited a noticeable SSA (1,471.6 m2/g) and adsorption capacity (514.8 mg/g), which were 3.6 and 37.1 times higher, respectively, compared to pristine corn stalk biochar (404.1 m2/g and 13.9 mg/g). The adsorption kinetics and the isotherm data followed pseudo-second-order kinetics and the Freundlich model, respectively, indicating chemisorption was the main factor limiting the adsorption rate. Thermodynamics demonstrated the adsorption process was physical, spontaneous, and exothermic. The porous biochars performed superior adsorption capacities under various environmental conditions (pH, inorganic salts, etc.). The removal rate of CBC-800 for low concentrations of PFOA (10-1,000 μg/g) ranged from 87.4% to 99.6%, and this rate was positively correlated with the initial concentrations. Additionally, CBC-800 effectively removed PFOA (40 μg/L) through six consecutive adsorption cycles (93.4%-94.5%). Mechanism analysis indicated dominant pore filling was greatly enhanced, while hydrogen bonding, electrostatic, and hydrophobic interactions were also involved. Our study demonstrated that biochar derived from low-cost agricultural and forestry residues combining KHCO3 modification has great potential for the adsorptive removal of emerging PFAS in contaminated water.
Keywords
INTRODUCTION
Perfluorooctanoic acid (PFOA) is a synthetic perfluoroalkyl substance (PFAS) that has distinctive properties such as hydrophilic and lipophilic characteristics, attributed to its unique amphiphilic structure consisting of a hydrophilic carboxyl head and a hydrophobic perfluoro tail[1,2]. It is extensively utilized in numerous products, including food contact paper, firefighting foam, non-stick cookware, and industrial antifouling agents[3,4]. Due to the pervasive use of these products, PFOA has been widely detected in a variety of environmental media, including municipal sewage and underground water[5,6]. The C–F bond of PFOA possesses a bond energy of about 530 kJ/mol, providing remarkable stability and rendering it resistant to environmental degradation[7]. PFOA has been found to have an increased risk of cardiovascular, respiratory, and neurological disease[8]. Due to growing concerns over the environmental (especially the water system) and health risks posed by PFOA, it is classified as an “emerging contaminant”. As a result, there is significant public concern regarding the remediation of PFOA-contaminated water.
Various technologies have been employed to eliminate PFOA from water, including membrane separation, adsorption, advanced oxidation processes, electrochemical degradation, photochemical degradation, etc.[2,9,10]. Among these, electrochemical and photochemical methods have obtained a lot of attention due to their high efficiency in degrading PFOA contaminants in aqueous environments. However, these methods often require additional energy input and specialized conditions, which may limit their practical application in certain scenarios. Adsorption is a promising technology due to its environmental friendliness, ease of operation, and renewability[11]. Biochar can serve as an attractive adsorbent with regulable physiochemical properties, abundant forestry and agricultural residues, simple preparation processes, and renewability[12,13]. However, conventional biochars have limited adsorption sites, a low specific surface area (SSA), and porosity, making it difficult to efficiently remove PFOA[14]. It has been documented that the porosity of the adsorbents plays a critical factor in the adsorption of PFAS[15,16]. Therefore, improving the pore structure of biochar could effectively enhance its adsorption capacity for PFOA. Currently, the predominant methods to increase the SSA of biochars involve chemical modification. For instance, Fagbayigbo et al. prepared grape leaf biochar at 900 °C and activated it with H3PO4, achieving a maximum adsorption capacity (Qm) of
Modification with KHCO3, as a mild and non-corrosive modification method, can increase biochar adsorption capacities for pollutants by improving the pore structure[19]. For instance, Wang et al. indicated that KHCO3-modified biochar pyrolyzed at 900 °C (PBC-900) exhibited a remarkably larger SSA
In this study, pine sawdust and corn stalks were chosen as biochar precursors owing to their widespread availability and representation of cellulose and lignin biomass[22], respectively. The porous biochar was prepared via KHCO3 modification and used for PFOA adsorption. The following were the study’s aims: (1) preparation and characterization of porous biochars obtained from different pyrolysis temperatures and biomass using KHCO3 modification; (2) comparison of PFOA adsorption on different obtained biochars; and (3) elucidation of the underlying mechanism for the enhancement of PFOA adsorption on porous biochar. This study used KHCO3 modification to enhance the adsorption capacity of biochars derived from widely available biomass, providing valuable insights into the development of sustainable and efficient materials for the removal of PFOA from contaminated water.
MATERIAL AND METHODS
Chemicals
PFOA (C8HF15O2), potassium hydrogen carbonate (KHCO3), sodium hydroxide (NaOH), and ethyl alcohol (EtOH) were supplied from Macklin Biochemical (Shanghai, China). Hydrochloric acid (HCl), sodium chloride (NaCl), and calcium chloride (CaCl2) were obtained from Sinopharm Group Chemical Reagent (Beijing, China). Acetonitrile and methanol were all HPLC quality from ANPEL Laboratory Technologies (Shanghai, China). Sodium dihydrogen phosphate (NaH2PO4) was obtained from Beijing Reagent (Beijing, China). Deionized water (DIW, > 18.25 MΩ·cm) was used during experiments. More information can be found in the Supplementary Table 1.
Preparation of biochar
After washing and drying the biomass corn stalks and pine sawdust, a 100-200 mesh screen was used to sift the biomass powder. The biomass powders and KHCO3 were magnetically stirred in a beaker containing DIW at a mass ratio of 2:1 for 1 h before being dried in an oven at 80 °C. Next, the mixtures were placed in a corundum boat and maintained for 2 h in a tubular furnace at the desired temperature, such as 600, 700, and 800 °C, with a heating rate of 5 °C/min. The pyrolysis temperature and the ratio of KHCO3 to biomass were selected according to the pre-experiment [Supplementary Figure 1]. After natural cooling to room temperature, the solid products were rinsed with 0.1 M HCl and DIW until the pH of the filtrate was neutral before being dried in an oven. The resulting modified pine sawdust and corn stalks biochar were denoted as PBC-T and CBC-T, respectively, where T represents the pyrolysis temperatures.
Characterization of biochar
The morphologies of porous biochars were observed by scanning electron microscopy (SEM, Zeiss Supra 55-VP). The pore structure of porous biochars was analyzed using the Brunauer-Emmett-Teller method (BET, QuadraSorb Station 1 analyzer). Biochars were heated for 4 h at 750 °C in a muffle furnace in order to measure their ash content. The functional groups of porous biochars were identified by Fourier transform infrared spectroscopy (FTIR, Nicolet iS10 spectrometer). The content of elements in porous biochars was obtained by an elemental analyzer (Euro Vector EA300), and the O content was inferred from the residual mass of these elements and ash content. The graphitization degree and defects of porous biochars were analyzed by Raman spectra (inVia Reflex, UK). This study used a zeta potential analyzer to measure the zeta potential of porous biochars (NanoBrook Omni).
Adsorption experiments
The PFOA solution was configured with 10 mM NaCl as the background solution, and 0.1 M HCl and NaOH were used to adjust the pH to 7 ± 0.2. Biochars (0.2 g/L) were added to glass bottles containing
For adsorption thermodynamics, an accurate 10 mg of biochar was added to glass bottles containing 50 mL of PFOA solution at a range of concentrations (30, 50, 80, 120, 160, 200, and 240 mg/L) and shaken in a shaker at desired temperatures (15, 25, 35 °C) at 180 rpm for 1 day. After equilibrium, the supernatant was extracted to quantify the PFOA concentration. In addition, the desorption experiments were performed immediately after adsorption reached equilibrium. Specifically, the liquid in the centrifugal tube was carefully removed, and different desorption solutions (DI water, 0.5 M NaOH, and 25%-95% ethanol) were added, and the PFOA concentration was determined after shaking for 48 h. The pH effect experiments on PFOA adsorption by biochar were investigated by changing the initial pH (3, 5, 7, 9, 11) of the solution. The influence of co-existing ions was compared by changing the concentrations of NaCl, CaCl2, NaNO3, and
To further assess the PFOA removal efficiency of CBC-800 at low concentrations, CBC-800 (0.2 g/L) was added into a glass bottle containing 50 mL of PFOA solutions at concentrations ranging from 0.01 to
PFOA analysis
The PFOA was quantitively measured according to our previous study[23]. Briefly, the concentration of PFOA was quantified by HPLC (Shimadzu LC-20 A, Kyoto, Japan) equipped with a C18 column (250 mm × 4.6 mm, 5 μm). The iso-volumetric elution of acetonitrile/20 mM NaH2PO4 (50/50, v/v) (pH 2) was performed at a flow rate of 1 mL/min with an injection volume of 25 μL, and the UV wavelength was
RESULTS AND DISCUSSION
Characterization of biochar
The microscopic morphologies of biochars are shown in Supplementary Figure 2. Both pine sawdust-modified biochars (PBC) and corn stalks-modified biochars (CBC) exhibited abundant etched pits and well-developed pore structures on their surfaces, indicating that the porous structures of biochars were largely improved after being activated with KHCO3, as evidenced by the comparison with the pristine corn stalk biochar in our previous study[23]. Additionally, biochars produced at higher pyrolysis temperatures displayed more intensive pits. This can be attributed to the decomposition of KHCO3 into K and K2O at high temperatures, with the K and K2O embedded in biochar promoting the formation of micropores [Equations (1)-(4)][24]. As pyrolysis temperature increased, the decomposition rate of KHCO3 accelerated, leading to a more thorough decomposition and consequently a larger SSA. This enhancement in SSA was favorable for the efficient transport of PFOA.
To further clarify the pore structure of porous biochars, the N2 adsorption/desorption curve was conducted, and the results were presented in Table 1 and Figure 1. The SBET of PBC and CBC increased significantly with the pyrolysis temperature, from 672.38 to 1,253.46 m2/g for PBC, and from to 1,471.6 m2/g for CBC, when the pyrolysis temperature was raised from 600 to 800 °C. Notably, the SBET of CBC-800 obviously exceeded that of the corn stalk biochar (404.10 m2/g) reported in our previous study[23]. This increase in SBET could be attributed to the reaction between KHCO3 and biochar, resulting in the formation of K and K2O, which become embedded in the biochar and promote the formation of micropores[25]. Furthermore, at the same pyrolysis temperature, CBC exhibited a higher SSA than PBC due to its higher content of cellulose[26].
Figure 1. (A) N2 adsorption-desorption isotherm of biochars and (B) pore size distribution curve measured by BJH method. BJH: Barrett-Joyner-Halenda.
Physical properties of porous biochars
Biochars | SBETa (m2/g) | Smicb (m2/g) | Smesc (m2/g) | Vtotald (cm3/g) | Vmice (cm3/g) | Ave-pore diameter (nm) |
PBC-600 | 672.39 | 619.99 | 52.39 | 0.32 | 0.25 | 1.92 |
PBC-700 | 955.89 | 892.73 | 63.14 | 0.51 | 0.42 | 2.15 |
PBC-800 | 1,253.46 | 1,173.81 | 79.65 | 0.60 | 0.49 | 1.91 |
CBC-600 | 765.48 | 672.91 | 92.56 | 0.42 | 0.28 | 2.17 |
CBC-700 | 1,197.19 | 1,015.54 | 181.65 | 0.81 | 0.57 | 2.71 |
CBC-800 | 1,471.64 | 1,361.86 | 109.78 | 0.81 | 0.62 | 2.20 |
The elemental analysis data and ash content for porous biochars are presented in Table 2. It was found that the ash content of PBC was lower than that of CBC, which could be attributed to the difference in the cellulose content of biomass. The atomic ratios analysis could indicate the aromatics and polarity of porous biochars[27]. The atomic ratio of H/C for PBC and CBC decreased from 0.219 to 0.065 and 0.141 to 0.048, respectively, demonstrating the enhancement in the aromaticity of porous biochars as the pyrolysis temperatures increased from 600 to 800 °C. Moreover, the aromaticity of CBC-800 was notably higher than that of the other biochars, suggesting that CBC-800 was favored for adsorbing aromatic pollutants. Based on Table 2, the analogous O/C and (O + N)/C atomic ratios of PBC (0.055-0.075 and 0.064-0.084) and CBC (0.058-0.154 and 0.072-0.168) indicated the porous biochars possessed similar hydrophilicity.
Elemental composition, atomic ratio, ash content and yield of biochars
Biochars | Element content (%) | Atomic ratio | Ash content (%) | Yield (%) | ||||||
C | H | N | S | O | H/C | O/C | (O + N)/C | |||
PBC-600 | 90.09 | 1.65 | 0.89 | 0.22 | 6.61 | 0.219 | 0.055 | 0.064 | 0.54 | 20.53 |
PBC-700 | 88.70 | 0.89 | 0.88 | 0.25 | 8.92 | 0.121 | 0.075 | 0.084 | 0.36 | 13.71 |
PBC-800 | 89.35 | 0.49 | 1.08 | 0.24 | 7.68 | 0.065 | 0.064 | 0.075 | 1.16 | 12.72 |
CBC-600 | 84.18 | 0.99 | 1.03 | 0.15 | 12.07 | 0.141 | 0.108 | 0.118 | 1.58 | 21.45 |
CBC-700 | 78.63 | 0.91 | 1.22 | 0.24 | 16.20 | 0.139 | 0.154 | 0.168 | 2.80 | 14.87 |
CBC-800 | 86.17 | 0.35 | 1.42 | 0.27 | 6.69 | 0.048 | 0.058 | 0.072 | 5.10 | 10.04 |
To further investigate the defects and the graphitization degree of biochars, Raman spectra were characterized, as shown in Figure 2. The ID/IG ratio could measure the graphite defects of porous biochars[16]. The ID/IG ratio of CBC (0.98-1.01) was obviously higher than PBC (0.90-0.99), indicating that CBC possessed more graphite defects. Both PBC and CBC showed an increase in the ID/IG ratio with increasing pyrolysis temperature, from 0.90 to 0.99 for PBC and 0.98 to 1.01 for CBC. This suggested that higher pyrolysis temperature contributed to the formation of graphite defects in biochars, which may explain the observed increase in SBET and pore formation. The graphite defects in the pores of porous biochars can provide rapid diffusion channels for the organic compounds, thereby promoting the adsorption of organic compounds[28].
Biochar had a lot of functional groups that played a critical role in the adsorption of organic pollutants[29]. The FTIR spectra of the porous biochars before adsorption of PFOA was shown in Supplementary Figure 3A. Typical functional groups exhibited large absorption peaks on the spectrum, such as -OH and -CHx stretching vibration at 3,483 cm-1 and 2,822-2,971 cm-1, respectively. In addition, the absorption peaks of
PFOA adsorption
The adsorption kinetics could reflect the rate at which adsorbates were adsorbed on the adsorbents, providing valuable information such as the kinetic adsorption constant. The adsorption kinetics of biochars for PFOA are shown in Figure 3A and B. It was observed that different biochars exhibited a similar trend in PFOA adsorption, with rapid PFOA removal occurring within the initial 60 min due to the abundance of active sites on their surfaces, facilitating swift uptake. Subsequently, as these sites were occupied, the adsorption rate gradually decreased until equilibrium was reached. Therefore, the PFOA adsorption process can be divided into distinct phases: an initial phase characterized by rapid adsorption (0-1 h) and a slower phase (1-24 h). The adsorption kinetics data for the porous biochars were fitted to the kinetic models, and the kinetic constants were shown in Supplementary Table 2. From the correlation coefficient (R2) in Supplementary Table 2, compared with the pseudo-first-order kinetic model (0.891-0.958), both the pseudo-second-order kinetic model (0.963-0.993) and the Elovich model (0.963-0.985) showed better fits. Furthermore, the experiment data better fitted the pseudo-second-order kinetic model, suggesting that chemisorption was the rate-limiting stage of biochar adsorption[31,32]. The α value of the Elovich model exceeded β, indicating a faster adsorption rate compared to the desorption process, suggesting a strong affinity between the PFOA and the adsorbent[33].
Figure 3. (A and B) Adsorption kinetics; (C and D) adsorption isotherms of biochars for PFOA; and (E and F) adsorption thermodynamics of PBC-800 and CBC-800 for PFOA. PFOA: Perfluorooctanoic acid; PBC: pine sawdust-modified biochars; CBC: corn stalks-modified biochars.
The maximal adsorption capacities of porous biochars were evaluated through the adsorption isotherm. The experiment data were fitted to the Langmuir and Freundlich models [Figure 3C and D], and the parameters obtained from these models offer valuable insights into the adsorption mechanism. According to the R2 in Supplementary Table 3, PFOA adsorption on porous biochars prepared at pyrolysis temperatures of 700 and 800 °C showed a better fit to the Freundlich model (0.969-0.995) than the Langmuir model (0.837-0.920), indicating the presence of multilayer adsorption[34]. The Langmuir and Freundlich models are based on the assumption of homogeneous and heterogeneous adsorbent surfaces, respectively. The Raman spectra of porous biochars possessed high enough D bands [Figure 2], suggesting the existence of heterogeneous surface sites, which was consistent with the model fitting results. In the Langmuir model, the adsorption affinity coefficient (KL) was obtained, with CBC-800 exhibiting a higher KL value (0.14) compared to other biochars (0.04-0.08). This suggested a stronger binding affinity between CBC-800 and PFOA[35]. In the Freundlich model, the n of porous biochars (0.26-0.35) indicated nonlinear sorption isotherms. The maximal adsorption capacities of porous biochars were significantly impacted by the pyrolysis temperatures. Specifically, PBC-800 and CBC-800 displayed impressive adsorption performance of 440.3 and 514.8 mg/g, respectively, whereas CBC-600, PBC-700, and CBC-700 exhibited lower adsorption capacities ranging from 82.1 to 239.7 mg/g. Notably, CBC-800 demonstrated exceptional adsorption performance (514.8 mg/g) compared to other adsorbents, such as unmodified corn stalks biochar
Furthermore, to investigate the spontaneity of biochar adsorption of PFOA, the superior adsorption capacities of CBC-800 and PBC-800 were selected for adsorption thermodynamics analysis
The obtained thermodynamic parameters are shown in Table 3. Within the temperature range of 298-318 K, all ΔG0 values (-10.59 to -12.30 kJ/mol) were negative, confirming that the adsorption processes were spontaneous[37]. In addition, the negative ΔH0 values (-9.20 and -18.30 kJ/mol) for PBC-800 and CBC-800 indicated that the adsorption process was exothermic[38]. The negative ΔG0 and ΔH0 values for both PBC-800 and CBC-800 represented that the adsorption was dominated by physical adsorption[39]. The parameter ΔS0 could quantify the degree of disorder during the biochar adsorption process. The positive ΔS0 values
Thermodynamic parameters of adsorption of PFOA by biochars
Biochars | T (K) | Freundlich | ΔG0 (kJ/mol) | ΔH0 (kJ/mol) | ΔS0 (J·mol-1·K-1) | ||
Kf (mg1-n·g-1·Ln) | n | R2 | |||||
PBC-800 | 288 | 102.11 ± 5.67 | 0.30 ± 0.01 | 0.994 | -11.08 | -9.20 | 5.92 |
298 | 71.89 ± 10.16 | 0.35 ± 0.03 | 0.975 | -10.59 | |||
308 | 79.97 ± 6.55 | 0.26 ± 0.02 | 0.982 | -11.22 | |||
CBC-800 | 288 | 170.38 ± 23.47 | 0.25 ± 0.03 | 0.956 | -12.30 | -18.30 | -20.61 |
298 | 141.67 ± 8.93 | 0.28 ± 0.01 | 0.991 | -12.27 | |||
308 | 103.57 ± 13.77 | 0.33 ± 0.03 | 0.974 | -11.88 |
The ionization degree of PFOA molecules and the charge of the biochars were impacted by the pH of the solution[41]. The influence of pH on porous biochar adsorption performances is illustrated in Figure 4A. It was shown that the PFOA adsorption capacity of CBC-800 (244.8 mg/g) and PBC-800 (240.6 mg/g) was optimal at pH 3, with adsorption gradually declining as pH increased. Although the adsorption performance of biochars decreased as the pH increased, both CBC-800 and PBC-800 also possessed considerable adsorption capacities of 160.6 and 164.4 mg/g, respectively. This demonstrated that CBC-800 and PBC-800 were capable of effectively adsorbing PFOA across a wide pH range.
Figure 4. Effect of (A) pH, (B) Na+, and (C) Ca2+ ions on the adsorption of PFOA by biochars. PFOA: Perfluorooctanoic acid.
The adsorption of PFOA by biochar was also influenced by the presence of ions in the solution, except pH. The impact of co-existing Na+ and Ca2+ ions at varying concentrations on the porous biochar adsorption performances is illustrated in Figure 4B and C. It was observed that the adsorption performance of porous biochars increased as the concentrations of Na+ and Ca2+ increased. This was due to the fact that the compressed double electric layer effect resulted in a decrease in the negative charge of biochars, thus weakening the electrostatic repulsion between biochars and PFOA molecules (and between the adsorbed PFOA anion)[42]. In comparison with Na+, Ca2+ demonstrated a more pronounced enhancement of the adsorption capacities of biochars for PFOA, with this effect becoming more evident at higher Ca2+ concentrations. PFOA molecules contained carboxyl groups, while the surface of biochar contained hydroxyl and carboxyl functional groups [Supplementary Figure 3A]. Ca2+ could serve as a bridge bond between the deprotonated PFOA molecules and the functional groups of biochars, thereby promoting the adsorption of PFOA[43]. The influence of NO3- and SO42- on the adsorption of PFOA by biochar was investigated [Supplementary Figure 4]. As the concentrations of NO3- and SO42- increased from 0 to 50 mM, the adsorption of PFOA by biochar was significantly enhanced. This increase was mainly due to the salting-out effects[34]. Furthermore, it was observed that the enhancement of Na2SO4 for adsorption was lower than NaNO3. This may be due to the fact that SO42- was more likely to compete with PFOA for adsorption sites on biochar[44].
To validate the application of CBC-800 in relevant environment concentrations, an adsorption experiment was conducted at low concentrations (10-1,000 μg/L) [Figure 5A]. The results demonstrated that CBC-800 exhibited an excellent removal rate (87.4%) even at an initial concentration of 10 μg/L. Furthermore, the removal efficiency of CBC-800 exhibited a positive correlation with the initial concentration. This was due to that at higher concentrations, there was a larger mass transfer driving force. This meant that the concentration gradient between the bulk solution and the adsorbent surface is greater, which facilitates the movement of PFOA molecules toward the biochar. This leads to an increased rate of adsorption, as the molecules are more likely to collide with and adhere to the biochar surface. However, at lower concentrations, this concentration gradient is much smaller, resulting in a reduced driving force for adsorption and fewer collisions between PFOA molecules and the biochar surface[45]. A recycling experiment was performed to assess the long-term adsorption performance of CBC-800 for PFOA at a concentration of 40 μg/L [Figure 5B]. The results indicated the removal rate of CBC-800 (93.4%-94.5%) did not significantly decline even at six consecutive adsorption cycles. The above findings showed CBC-800 had excellent consecutive adsorption performance at low concentrations of PFOA, making it able to effectively remediate the PFOA-contaminated water.
Figure 5. (A) The removal rate of CBC-800 for different initial concentrations; (B) the adsorption cycles of CBC-800 for low concentration of PFOA; (C) PFOA desorption by different desorption solutions and (D) correlation coefficient between the physical properties of biochars and the maximum adsorption capacity (Qm). CBC: Corn stalks-modified biochars; PFOA: perfluorooctanoic acid.
Adsorption stability was regarded as a key factor for practical application and economic feasibility. The adsorption stability was evaluated through a desorption experiment. PFOA adsorbed on porous biochars was desorbed using DIW, 0.5 M NaOH, and different concentrations of ethanol. As illustrated in Figure 5C, CBC-800 and PBC-800 exhibited low PFOA desorption rates in 0.5 M NaOH (3.6% and 7.4%) and DIW (19.6% and 25.9%), demonstrating CBC-800 and PBC-800 owned excellent adsorption stability for PFOA. The lower desorption rate of CBC-800 was consistent with the result that CBC-800 showed a stronger combination than PBC-800 according to the ΔS0 values of adsorption thermodynamics. Additionally, the PFOA desorption rates of CBC-800 and PBC-800 in ethanol (25%-95%) ranged from 75.5% to 95.1% and 79.4% to 93.2%, respectively, suggesting that low concentrations of ethanol could be effective for biochar regeneration.
Adsorption mechanisms
The adsorption mechanisms of PFOA on adsorbents primarily included hydrophobic interaction, pore filling, electrostatic interaction, and hydrogen bonding[41]. In this study, the porous biochar exhibited notable adsorption performance for PFOA. However, the adsorption mechanisms between KHCO3-modified biochar and PFOA remained unclear.
Hydrophobic interaction was expected to follow a linear isotherm, with the Freundlich nonlinearity factor (n) approaching 1, showing that more hydrophobic interactions were involved in the adsorption of biochars. However, the nonlinearity factor n (0.26-0.35) for all biochars was relatively low, suggesting that hydrophobic interaction was not important for the adsorption of PFOA by porous biochars. Pore filling was a crucial mechanism in PFOA adsorption[46]. The porous biochars had an average pore size of greater than 1.9 nm [Table 1], which allowed PFOA molecules, with a size of approximately 1.1 nm [Supplementary Table 1], to enter their pore structure. Furthermore, the Pearson correlation coefficient (R2) between the Qm of biochars obtained from the Langmuir model [Supplementary Table 3] and the physical properties of biochars [Table 1] showed a strong positive correlation between Qm and both the SBET and Smic, indicating that biochar adsorption capacity was greatly impacted by the pore structure [Figure 5D]. Therefore, pore filling was the dominant adsorption mechanism. For electrostatic interaction, the zeta potential of all biochars was consistently negative across the pH range of 3-11 [Supplementary Figure 5]. Given that the pKa of PFOA was 1.3, the hydrophilic head of PFOA existed as negatively charged ions when pH > pKa[47]. Consequently, as pH increased, electrostatic repulsion between the biochar and PFOA increased, leading to a decrease in biochar adsorption capacity [Figure 4A]. Therefore, electrostatic interaction significantly affected the PFOA adsorption by biochars. The -COOH and -OH functional groups on biochar enabled it to serve as a hydrogen donor. Simultaneously, the deprotonation of carboxyl groups at the head of PFOA molecules facilitates their role as hydrogen acceptors[48]. By comparing the FTIR of biochars before and after PFOA adsorption [Supplementary Figure 3A and B], the -OH of biochars (3,420 cm-1) showed no obvious difference, indicating that hydrogen bonding interaction was not the dominant adsorption mechanism.
In summary, KHCO3 modification significantly increased the SBET of the biochar, resulting in pore filling dominating the PFOA adsorption, and electrostatic interaction played an important role, while hydrogen bonding and hydrophobic interaction also existed, as illustrated in Figure 6.
These findings demonstrated that the KHCO3 modification can significantly enhance the surface properties of the biochar, improving its adsorption capacity for PFOA. KHCO3 is a relatively mild pore-enlarging reagent with minimal environmental impact, making the production of modified biochar economically viable for large-scale water treatment applications[19]. Additionally, the porous biochar could be regenerated with a low concentration of ethanol, which can reduce the overall cost. However, the modification also had some disadvantages. For example, although KHCO3-modified biochar may effectively adsorb PFOA, it may also adsorb other contaminants present in the water[49], leading to competition and reduced efficiency in specific PFOA removal. Organic matter in water, such as HA, may interact with the biochar, and the part adsorption sites were occupied[24], leading to a decrease in the adsorption capacity. While KHCO3-modified biochar presented a promising solution for PFOA removal, careful consideration of its advantages and disadvantages is essential for its effective application in environmental remediation strategies.
CONCLUSIONS
In this study, low-cost and environmentally friendly porous biochar was successfully prepared by pyrolyzing corn stalks and pine sawdust with KHCO3 as a green activator. The resulting biochar, CBC-800, demonstrated more graphite defects and a higher SSA, which provide fast diffusion channels for the diffusion of PFOA, thereby promoting the adsorption of PFOA. The adsorption of PFOA on CBC-800 was fast and efficient, with the maximum adsorption capacity, as obtained by the Langmuir model, reaching 514.8 mg/g, which was advantageous over other adsorbents. The adsorption kinetic and isotherm data were better fitted by the pseudo-second-order kinetics and Freundlich models, respectively, indicating that chemisorption was the rate-limiting stage in the adsorption process. Thermodynamic results demonstrated that the adsorption process was both physically spontaneous and exothermic. CBC-800 demonstrated a high adsorption capacity for PFOA across a wide pH range and in the presence of various ions. Furthermore, the removal rate of low concentrations experiments demonstrated that CBC-800 had excellent consecutive adsorption performance at low PFOA concentrations. The adsorption mechanism study provided that pore filling was identified as the dominant adsorption mechanism. Given the superior adsorption capacity and low environmental impact of CBC-800, KHCO3-modified biochar has a promising potential application in practical water-contaminated environments and wastewater treatment processes to remove PFOA and contribute to environmental protection.
DECLARATIONS
Authors’ contributions
Formal analysis, investigation, conceptualization, data curation, writing - original draft, visualization: Hu, T.
Conceptualization, writing - review and editing, formal analysis: Wu, J. Q.
Methodology, formal analysis, data curation: Wang, T. S.
Resources, formal analysis: Li, S. J.
Resources, conceptualization, writing-review and editing, supervision, project administration, funding acquisition: Chen, J. W.
Availability of data and materials
The raw data supporting the findings of this study are available within this Article and its Supplementary Materials. Further data are available from the corresponding author upon reasonable request.
Financial support and sponsorship
This study was supported by the National Natural Science Foundation of China (41731282).
Conflicts of interest
Chen, J. W. is the Guest Editor of the Special Issue “Research Advances in the Control and Removal Strategies for PFAS Contaminants” and an Editorial Board member of the journal Water Emerging Contaminants & Nanoplastics. Chen, J. W. was not involved in any steps of editorial processing, notably including reviewer selection, manuscript handling, and decision making. The other authors declared that there are no conflicts of interest.
Ethical approval and consent to participate
Not applicable.
Consent for publication
Not applicable.
Copyright
© The Author(s) 2025.
Supplementary Materials
REFERENCES
1. Kucharzyk, K. H.; Darlington, R.; Benotti, M.; Deeb, R.; Hawley, E. Novel treatment technologies for PFAS compounds: a critical review. J. Environ. Manage. 2017, 204, 757-64.
2. Wang, J.; Cao, C.; Wang, Y.; Wang, Y.; Sun, B.; Zhu, L. In situ preparation of p-n BiOI@Bi5O7I heterojunction for enhanced PFOA photocatalytic degradation under simulated solar light irradiation. Chem. Eng. J. 2020, 391, 123530.
3. Lang, J. R.; Allred, B. M.; Field, J. A.; Levis, J. W.; Barlaz, M. A. National estimate of per- and polyfluoroalkyl substance (PFAS) release to U.S. municipal landfill leachate. Environ. Sci. Technol. 2017, 51, 2197-205.
4. Evich, M. G.; Davis, M. J. B.; McCord, J. P.; et al. Per- and polyfluoroalkyl substances in the environment. Science 2022, 375, eabg9065.
5. Houtz, E. F.; Higgins, C. P.; Field, J. A.; Sedlak, D. L. Persistence of perfluoroalkyl acid precursors in AFFF-impacted groundwater and soil. Environ. Sci. Technol. 2013, 47, 8187-95.
6. Yu, H.; Chen, H.; Zhang, P.; et al. In situ self-sacrificial synthesis of polypyrrole/biochar composites for efficiently removing short- and long-chain perfluoroalkyl acid from contaminated water. J. Environ. Manage. 2023, 344, 118745.
7. Tian, D.; Geng, D.; Tyler, M. e. h. l. e. r. . W.; et al. Removal of perfluorooctanoic acid (PFOA) from aqueous solution by amino-functionalized graphene oxide (AGO) aerogels: Influencing factors, kinetics, isotherms, and thermodynamic studies. Sci. Total. Environ. 2021, 783, 147041.
8. Zeng, Z.; Song, B.; Xiao, R.; et al. Assessing the human health risks of perfluorooctane sulfonate by in vivo and in vitro studies. Environ. Int. 2019, 126, 598-610.
9. Chen, Z.; Liu, Y.; Wei, W.; Ni, B. Recent advances in electrocatalysts for halogenated organic pollutant degradation. Environ. Sci. Nano. 2019, 6, 2332-66.
10. Luo, P.; Zhang, Y.; Peng, Z.; et al. Photocatalytic degradation of perfluorooctanoic acid (PFOA) from water: a mini review. Environ. Pollut. 2024, 343, 123212.
11. Singh, M.; Ahsan, M.; Pandey, V.; et al. Comparative assessment for removal of anionic dye from water by different waste-derived biochar vis a vis reusability of generated sludge. Biochar 2022, 4, 140.
12. Deng, S.; Chen, J.; Chang, J. Application of biochar as an innovative substrate in constructed wetlands/biofilters for wastewater treatment: performance and ecological benefits. J. Clean. Prod. 2021, 293, 126156.
13. Zhang, X.; Tian, J.; Wang, P.; et al. Highly-efficient nitrogen self-doped biochar for versatile dyes’ removal prepared from soybean cake via a simple dual-templating approach and associated thermodynamics. J. Clean. Prod. 2022, 332, 130069.
14. Zhang, J.; Chen, Z.; Liu, Y.; Wei, W.; Ni, B. Removal of emerging contaminants (ECs) from aqueous solutions by modified biochar: a review. Chem. Eng. J. 2024, 479, 147615.
15. He, J.; Gomeniuc, A.; Olshansky, Y.; et al. Enhanced removal of per- and polyfluoroalkyl substances by crosslinked polyaniline polymers. Chem. Eng. J. 2022, 446, 137246.
16. Yu, H.; Zhang, P.; Chen, H.; et al. Porous polypyrrole with a vesicle-like structure for efficient removal of per- and polyfluoroalkyl substances from water: crucial role of porosity and morphology. J. Hazard. Mater. 2024, 462, 132748.
17. Fagbayigbo, B. O.; Opeolu, B. O.; Fatoki, O. S.; Akenga, T. A.; Olatunji, O. S. Removal of PFOA and PFOS from aqueous solutions using activated carbon produced from Vitis vinifera leaf litter. Environ. Sci. Pollut. Res. Int. 2017, 24, 13107-20.
18. Deng, S.; Nie, Y.; Du, Z.; et al. Enhanced adsorption of perfluorooctane sulfonate and perfluorooctanoate by bamboo-derived granular activated carbon. J. Hazard. Mater. 2015, 282, 150-7.
19. Cheng, H.; Bian, Y.; Wang, F.; et al. Green conversion of crop residues into porous carbons and their application to efficiently remove polycyclic aromatic hydrocarbons from water: sorption kinetics, isotherms and mechanism. Bioresour. Technol. 2019, 284, 1-8.
20. Wang, W.; Lin, J.; Shao, S.; Chen, H.; Dai, J.; Yang, Y. Enhanced adsorption of benzo(a)pyrene in soil by porous biochar: adsorption kinetics, thermodynamics, and mechanisms. J. Environ. Chem. Eng. 2023, 11, 109002.
21. Ma, P.; Yao, S.; Wang, Z.; Qi, F.; Liu, X. Preparation of nitrogen-doped hierarchical porous carbon aerogels from agricultural wastes for efficient pollution adsorption. Sep. Purif. Technol. 2023, 311, 123250.
22. Jing, F.; Guan, J.; Tang, W.; Chen, J. Mechanistic insight into adsorptive removal of ionic NOR and nonionic DEP organic contaminates by clay-biochar composites. Environ. Pollut. 2022, 310, 119881.
23. Wang, T.; Wu, J.; Hu, T.; et al. Mechanistic insights into adsorption-desorption of PFOA on biochars: effects of biomass feedstock and pyrolysis temperature, and implication of desorption hysteresis. Sci. Total. Environ. 2024, 957, 177668.
24. Wang, K.; Wang, Y.; Zhang, S.; Chen, Y. D.; Wang, R.; Ho, S. H. Tailoring a novel hierarchical cheese-like porous biochar from algae residue to boost sulfathiazole removal. Environ. Sci. Ecotechnol. 2022, 10, 100168.
25. Ouyang, T.; Zhang, T.; Wang, H.; et al. High-throughput fabrication of porous carbon by chemical foaming strategy for high performance supercapacitor. Chem. Eng. J. 2018, 352, 459-68.
26. Asadullah, M.; Asaduzzaman, M.; Kabir, M. S.; Mostofa, M. G.; Miyazawa, T. Chemical and structural evaluation of activated carbon prepared from jute sticks for Brilliant Green dye removal from aqueous solution. J. Hazard. Mater. 2010, 174, 437-43.
27. Li, X.; Huang, Y.; Liang, X.; et al. Characterization of biochars from woody agricultural wastes and sorption behavior comparison of cadmium and atrazine. Biochar 2022, 4, 132.
28. Zhu, H.; Liu, X.; Jiang, Y.; Lin, D.; Yang, K. Sorption kinetics of 1,3,5-trinitrobenzene to biochars produced at various temperatures. Biochar 2022, 4, 157.
29. Wu, J.; Wang, T.; Liu, Y.; Tang, W.; Geng, S.; Chen, J. Norfloxacin adsorption and subsequent degradation on ball-milling tailored N-doped biochar. Chemosphere 2022, 303, 135264.
30. Tang, W.; Jing, F.; Laurent, Z. B. L. G.; Liu, Y.; Chen, J. High-temperature and freeze-thaw aged biochar impacts on sulfonamide sorption and mobility in soil. Chemosphere 2021, 276, 130106.
31. Xu, Q.; Liu, T.; Li, L.; et al. Hydrothermal carbonization of distillers grains with clay minerals for enhanced adsorption of phosphate and methylene blue. Bioresour. Technol. 2021, 340, 125725.
32. Yang, Y.; Luo, X.; Zhang, J.; Ma, X.; Sun, P.; Zhao, L. Sewage sludge–coconut fiber co-pyrolysis biochar: mechanisms underlying synergistic heavy metal stabilization and ciprofloxacin adsorption. J. Clean. Prod. 2022, 375, 134149.
33. Kan, T.; Strezov, V.; Evans, T.; et al. Catalytic pyrolysis of biomass impregnated with elements from steelmaking slag leaching and simultaneous fabrication of phosphorus adsorbent. J. Clean. Prod. 2021, 328, 129490.
34. Lei, X.; Yao, L.; Lian, Q.; et al. Enhanced adsorption of perfluorooctanoate (PFOA) onto low oxygen content ordered mesoporous carbon (OMC): adsorption behaviors and mechanisms. J. Hazard. Mater. 2022, 421, 126810.
35. Li, Y.; Shang, H.; Cao, Y.; Yang, C.; Feng, Y.; Yu, Y. High performance removal of sulfamethoxazole using large specific area of biochar derived from corncob xylose residue. Biochar 2022, 4, 128.
36. Velusamy, K.; Periyasamy, S.; Kumar, P. S.; et al. Analysis on the removal of emerging contaminant from aqueous solution using biochar derived from soap nut seeds. Environ. Pollut. 2021, 287, 117632.
37. Wang, B.; Jiang, Y. S.; Li, F. Y.; Yang, D. Y. Preparation of biochar by simultaneous carbonization, magnetization and activation for norfloxacin removal in water. Bioresour. Technol. 2017, 233, 159-65.
38. Ma, Y.; Li, M.; Li, P.; et al. Hydrothermal synthesis of magnetic sludge biochar for tetracycline and ciprofloxacin adsorptive removal. Bioresour. Technol. 2021, 319, 124199.
39. Heo, J.; Yoon, Y.; Lee, G.; Kim, Y.; Han, J.; Park, C. M. Enhanced adsorption of bisphenol A and sulfamethoxazole by a novel magnetic CuZnFe2O4-biochar composite. Bioresour. Technol. 2019, 281, 179-87.
40. Wang, G.; Yong, X.; Luo, L.; Yan, S.; Wong, J. W.; Zhou, J. Structure-performance correlation of high surface area and hierarchical porous biochars as chloramphenicol adsorbents. Sep. Purif. Technol. 2022, 296, 121374.
41. Yea, Y.; Kim, G.; Wang, D.; et al. Selective sequestration of perfluorinated compounds using polyaniline decorated activated biochar. Chem. Eng. J. 2022, 430, 132837.
42. Qian, J.; Martinez, A.; Marek, R. F.; et al. Polymeric nanofiber-carbon nanotube composite mats as fast-equilibrium passive samplers for polar organic contaminants. Environ. Sci. Technol. 2020, 54, 6703-12.
43. Liu, Z.; Zhang, P.; Wei, Z.; et al. Porous Fe-doped graphitized biochar: an innovative approach for co-removing per-/polyfluoroalkyl substances with different chain lengths from natural waters and wastewater. Chem. Eng. J. 2023, 476, 146888.
44. Lei, X.; Lian, Q.; Zhang, X.; et al. Removal of perfluorooctanoic acid via polyethyleneimine modified graphene oxide: effects of water matrices and understanding mechanisms. Chemosphere 2022, 308, 136379.
45. Sekulic M, Boskovic N, Milanovic M, Grujic Letic N, Gligoric E, Pap S. An insight into the adsorption of three emerging pharmaceutical contaminants on multifunctional carbonous adsorbent: mechanisms, modelling and metal coadsorption. J. Mol. Liq. 2019, 284, 372-82.
46. Heidari, H.; Abbas, T.; Ok, Y. S.; Tsang, D. C. W.; Bhatnagar, A.; Khan, E. GenX is not always a better fluorinated organic compound than PFOA: a critical review on aqueous phase treatability by adsorption and its associated cost. Water. Res. 2021, 205, 117683.
47. Fang, J.; Xu, K.; Liu, A.; et al. Selective perfluorooctanoic acid (PFOA) and perfluorooctane sulfonate (PFOS) adsorption by nanoscale zero-valent iron (nZVI): performance and mechanisms. Environ. Sci. Nano. 2024, 11, 1915-25.
48. Wang, M.; Orr, A. A.; Jakubowski, J. M.; et al. Enhanced adsorption of per- and polyfluoroalkyl substances (PFAS) by edible, nutrient-amended montmorillonite clays. Water. Res. 2021, 188, 116534.
Cite This Article

How to Cite
Download Citation
Export Citation File:
Type of Import
Tips on Downloading Citation
Citation Manager File Format
Type of Import
Direct Import: When the Direct Import option is selected (the default state), a dialogue box will give you the option to Save or Open the downloaded citation data. Choosing Open will either launch your citation manager or give you a choice of applications with which to use the metadata. The Save option saves the file locally for later use.
Indirect Import: When the Indirect Import option is selected, the metadata is displayed and may be copied and pasted as needed.
About This Article
Special Issue
Copyright
Data & Comments
Data
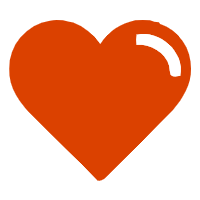
Comments
Comments must be written in English. Spam, offensive content, impersonation, and private information will not be permitted. If any comment is reported and identified as inappropriate content by OAE staff, the comment will be removed without notice. If you have any queries or need any help, please contact us at [email protected].