Unity quantum yield of InP/ZnSe/ZnS quantum dots enabled by Zn halide-derived hybrid shelling approach
Abstract
Environment-benign indium phosphide (InP) quantum dots (QDs) show great promise as visible emitters for next-generation display applications, where bright and narrow emissivity of QDs should be required toward high-efficiency, high-color reproducibility. The photoluminescence (PL) performance of InP QDs has been consistently, markedly improved, particularly owing to the exquisite synthetic control over core size homogeneity and core/shell heterostructural variation. To date, synthesis of most high-quality InP QDs has been implemented by using zinc (Zn) carboxylate as a shell precursor that unavoidably entails the formation of surface oxide on InP core. Herein, we demonstrate synthesis of superbly bright, color-pure green InP/ZnSe/ZnS QDs by exploring an innovative hybrid Zn shelling approach, where Zn halide (ZnX2, X = Cl, Br, I) and Zn oleate are co-used as shell precursors. In the hybrid Zn shelling process, the type of ZnX2 is found to affect the growth outcomes of ZnSe inner shell and consequent optical properties of the resulting heterostructured InP QDs. Enabled by not only the near-complete removal of the oxide layer on InP core surface through the hybrid Zn shelling process but the controlled growth rate of ZnSe inner shell, green InP/ZnSe/ZnS QDs achieve a record quantum yield (QY) up to unity along with a highly sharp linewidth of 32 nm upon growth of an optimal ZnSe shell thickness. This work affords an effective means to synthesize high-quality heterostructured InP QDs with superb emissive properties.
Keywords
INTRODUCTION
Today, colloidal quantum dots (QDs) are scientifically and industrially important nanomaterials particularly as visible emitters for post-organic light-emitting diode next-generation displays[1-4]. Besides, high compatibility of QDs with solution processing and for inorganic-organic hybridization makes them prospective candidates for flexible/stretchable display devices[5,6]. To meet the high standards of state-of-the-art display devices that require high efficiency and color gamut, bright and narrow emissivity of QDs must be achieved. Pb-containing perovskite- and CdSe-based QDs with excellent photoluminescence (PL) quantum yield (QY) (> 90%) and sharp linewidth [full-width-at-half-maximum (FWHM)] (< 20-30 nm) are considered ideal light emitters[2,7-11], but their use is strictly limited to the commercial display panels due to the environmental regulations on toxic heavy metal elements[12,13]. In this regard, visible-emissive indium phosphide (InP)-based QDs with an environmental benignity have emerged as the most promising alternative[14-18]. A primary factor that limits the optical properties of InP QDs is the incomplete surface passivation, by which unpassivated surface dangling bonds serve as the trap sites for photoexcited carriers, detrimental to the radiative recombination. In heterovalent core/shell structure of InP QDs, ZnS has been initially chosen as a shell due to its wide band gap which enables the effective confinement of charge carriers within the InP core domain[19-22]. However, PL performance of the resulting InP/ZnS QDs was often unsatisfactory mostly thanks to the considerable interfacial strain developed by a sizable InP-ZnS lattice mismatch. Placement of better lattice-matched shells, such as GaP[23-25], ZnSe[14,26], and ZnSeS[27-29], between InP and ZnS led to significant enhancements of PL figures-of-merit (i.e., QY and FWHM). The state-of-the-art heterostructured InP QDs for green and red colors displayed PL QYs of ≥ 95% and FWHMs of ca.
In a typical synthesis of InP QDs, the carboxylic acid used to generate indium carboxylate produces water through a ketonization reaction at high temperatures. This water, in turn, induces the unwanted formation of a surface oxidation product on as-synthesized InP core[34,35], which can hamper not only full passivation of core surface but uniform growth of epitaxial shell. For an effort to etch out such surface oxide species, hydrofluoric acid (HF) was directly introduced[32] or indirectly in-situ generated via the reaction between ZnF2 and carboxylic acid[36] in synthesis of “red-emissive” InP/ZnSe/ZnS QDs, resulting in PL QYs of 90%-100% and FWHMs of 35-36 nm. Although the HF treatment is a useful means for the effective removal of the surface oxide layer on InP core, its highly corrosive, toxic character may become a stumbling block for eco-friendly, sustainable mass production in the future. Thus, an alternative safe synthetic method should be unearthed. Moreover, judging from no noteworthy report on the efficacy of HF treatment on “green-emissive” InP QDs, it is highly likely that HF treatment works well only to a relatively large-sized (i.e., red-emissive) core, even though its cause appears still ambiguous.
In this contribution, a new and innovative means toward synthesis of green InP/ZnSe/ZnS QDs with a unity PL QY together with a sharp emissivity is developed. The key to synthetic success involves adding zinc oleate [Zn(OA)2] to the existing zinc halide (ZnX2)-based shell growth process. We first explore the effects of different shell precursors of Zn(OA)2 and ZnX2 (X = Cl, Br, I) on growth outcomes and optical properties of the resulting heterostructured InP QDs. InP/ZnSe/ZnS QDs shelled with oxygen-free Zn precursors of ZnX2 exhibit not only higher PL QYs (75%-89%, depending on the type of ZnX2) but more homogeneous particle morphologies compared to those shelled with a common Zn precursor of Zn(OA)2. The subsequent hybrid shelling approach, where co-use of Zn(OA)2 and ZnX2 synergically leads to the effective removal of surface oxide species on InP core, further enables synthesis of green InP QDs having PL QYs of up to 100% along with a narrow FWHM of 32 nm upon adopting an appropriate halide precursor of ZnBr2 and reaching an optimal ZnSe inner shell thickness.
EXPERIMENTAL
Synthesis of InP core QDs
Synthesis of green-emissive InP cores was conducted by mixing 0.15 mmol of indium (In) acetate,
Double shelling of InP core with ZnSe/ZnS based on Zn oleate or Zn halides
For Zn oleate [Zn(OA)2]-based shelling, 5.0 mmol of Zn acetate, 3 mL of ODE, and 10 mmol of oleic acid (OA), and 1 mL of InP core dispersion (in toluene) were loaded in a 50 mL flask. After the toluene was fully removed through evacuation at 120 °C for 30 min, the mixture was heated to 160 °C. For ZnSe inner shelling, Se-TOP solution [1.0 mmol of selenium (Se) dissolved in 1 mL of TOP] was rapidly introduced, followed by the reaction at 300 °C for 60 min. For a sequential growth of ZnS outer shell, S-TOP solution (0.5 mmol of S dissolved in 0.5 mL of TOP) was injected, followed by the reaction at 300 °C for 60 min. The whole growth reaction was finalized by adding 0.75 mL of 1-octanethiol (OTT) and reacting at 230 °C for
Double shelling of InP core with ZnSe/ZnS based on Zn halide-derived hybrid approach
First, 5.0 mmol of Zn halide (ZnCl2, ZnBr2, or ZnI2), 3 mL of ODE, 3 mL of OLA, and 1 mL of InP core dispersion were loaded in a flask, followed by the same procedures as above prior to ZnSe inner shelling. For ZnSe inner shelling, Se-TOP solution (1.0 mmol of Se dissolved in 1 mL of TOP) and Zn stock solution (2.8 mmol of Zn acetate dissolved in 0.88 mL of OA and 8 mL of ODE) were rapidly injected together, followed by the reaction at 300 °C for 60 min. Then, ZnS outer shelling was conducted by injecting S-TOP solution (0.5 mmol of S dissolved in 0.5 mL of TOP) and the Zn stock solution (0.7 mmol of Zn acetate dissolved in 0.44 mL of OA and 2 mL of ODE) and reacting at 300 °C for 60 min. The subsequent procedures were the same as above. The overall synthetic procedures are illustrated in Scheme 1.
Characterization
Absorption and PL spectra of InP QDs dispersed in hexane were measured by using ultraviolet (UV)-visible spectroscopy (Shimadzu, UV-2450) and a 500 W Xe lamp-equipped spectrophotometer (PSI Co. Ltd., Darsa Pro-5200), respectively. PL QY of QDs was assessed in an integrating hemisphere with an absolute PL QY measurement system (Otsuka, QE-2000). PL decay profile of QDs in hexane dispersion was recorded using the time-correlated single-photon counting method on a spectrophotometer (Edinburgh Instruments, FS5) equipped with a picosecond pulsed laser diode (EPL-375). High-resolution transmission electron microscopy (TEM) images of QDs were obtained with a JEM-2100F (JEOL Ltd). X-ray photoelectron spectroscopic (XPS) (Thermo Scientific Inc., K-Alpha) analysis on QDs was performed to identify their surface chemical composition. Powder X-ray diffraction (XRD) with Cu Ka radiation (Rigaku, Ultima IV) was used to additionally provide information on size variation of a series of heterostructured InP QDs. 31P solid-state nuclear magnetic resonance (NMR) experiments were recorded on a spectrometer (Bruker, AVANCE II+ 400 MHz NMR system). The samples were packed into 3.2 mm zirconia rotors. The rotors were spun 10 kHz at room temperature. 31P solid-state NMR was performed with a recycle delay of 100 s. 31P chemical shifts were referenced to an external 85% H3PO4 sample. Raman spectral analysis was conducted utilizing a Raman imaging microscope (Thermo Scientific Inc., DXR2xi). The samples were prepared by drop-casting the QDs solution on a silicon substrate and drying in ambient condition. All samples were excited at a wavelength of 455 nm employing a laser diode. The resultant scattering signals were captured via an electron-multiplying charge-coupled device (CCD).
RESULTS AND DISCUSSION
The present green-emissive InP cores were synthesized by heating up a mixture of (TMS)3P, In acetate, Zn acetate, palmitic acid, and ODE to 240 °C, where the formed Zn carboxylate plays a role in regulating the reactivity of indium precursor with (TMS)3P via Zn-P coupling toward narrow size distribution[37-39]. The resulting InP cores displayed a well-defined absorption feature with the first excitonic peak at 440 nm along with a high valley depth (VD) of 0.4 [Supplementary Figure 1A]. The VD value, defined as the ratio of absorption between the first maximum and the minimum inflection point, is a convenient measure for assessing the size distribution particularly for binary QDs, as their band gap (Eg) variation is derived exclusively from the degree of size distribution with the compositional deviation excluded. Nemoto et al. reported an even higher VD of 0.51 from green-emissive InP cores[40], indicating there is still room for synthetic optimization of our InP cores toward higher size homogeneity. The size of InP core required to secure green emissions of ca. 525-535 nm in PL peak wavelength typically ranges in 2-2.5 nm (corresponding to the first excitonic absorption peaks at ca. 430-460 nm), although the ultimate PL wavelength nontrivially varies with details of core/shell heterostructure including shell chemical composition and thickness. As seen in a TEM image of InP cores [Supplementary Figure 1B], the precise size determination of green-emissive InP core is highly challenging from direct microscopic measurement due to its extremely tiny size. Such size uncertainty is often tackled with the correlation between Eg versus diameter (d) (i.e., sizing curve) such as Eg = 1.401 + 3.493/d1.172 for InP cores[41], by which the size of the present InP cores was estimated to be 2.0 nm.
InP cores were placed in a two-step shelling process, which rules out the gradual incorporation of unreacted species (yielding the non-radiative recombination channels) into the subsequent heteroepitaxial shell[42]. Distinct from the existing shelling protocol, where Zn(OA)2 is exclusively used for ZnSe inner and ZnS outer shell growth, we uniquely adopted Zn halide precursors of ZnX2 (X = Cl, Br, I). For convenience’s sake, we denoted InP QDs shelled with different types of Zn precursors as InP-ZnX2 and InP-Zn(OA)2. Figure 1A presents the Zn precursor-dependent variations of PL QYs and peak wavelengths of green InP/ZnSe/ZnS QDs. InP-ZnX2/ZnSe/ZnS QDs exhibited overall higher PL QYs (specifically, 75%-89%, depending on the type of Zn halide) compared to InP-Zn(OA)2/ZnSe/ZnS ones (71%), while use of ZnBr2 yielded the peak value of 89%. As compared in their absorption spectra normalized at the 1S peak [Figure 1B], absorbance of InP-ZnX2/ZnSe/ZnS QDs in blue-to-near UV region increased in the order of ZnI2 < ZnBr2 < ZnCl2, while that of InP-Zn(OA)2/ZnSe/ZnS QDs was slightly lower relative to InP-ZnBr2/ZnSe/ZnS ones. Such a disparity in absorption is directly correlated with the thickness (or volume) of the ZnSe shell[30,43], indicative of the formation of the thickest and thinnest ZnSe shell for InP-ZnCl2/ZnSe/ZnS and InP-ZnI2/ZnSe/ZnS QDs, respectively. Being in line with the above absorption trend, the average sizes of a series of InP-ZnX2/ZnSe/ZnS QDs systematically decreased from 10.2 [Figure 1C], 6.7 [Figure 1D] to 5.3 nm [Figure 1E] for X = Cl, Br, I, respectively, while that of InP-Zn(OA)2/ZnSe/ZnS ones was 6.0 nm [Figure 1F] (refer to Supplementary Figure 2 for the respective size distribution histograms). Note that InP-ZnX2/ZnSe/ZnS QDs overall possessed more uniform, spherical particle morphologies compared to InP-Zn(OA)2/ZnSe/ZnS counterparts. Use of Zn carboxylate [e.g., Zn(OA)2] as a shell precursor is well-known to entail the generation of surface InPOx during shell growth (as will be discussed later in more detail), which, in turn, renders the homogeneous growth of subsequent ZnSe shell difficult. In the case of InP/ZnSe QD heterostructure, the thickness of ZnSe shell influences the band gap (consequently, PL) primarily owing to the delocalization of electron wavefunction across the shell (which is more predominant in green-emissive core due to a relatively small conduction band offset compared to red-emissive counterpart), leading to the reduction in quantum confinement effects and consequently band gap from a thicker ZnSe shell (refer to Supplementary Figure 3A magnified from Figure 1B). On that account, as presented in normalized PL spectra of the above series of InP/ZnSe/ZnS QDs [Supplementary Figure 3B], PL peak wavelength was well proportional to ZnSe shell thickness (e.g., the longest and shortest wavelengths of 538 and 530 nm from ZnCl2- and ZnI2-based InP/ZnSe/ZnS QDs, respectively). Aligning with the above results, the time-resolved PL decay profiles of the same set of InP/ZnSe/ZnS QDs showed a slower decay behavior with a thicker ZnSe shell [Supplementary Figure 3C]. We will further discuss different ZnSe shell thicknesses produced as a function of equimolar ZnX2 later.
Figure 1. (A) Variations of PL QY and peak wavelength; (B) absorption spectra; and (C-F) TEM images of green InP/ZnSe/ZnS QDs synthesized with different Zn precursor types of ZnX2 (X = Cl, Br, I) and Zn(OA)2 for growth of ZnSe inner and ZnS outer shell. The double shell thicknesses of ZnSe/ZnS for individual QDs are also depicted in (C-F). The scale bars in (C-F) are all 20 nm. PL: Photoluminescence; QY: quantum yield; TEM: transmission electron microscopy; InP: indium phosphide; QDs: quantum dots.
Surface of highly oxyphilic InP is susceptible to the oxidation. Under the synthetic environment of InP QDs in the presence of carboxylic acids, the surface oxide species (e.g., InPOx) becomes easily formed by water released via the ketonization reaction (R1COOH + R2COOH → R1COR2 + CO2 + H2O) during both core growth and subsequent shelling stage at elevated temperatures[32,36,44]. Formation of such surface oxide is well verified in the pristine InP and Zn(OA)2-reacted InP core [InP-Zn(OA)2] samples by an XPS analysis [Figure 2A]. The P 2p spectrum clearly shows the presence of two chemical environments for P atoms, consisting of the strong peak at 127.6-129.8 eV for InP and the weak peak at 131.7-134.1 eV for InPOx. The latter peak was intensified upon an additional reaction of InP core with Zn(OA)2, indicative of further promotion of InPOx formation. Meanwhile, ZnX2-reacted InP cores (InP-ZnX2) did not exhibit a notable change in XPS P spectrum regardless of halide type [Figure 2B] compared to pristine InP core due to the oxygen-free Zn halide sources, while the surface oxide formed during core growth still remained. For an effort to remove the remnant surface InPO4, we introduced a hybrid Zn process, where the growth of ZnSe shell proceeds under co-use of ZnX2 and Zn(OA)2. Here, we denote InP core reacted with ZnX2 and
Figure 2. P 2p XPS spectra of (A) pristine InP and InP-Zn(OA)2 cores; (B) a series of InP-ZnX2 cores; and (C) a series of InP-ZnX2-Zn(OA)2 cores; (D) 31P NMR spectra of InP-Zn(OA)2 and a series of InP-ZnX2-Zn(OA)2 cores; Photographs of a set of (E) InP-ZnX2 and (F) InP-ZnX2-Zn(OA)2 cores under UV irradiation. XPS: X-ray photoelectron spectroscopic; InP: indium phosphide; NMR: nuclear magnetic resonance; UV: ultraviolet.
Leveraging the above hybrid Zn process, subsequent ZnSe shelling was implemented for InP-ZnX2-Zn(OA)2 by co-injecting the equimolar Se stock solution. Figure 3A-C presents TEM images of a set of InP/ZnSe QDs with use of ZnCl2, ZnBr2, and ZnI2, corresponding to the average sizes of 8.0, 5.6, and 5.1 nm, respectively. This size trend is in line with that from the previous InP-ZnX2/ZnSe/ZnS QDs [Figure 1C-E], manifesting that the thickness of ZnSe shell is sensitively dependent on the type of ZnX2 despite use of the identical molar quantity of ZnX2. In the case of synthesis of aminophosphine-derived InP cores, where InX3 as a cationic precursor is used in the presence of amines such as OLA, the size of the resulting cores tends to increase in the order of Cl > Br > I[52], which is analogous to the present ZnX2-dependent variation of ZnSe shell thickness. Based on the assumption of surface reaction-limited condition, ZnSe shell growth would be expected to be facilitated under the enhanced solute solubility and the increased rate constant of surface reaction[52]. For ZnX2, OLA, categorized as a hard base, may be able to produce the complexes by more strongly coordinating with ZnCl2 compared to ZnI2, indicative of a higher solute solubility of the former versus the latter. Meanwhile, given the adsorption of halide on InP core surface (as evidenced from Supplementary Figure 4), the least bulky surface halide of Cl- ions will render ZnSe shell growth easier, raising the rate of surface reaction. Disparity in ZnSe shell thickness as a function of ZnX2 is well reflected in absorption spectra normalized at the 1S peak [Figure 3D], where a greater absorbance in blue-to-near UV region was observed in the sequence of Cl > Br > I. Accordingly, PL systematically shifted with a thicker ZnSe shell from 531 (for ZnI2) to 539 nm (for ZnCl2) [Figure 3E], being related to the ZnSe shell thickness-dependent delocalization of electron wavefunction aforementioned. Even without ZnS outer shelling, PL QYs of a series of InP/ZnSe QDs were relatively high. In InP-ZnX2-Zn(OA)2/ZnSe QDs ZnBr2 yielded the highest PL QY of 93%, while ZnCl2 and ZnI2 gave comparable values of 83%-85% [Figure 3F]. As argued above, use of ZnCl2 leads to the fastest ZnSe shell growth, which stochastically renders controlled heteroepitaxial shell growth unlikely, thus leaving some imperfect sites at hetero-interface and/or shell interior. As an opposite case, the introduction of ZnI2 induces the slowest shell growth reaction entailing the formation of the thinnest ZnSe shell, which can often encounter the high probability of incomplete shell coverage on core surface. Besides, HI is known as an unstable chemical as it spontaneously oxidizes to molecular iodine (I2) during the reaction[53,54], implying that in removing the surface oxide, it may not serve as a perfect reagent (vs. HCl and HBr), leaving unetched InPOx residue. This scenario may be supported by a marginal XPS signal trace of InPOx from InP-ZnI2-Zn(OA)2 unlike other two samples [Figure 2C]. In this context, ZnBr2 is regarded as an optimal halide as it allows for not only the relevant ZnSe growth rate but the near-complete removal of surface oxide, synergically rendering the heteroepitaxial shell growth perfect. Meanwhile, InP-ZnX2-Zn(OA)2/ZnSe QDs with ZnCl2 and ZnBr2 exhibited nearly the same PL FWHM of 32 nm, while a markedly broad value of 37 nm resulted from InP-ZnI2-Zn(OA)2/ZnSe ones [Figure 3F]. Given the identical InP core, the major determinants of PL linewidth of InP/ZnSe QDs are the average thickness and thickness uniformity of ZnSe shell. As noticed from size distributions [Figure 3A-C], InP-ZnCl2-Zn(OA)2/ZnSe QDs possessed a relatively wide size distribution, i.e., lack of shell thickness uniformity over individual QDs (resulting from an uncontrollably fast shell growth rate), when compared to other two samples. Nevertheless, a sharp linewidth of InP-ZnCl2-Zn(OA)2/ZnSe QDs benefits from a sufficiently thick ZnSe, which, in turn, can mitigate the exciton-phonon coupling strength via the increasing delocalization of charge carriers[55]. A broad linewidth of InP-ZnI2-Zn(OA)2/ZnSe QDs is likely ascribable to the combined consequence of comparatively thin shell thickness and imperfect heteroepitaxial shell growth (aforementioned), both of which have unfavorable effects on the reduction of exciton-phonon coupling strength. Subsequent growth of the ZnS outer shell, for which the equimolar Zn(OA)2 and S-TOP were identically used, on a series of InP-ZnX2-Zn(OA)2/ZnSe heterostructures above further boosted PL QY to 89%-100%, depending on the type of ZnX2, while emission linewidths stayed the same [Figure 4]. Also refer to Supplementary Figure 5 for the reproducible results of unity PL QYs from the representative three InP-ZnBr2-Zn(OA)2/ZnSe/ZnS QD samples. Such superb emissive figures-of-merit possessing a narrow FWHM (32 nm) and a unity PL QY of InP-ZnBr2-Zn(OA)2/ZnSe/ZnS QDs are definitely record values among green-emissive InP ones to date. The average sizes of InP-ZnX2-Zn(OA)2/ZnSe/ZnS QDs were 9.2, 6.4, and 5.7 nm for ZnCl2, ZnBr2, and ZnI2, respectively (refer to Supplementary Figure 6 for the respective size distribution histograms and Supplementary Figure 7 for the corresponding XRD patterns, where the heteroepitaxial coherent reflection peaks became gradually broader from ZnCl2, ZnBr2 to ZnI2), and thus, the average ZnS shell thicknesses were estimated to be 0.6, 0.4, and 0.3 nm, respectively. The thickness trend in ZnS shell also aligns with that in ZnSe shell [Figure 3A-C], implying that the growth kinetics of ZnS shell depends on the type of halides remaining after ZnSe shelling in a similar manner to that of ZnSe shell.
Figure 3. (A-C) TEM images and size distribution histograms (insets); (D) absorption spectra normalized at the 1S peak; (E) normalized PL spectra; and (F) variations of FWHM and PL QY of a series of InP-ZnX2-Zn(OA)2/ZnSe QDs. TEM: Transmission electron microscopy; PL: photoluminescence; FWHM: full-width-at-half-maximum; QY: quantum yield; InP: indium phosphide; QDs: quantum dots.
Figure 4. Absorption, PL spectra, UV-irradiated fluorescent images (insets) and TEM images (scale bar: 20 nm) of a series of InP-ZnX2-Zn(OA)2/ZnSe/ZnS QDs, where ZnX2 is (A) ZnCl2, (B) ZnBr2, and (C) ZnI2. PL: Photoluminescence; UV: ultraviolet; TEM: transmission electron microscopy; InP: indium phosphide; QDs: quantum dots.
Lastly, based on the best-performing InP-ZnBr2-Zn(OA)2/ZnSe/ZnS QDs, the thickness of ZnSe inner shell was further modulated simply by proportionally varying the respective amounts of Se-TOP (specifically, 0.5, 1.0, and 1.5 mmol) and Zn(OA)2 (specifically, 1.4, 2.8, and 4.2 mmol) injected for ZnSe shelling and the resulting inner shell is denoted as thin-, medium-, and thick-ZnSe for convenience’s sake. Also note that the above InP-ZnBr2-Zn(OA)2/ZnSe/ZnS QDs in Figure 4B correspond to medium-ZnSe having a ZnSe shell thickness (tZnSe) of 1.8 nm. With increasing thickness of ZnSe inner shell, the sizes increased from 3.9 to
Figure 5. TEM images (scale bar: 20 nm) of InP-ZnBr2-Zn(OA)2/ZnSe/ZnS QDs having (A) thin- and (B) thick-ZnSe; (C) Absorption spectra; (D) time-resolved PL decay profiles; (E) variations of FWHM and PL QY of InP-ZnBr2-Zn(OA)2/ZnSe/ZnS QDs as a function of ZnSe shell thickness; (F) ZnSe shell thickness-dependent change of heterostructural strain of InP core and ZnSe shell of InP-ZnBr2-Zn(OA)2/ZnSe QDs. TEM: Transmission electron microscopy; InP: indium phosphide; QDs: quantum dots; PL: photoluminescence; FWHM: full-width-at-half-maximum; QY: quantum yield.
CONCLUSIONS
In green-emissive heterostructured InP QDs, the effects of the type of Zn precursor for shelling on shell growth outcomes and consequent optical properties were investigated. When Zn halides were used for shelling, the resulting InP/ZnSe/ZnS QDs overall possessed not only more uniform, spherical particle morphologies but higher PL QYs compared to those shelled with the conventional Zn precursor of
DECLARATIONS
Authors’ contributions
Made substantial contributions to the conception and design of the study: Yang H, Ryu CW, Jo DY
Performed data analysis and interpretation and wrote the manuscript: Jo DY, Kim HM, Ryu CW, Yang H
Provided administrative, technical, and material support: Park GM, Shin D, Kim Y, Kim YH
Availability of data and materials
Not applicable.
Financial support and sponsorship
This research was supported by the National Research Foundation of Korea (NRF) grant funded by the Korean government (MSIT) (RS-2024-00411892, 2020M3H4A3082656) and the Technology Innovation Program (20010737, 20016332) funded by the Ministry of Trade, Industry & Energy (MOTIE, Korea). This work was also supported by the 2023 Hongik University Research Fund.
Conflicts of interest
All authors declared that there are no conflicts of interest.
Ethical approval and consent to participate
Not applicable.
Consent for publication
Not applicable.
Copyright
© The Author(s) 2024.
Supplementary Materials
REFERENCES
1. Pietryga JM, Park YS, Lim J, et al. Spectroscopic and device aspects of nanocrystal quantum dots. Chem Rev 2016;116:10513-622.
2. Yang Z, Gao M, Wu W, et al. Recent advances in quantum dot-based light-emitting devices: challenges and possible solutions. Mater Today 2019;24:69-93.
3. Dai X, Deng Y, Peng X, Jin Y. Quantum-dot light-emitting diodes for large-area displays: towards the dawn of commercialization. Adv Mater 2017;29:1607022.
4. Han C, Yang H. Development of colloidal quantum dots for electrically driven light-emitting devices. J Korean Ceram Soc 2017;54:449-69.
5. Lin Q, Zhu Y, Wang Y, et al. Flexible quantum dot light-emitting device for emerging multifunctional and smart applications. Adv Mater 2023;35:e2210385.
6. Kim DC, Seung H, Yoo J, et al. Intrinsically stretchable quantum dot light-emitting diodes. Nat Electron 2024;7:365-74.
8. Mashford BS, Stevenson M, Popovic Z, et al. High-efficiency quantum-dot light-emitting devices with enhanced charge injection. Nature Photon 2013;7:407-12.
9. Lu M, Zhang Y, Wang S, Guo J, Yu WW, Rogach AL. Metal halide perovskite light-emitting devices: promising technology for next-generation displays. Adv Funct Mater 2019;29:1902008.
10. Chen O, Zhao J, Chauhan VP, et al. Compact high-quality CdSe-CdS core-shell nanocrystals with narrow emission linewidths and suppressed blinking. Nat Mater 2013;12:445-51.
11. Hu S, Shabani F, Liu B, et al. High-performance deep red colloidal quantum well light-emitting diodes enabled by the understanding of charge dynamics. ACS Nano 2022;16:10840-51.
12. Yang SJ, Oh JH, Kim S, Yang H, Do YR. Realization of InP/ZnS quantum dots for green, amber and red down-converted LEDs and their color-tunable, four-package white LEDs. J Mater Chem C 2015;3:3582-91.
13. Liu P, Lou Y, Ding S, et al. Green InP/ZnSeS/ZnS core multi-shelled quantum dots synthesized with aminophosphine for effective display applications. Adv Funct Mater 2021;31:2008453.
14. Ramasamy P, Kim N, Kang Y, Ramirez O, Lee J. Tunable, bright, and narrow-band luminescence from colloidal indium phosphide quantum dots. Chem Mater 2017;29:6893-9.
15. Cao F, Wang S, Wang F, Wu Q, Zhao D, Yang X. A layer-by-layer growth strategy for large-size InP/ZnSe/ZnS core–shell quantum dots enabling high-efficiency light-emitting diodes. Chem Mater 2018;30:8002-7.
16. Tamang S, Lincheneau C, Hermans Y, Jeong S, Reiss P. Chemistry of InP nanocrystal syntheses. Chem Mater 2016;28:2491-506.
17. Song W, Lee S, Yang H. Fabrication of warm, high CRI white LED using non-cadmium quantum dots. Opt Mater Express 2013;3:1468-73.
18. Kim K, Jo J, Jo D, et al. Cation-exchange-derived InGaP alloy quantum dots toward blue emissivity. Chem Mater 2020;32:3537-44.
19. Lad AD, Mahamuni S. Effect of ZnS shell formation on the confined energy levels of ZnSe quantum dots. Phys Rev B 2008;78:125421.
20. Li Z, Wei J, Wang F, et al. Carrier dynamics in alloyed chalcogenide quantum dots and their light-emitting devices. Adv Energy Mater 2021;11:2101693.
21. Li L, Reiss P. One-pot synthesis of highly luminescent InP/ZnS nanocrystals without precursor injection. J Am Chem Soc 2008;130:11588-9.
22. Ryu E, Kim S, Jang E, et al. Step-wise synthesis of InP/ZnS core−shell quantum dots and the role of zinc acetate. Chem Mater 2009;21:573-5.
23. Kim S, Kim T, Kang M, et al. Highly luminescent InP/GaP/ZnS nanocrystals and their application to white light-emitting diodes. J Am Chem Soc 2012;134:3804-9.
24. Park JP, Lee JJ, Kim SW. Highly luminescent InP/GaP/ZnS QDs emitting in the entire color range via a heating up process. Sci Rep 2016;6:30094.
25. Xu Y, Lv Y, Wu R, et al. Preparation of highly stable and photoluminescent cadmium-free InP/GaP/ZnS core/shell quantum dots and application to quantitative immunoassay. Part Part Syst Char 2020;37:1900441.
26. Li Y, Hou X, Dai X, et al. Stoichiometry-controlled InP-based quantum dots: synthesis, photoluminescence, and electroluminescence. J Am Chem Soc 2019;141:6448-52.
27. Lim J, Bae WK, Lee D, et al. InP@ZnSeS, core@composition gradient shell quantum dots with enhanced stability. Chem Mater 2011;23:4459-63.
28. Hahm D, Chang JH, Jeong BG, et al. Design principle for bright, robust, and color-pure InP/ZnSexS1-x/ZnS heterostructures. Chem Mater 2019;31:3476-84.
29. Jo J, Jo D, Lee S, et al. InP-based quantum dots having an InP core, composition-gradient ZnSeS inner shell, and ZnS outer shell with sharp, bright emissivity, and blue absorptivity for display devices. ACS Appl Nano Mater 2020;3:1972-80.
30. Lee SH, Kim Y, Jang H, et al. The effects of discrete and gradient mid-shell structures on the photoluminescence of single InP quantum dots. Nanoscale 2019;11:23251-8.
31. Kim Y, Ham S, Jang H, et al. Bright and uniform green light emitting InP/ZnSe/ZnS quantum dots for wide color gamut displays. ACS Appl Nano Mater 2019;2:1496-504.
32. Won YH, Cho O, Kim T, et al. Highly efficient and stable InP/ZnSe/ZnS quantum dot light-emitting diodes. Nature 2019;575:634-8.
33. Cui J, Beyler AP, Marshall LF, et al. Direct probe of spectral inhomogeneity reveals synthetic tunability of single-nanocrystal spectral linewidths. Nat Chem 2013;5:602-6.
34. Cros-Gagneux A, Delpech F, Nayral C, Cornejo A, Coppel Y, Chaudret B. Surface chemistry of InP quantum dots: a comprehensive study. J Am Chem Soc 2010;132:18147-57.
35. Choi S, Kim H, Yoon S, et al. Aminophosphine-derived, high-quality red-emissive InP quantum dots by the use of an unconventional in halide. J Mater Chem C 2022;10:2213-22.
36. Li H, Zhang W, Bian Y, Ahn TK, Shen H, Ji B. ZnF2-assisted synthesis of highly luminescent InP/ZnSe/ZnS quantum dots for efficient and stable electroluminescence. Nano Lett 2022;22:4067-73.
37. Xi L, Cho DY, Besmehn A, et al. Effect of zinc incorporation on the performance of red light emitting InP core nanocrystals. Inorg Chem 2016;55:8381-6.
38. Koh S, Eom T, Kim WD, et al. Zinc–phosphorus complex working as an atomic valve for colloidal growth of monodisperse indium phosphide quantum dots. Chem Mater 2017;29:6346-55.
39. Kim K, Suh Y, Kim D, et al. Zinc oxo clusters improve the optoelectronic properties on indium phosphide quantum dots. Chem Mater 2020;32:2795-802.
40. Nemoto K, Watanabe J, Sun HT, Shirahata N. Coherent InP/ZnS core@shell quantum dots with narrow-band green emissions. Nanoscale 2022;14:9900-9.
41. Cho E, Jang H, Lee J, Jang E. Modeling on the size dependent properties of InP quantum dots: a hybrid functional study. Nanotechnology 2013;24:215201.
42. Taylor DA, Teku JA, Cho S, Chae W, Jeong S, Lee J. Importance of surface functionalization and purification for narrow FWHM and bright green-emitting InP core–multishell quantum dots via a two-step growth process. Chem Mater 2021;33:4399-407.
43. Jo J, Jo D, Choi S, et al. Highly bright, narrow emissivity of InP quantum dots synthesized by aminophosphine: effects of double shelling scheme and Ga treatment. Adv Opt Mater 2021;9:2100427.
44. Kim TG, Zherebetskyy D, Bekenstein Y, et al. Trap passivation in indium-based quantum dots through surface fluorination: mechanism and applications. ACS Nano 2018;12:11529-40.
45. Xu S, Ziegler J, Nann T. Rapid synthesis of highly luminescent InP and InP/ZnS nanocrystals. J Mater Chem 2008;18:2653-6.
46. Tessier MD, Baquero EA, Dupont D, et al. Interfacial oxidation and photoluminescence of InP-based core/shell quantum dots. Chem Mater 2018;30:6877-83.
47. Ubbink RF, Almeida G, Iziyi H, et al. A water-free in situ HF treatment for ultrabright InP quantum dots. Chem Mater 2022;34:10093-103.
48. Anderson NC, Hendricks MP, Choi JJ, Owen JS. Ligand exchange and the stoichiometry of metal chalcogenide nanocrystals: spectroscopic observation of facile metal-carboxylate displacement and binding. J Am Chem Soc 2013;135:18536-48.
49. Kirkwood N, Monchen JOV, Crisp RW, et al. Finding and fixing traps in II-VI and III-V colloidal quantum dots: the importance of Z-type ligand passivation. J Am Chem Soc 2018;140:15712-23.
50. Purcell-milton F, Chiffoleau M, Gun’ko YK. Investigation of quantum dot–metal halide interactions and their effects on optical properties. J Phys Chem C 2018;122:25075-84.
51. Calvin JJ, Swabeck JK, Sedlak AB, Kim Y, Jang E, Alivisatos AP. Thermodynamic investigation of increased luminescence in indium phosphide quantum dots by treatment with metal halide salts. J Am Chem Soc 2020;142:18897-906.
52. Tessier MD, Dupont D, De Nolf K, De Roo J, Hens Z. Economic and size-tunable synthesis of InP/ZnE (E = S, Se) colloidal quantum dots. Chem Mater 2015;27:4893-8.
53. Zhu Y, Shen C, Xu X, et al. Photoluminescence properties of InP/GaP/ZnS core/shell/shell colloidal quantum dots treated with halogen acids. J Lumin 2023;256:119651.
54. Jun B, Lee HK, Park Y, Kwon Y. Degradation of full aromatic polyamide NF membrane by sulfuric acid and hydrogen halides: change of the surface/permeability properties. Polym Degrad Stabil 2019;162:1-11.
55. Park J, Won YH, Kim T, Jang E, Kim D. Electrochemical charging effect on the optical properties of InP/ZnSe/ZnS quantum dots. Small 2020;16:e2003542.
56. Li H, Bian Y, Zhang W, et al. High performance InP-based quantum dot light-emitting diodes via the suppression of field-enhanced electron delocalization. Adv Funct Mater 2022;32:2204529.
Cite This Article
How to Cite
Jo, D. Y.; Kim H. M.; Park G. M.; Shin D.; Kim Y.; Kim Y. H.; Ryu C. W.; Yang H. Unity quantum yield of InP/ZnSe/ZnS quantum dots enabled by Zn halide-derived hybrid shelling approach. Soft Sci. 2024, 4, 27. http://dx.doi.org/10.20517/ss.2024.19
Download Citation
Export Citation File:
Type of Import
Tips on Downloading Citation
Citation Manager File Format
Type of Import
Direct Import: When the Direct Import option is selected (the default state), a dialogue box will give you the option to Save or Open the downloaded citation data. Choosing Open will either launch your citation manager or give you a choice of applications with which to use the metadata. The Save option saves the file locally for later use.
Indirect Import: When the Indirect Import option is selected, the metadata is displayed and may be copied and pasted as needed.
About This Article
Special Issue
Copyright
Data & Comments
Data
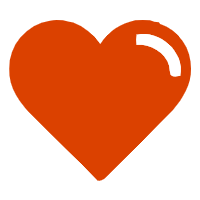
Comments
Comments must be written in English. Spam, offensive content, impersonation, and private information will not be permitted. If any comment is reported and identified as inappropriate content by OAE staff, the comment will be removed without notice. If you have any queries or need any help, please contact us at support@oaepublish.com.