Organic flexible thermoelectrics for thermal control
Abstract
Despite the lower efficiency for thermoelectric cooling technology compared to conventional mechanical cooling technology, it finds application in commercial portable cooling due to its compactness, simple device design, and low noise. The rapid progress in flexible and wearable electronics opens the need for flexible cooling technology for local thermal regulation where thermoelectric cooling technology offers niche advantages suitable for flexible cooling such as light weight and no moving parts. Organic thermoelectrics hold promise for flexible and wearable cooling applications due to their intrinsic mechanical flexibility, low thermal conductivity, and ease of processing. However, research on organic Peltier cooling devices remains limited, and more work is required to exploit their potential for flexible cooling applications. This review discussed the state-of-the-art organic Peltier cooling devices and the materials and device design considerations required for advancing organic Peltier device technology toward practical applications.
Keywords
INTRODUCTION
Refrigeration plays an essential role in modern society where it is key to storage, science, quality of life and beyond. Around 25%-30% of global electricity consumption is spent on it[1]. Mechanical refrigeration based on vapor-compression cycle has enabled wide spread of commercial refrigerators due to their high efficiency. However, for some applications, it is undesirable to involve mechanical moving parts, and hence, novel concepts based on magnetocaloric, electrocaloric and thermoelectric cooling have emerged, targeting small-scale applications[2-4]. Among these emerging technologies, thermoelectric cooling based on the Peltier effect has the following advantages: lightweight, compact, and simple device structure, making it suitable for portable refrigeration equipment. The Peltier effect was first discovered in 1,834 where the application of a current through a conductor/semiconductor transfers the heat from one side to the other, hence creating a hot end and a cold end. The advantages of the Peltier cooling elements have triggered substantial research since the demonstration of thermoelectric cooling based on bismuth telluride, Bi2Te3, in 1954[5]. However, one of the key challenges of Peltier cooling technology is its relatively low efficiency, limiting its applications to niche areas in portable devices, such as containers in automobiles with thermal regulation functions, where lower efficiency can be accepted[6].
The rise of flexible and wearable electronics in recent years has opened up many niche application areas that conventional mechanical refrigeration could not be used such as temperature regulation of the human body and flexible cooling devices for analgesia[7]. Generally, two methods can introduce mechanical flexibility to thermoelectric devices: reducing the thickness of inorganic thermoelectric materials and working with materials systems that possess intrinsic mechanical flexibility such as organic semiconductors, 2D materials, etc. While the development of thin inorganic thermoelectric materials on soft substrates has already led to numerous flexible cooling devices including high performance wearable thermoelectric devices with temperature differences over 10 °C and coefficients of performance > 1.5[8-10] flexible organic cooling devices are scarce due to various challenges such as high conductivity anisotropy[11]. The inorganic thermoelectric approach could attain higher cooling performance as inorganic thermoelectric materials are more established and possess higher ZT (thermoelectric figure of merit) factor over 1. Since the existing reviews mostly covered Peltier cooling based on inorganic thermoelectric materials[1,9], we focus on organic materials for Peltier cooling applications. We aim to provide a comprehensive overview of the state-of-the-art organic Peltier cooling work and insights into materials and device design aspects required for advancing organic thermoelectric coolers.
ORGANIC PELTIER COOLERS
Organic semiconductors have enabled a paradigm shift in the small to medium display market due to their excellent optoelectronic properties, ease of processing and low cost. They continue to find applications in areas of emerging technologies such as photodetectors, bioelectronics and thermoelectrics[12-14]. Organic thermoelectrics research is fueled by the advances in carrier mobility and efficient doping strategies over the past two decades through synthetic design and morphology control, hence boosting the thermoelectric power[12],
where S and σ are the Seebeck coefficient and electrical conductivity, respectively. For thermoelectric materials, their thermoelectric performance is determined by a dimensionless figure of merit[15],
where k is thermal conductivity. Given the typical low thermal conductivity in organic semiconductors due to the weak Van der Waals interactions that limit the heat transfer mediated by phonons, the improvement in thermoelectric power factor leads to a high ZT value approaching 1 for both p- and n-type organic semiconductors[16-19]. The intrinsic mechanical flexibility of organic semiconductors makes them particularly attractive for flexible thermoelectric applications.
While research on organic semiconductors-based thermoelectric energy harvesting (Seebeck effect) has steadily increased over the past decade[20], experimental work on the Peltier effect in organic semiconductors remains relatively unexplored. While the Seebeck effect is directly linked to the Peltier effect by thermodynamics, different to Seebeck effect measurement that simply involves the measurement of thermo-voltage under a temperature gradient, Peltier effect measurement is more complex as passing an electrical current through a thermoelectric material would involve not only the heat transfer from one end to the other end due to the Peltier effect but also Joule heating and heat conduction[1]. A major challenge with organic semiconductors is their relatively high electrical resistivity and charge transport anisotropy such that their out-of-plane resistivity is often the highest. This limitation makes the amount of Joule heating in vertical organic semiconductor junction to be very significant and could easily overshadow the Peltier effect as the cooling achieved by the Peltier effect linearly depends on electrical current whereas heating generated by the Joule effect has a quadratic dependence on the applied electrical current. For thermoelectric cooling, the device performance is often determined by several parameters such as the maximum cooling temperature achievable, response time, coefficient of performance, and maximum cooling capacity[9]. The derivation of these parameters and their dependence on thermoelectric materials parameters are well covered by other review papers[1,4,6]. Nevertheless, in order to achieve efficient thermoelectric cooling, it is important to work with high ZT materials considering whether the ZT value is anisotropic and the electrical resistivity of the thermoelectric material along the direction of the current.
State-of-the-art organic Peltier coolers
The first work experimented with Peltier cooling in organic semiconductors was performed by Hu et al. in 2005[21]. They observed the first signs of Peltier cooling in polypyrrole (PPy) powder despite the weak and unstable effect due to material degradation under electrical current. Similarly, the experimental setup used to probe the cooling effect is also non-optimal as the polymer particles are directly placed between two metal plates, and the thermal insulation chamber is not well-designed, making measurement of tiny temperature changes using thermocouple attached/thermal camera challenging [Figure 1A]. It is worth mentioning that the Seebeck coefficient for PPy is only 10 µV/K, which is small compared with current high performance organic thermoelectric materials. Since then, organic semiconductor research has accelerated, motivated by commercializing organic light-emitting diodes with several high ZT materials developed by optimizing the charge transport and doping in the organic materials[12]. These high ZT organic thermoelectric materials show promising performance for energy harvesting applications. However, research in Peltier cooling with organic semiconductors did not progress significantly despite the discovery of these high ZT organic thermoelectric materials due to large Joule heating coming from the relatively high electrical resistivity and heat conduction with the material[1,11].
Figure 1. (A) Schematic illustration of the test rig used to measure Peltier effect in PPy in 2005. Reproduced with permission[21]. Copyright 2005, Elsevier; (B) Schematic illustration of the suspended Poly(Ni-ett) Peltier measurements and results. Reproduced with permission[11]. Copyright 2018, Nature Publishing Group; (C) Experimental setup used for the doped C60 Peltier coolers with the temporal cooling response. Images reproduced with permission[22]. Copyright 2022, Wiley-VCH. PPy: Peltier cooling in polypyrrole.
Jin et al. have made major progress in fundamental understanding of the Peltier effect in high ZT organic semiconductors [poly(Ni-ett)] in 2018, where they used a suspended device design under vacuum to minimize heat dissipation together with an alternative current measurement scheme to separate the temperature change induced by Joule heating and Peltier effect[11]. In particular, they showed the possibility to achieve large Peltier cooling around 40 K under high applied current density of 5 A·mm-2 highlighting the potential of organic Peltier cooling devices [Figure 1B]. Wang et al. tried to overcome the typical electrical conductivity anisotropy in organic semiconductors by working with n-type doped C60 thin films with decent ZT factor of around 0.1 where the buckyball structure of C60 allows the charge transport to be nearly isotropic within the film[22]. The results show net cooling under electrical current excitation implying that vertical electrical conductivity is an important roadblock in organic Peltier cooling devices [Figure 1C]. Moreover, they showed that the vertical organic Peltier devices have a fast response time of around 25 µs, making them one of the fastest micro-thermoelectric coolers[9]. Both studies use thermal reflectance microscopy techniques to measure the temperature change induced by Peltier cooling. The thermal reflectance microscopy technique has the advantages of being non-destructive, non-contact, full-field and real-time, making probing the Peltier cooling devices straightforward.
Another branch of organic Peltier cooling devices involves molecular junctions that are important for understanding electrical and thermal transport at the molecular scale. The ultralow cooling power in the picowatt range in molecular junction makes the measurements challenging in terms of technical aspects. Cui et al. demonstrated molecular refrigeration in molecular junctions using a conductive AFM technique and integrated calorimetric microdevices[23]. This measurement scheme allows the precise formation of molecular junctions at desired regions and accurate measurement of heat transfer with the calorimetric device. The measurement scheme with calorimetric devices would also apply to organic Peltier cooling devices where a high-precision heat sensor is required when the cooling power is limited.
Material design considerations
One of the key material properties for advancing the organic Peltier cooling device is improving vertical electrical conductivity. Two factors contribute to the electrical conductivity: the charge carrier mobility and the number of free charge carriers in the material. For organic semiconductors, the number of charge carriers can be controlled by molecular doping. There has been significant progress in achieving highly efficient doping in organic semiconductors with synthesis of novel molecular dopants and new processing strategies allowing the free charge carrier density of more than 1020 cm-3 possible[24-27]. Moreover, the new processing method, such as sequential and ion-exchange doping, allows the order of the organic semiconductor to be preserved after doping, which is important for achieving high electrical conductivity greater than 1,000 S/cm[27]. However, there is one issue with enhancing electrical conductivity by doping as increasing carrier concentration also led to a decrease in the Seebeck coefficient, such that there is an optimum doping concentration for maximizing the thermoelectric ZT factor[12]. On the other hand, increasing electrical conductivity by enhancing charge carrier mobility has little impact on the Seebeck coefficient and is, therefore, a more promising approach in boosting the thermoelectric ZT factor. Organic semiconductors typically have strong conductivity anisotropy where the out-of-plane carrier mobility is orders of magnitude lower than in-plane conductivity limited by its insulating side chains[28]. One possible way to circumvent the anisotropy limitation on vertical electrical conductivity is to align the organic semiconductor and orient their high-mobility in-plane polymer chains perpendicular to the substrate [Figure 2A]. As shown in Figure 2A and B, the vertical electrical conductivity of poly(3-hexylthiophene) (P3HT) could be improved by several orders of magnitude to around 3 cm2·V-1·s-1 by orienting the in-plane polymer chains perpendicular to the substrate using a mechanical pressing method[28]. Another possibility for small molecule devices is introducing order in the molecules with a novel crystallization method, thereby improving the vertical charge carrier transport[29]. The process involves transforming vacuum-deposited amorphous organic semiconductor thin films into highly ordered organic semiconductor crystalline thin films. The thickness of the crystalline film can be tuned by subsequent deposition of molecules by epitaxial growth on the formed crystalline template layer. In addition, dopants can be introduced during epitaxial growth to introduce free charge carriers and, hence, increase the electrical conductivity.
Figure 2. (A) Schematic illustration of the P3HT polymer chain orientation and the process of aligning the polymer chain to chain-on orientation. The scale bar denotes 10 µm; (B) The current-voltage characteristics and the extracted charge carrier mobility of the aligned P3HT in comparison to conventional smooth film. Images reproduced with permission[28]. Copyright 2016, Wiley-VCH. P3HT: Poly(3-hexylthiophene).
Another approach to tune the vertical electrical conductivity and other thermoelectric material parameters is by blending different materials. Numerous high ZT inorganic thermoelectric materials are developed from classic Bi2Te3 to other emerging Sn-based alloys with ZT factor over 1[30]. There has been continuous effort in optimizing the thermoelectric ZT factor through materials composites such as carbon nanotube-organic semiconductors and Bi2Te3-organic semiconductor systems[31-33]. The aim is to achieve a material composite with the best of two worlds, i.e., improve the electrical conductivity by incorporating inorganic materials/carbon nanotubes while lowering the overall thermal conductivity by the organic semiconductors. In addition, other interfacial effects at the interfaces of the two materials, such as energy filtering and phonon scattering, could further contribute to improving the thermoelectric device performance[34]. Currently, research efforts have focused on improving the thermoelectric ZT factor through the blend material system for energy harvesting applications, but this approach would also hold promise for engineering the material parameters required for efficient organic Peltier cooling devices.
Device design considerations
Apart from optimizing the materials, it is important to consider the device design to achieve optimal organic Peltier cooling devices. The geometry of the Peltier cooling modules plays an important role in determining the cooling performance of the device. Two dimensionless parameters are used to characterize the geometry of the Peltier module: Aspect ratio (AR) and fill factor (FF)[8]. The AR describes the geometry of the Peltier leg while the FF express how compact the Peltier elements are packed, as given by:
where l and w denote leg length and width, respectively. N is the total number of legs, and A is the module base area. Increasing the leg length, and hence AR, would result in a larger sustainable temperature gradient (ΔT) but a reduction in the cooling capacity (ΔQ). For high FF, the ΔQ is high as there is compact distribution of Peltier elements, but the maximum achievable ΔT is reduced compared to the case for low FF due to the thermal interaction between nearby Peltier elements[8]. The trade-off between the ΔT and ΔQ can, nevertheless, be avoided using cascade Peltier modules where multiple stages of Peltier elements are stacked together with the thin device with high ΔQ in contact with the heat reservoir and thicker devices stacked on top of the thinner device to enable higher ΔT. In terms of the fabrication technology for such cascade Peltier devices with organic semiconductors, inkjet printing or electrohydrodynamic printing would be a suitable preparation technique as these deposition methods waste minimal material and could control the thickness precisely by adjusting the number of printing passes[12].
Another important aspect to consider for organic Peltier cooling devices is contact resistance. Two types of contact resistance exist: the electrical contact resistance between the electrode and organic semiconductor interface and the thermal contact resistance that can either be internal (between the electrode and organic semiconductor) or external (between the Peltier cooling device and the heat source). The interfacial electrical contact resistance would need to be minimized to avoid additional Joule heating in the device. The electrical contact resistance could be reduced by contact doping and the selection of electrode material with work function aligning with the energy level of the organic semiconductors where a lot of work has been done in the organic transistor technology[35]. As for thermal contact resistance, the ideal internal thermal contact resistance has been estimated to be < 1 × 10-7 m2·K·W-1 according to computational simulations[36]. The external thermal contact resistance is more complex and generally depends on the adhesion of the Peltier cooling device on the heat source and their thermal conductivity mismatch. Under a thermally resistive environment such as the human body, the thermoelectric ZT factor is not the only decisive factor for the cooling capacity attainable where a low thermal conductivity can result in a significantly higher cooling capacity in comparison to the same ZT factor achieved by either electrical conductivity or Seebeck coefficient [Figure 3] making organic Peltier cooling devices attractive for wearable cooling applications[8,22].
Figure 3. Simulated Peltier cooling capacity as a function of current for different cases of heat transfer coefficient (h) with varying thermoelectric material parameters. The smaller the h denotes a more thermally resistive environment. Reproduced with permission[8]. Copyright 2019, Nature Publishing Group.
Reliability and stability are other important device considerations apart from the Peltier cooling performance. For organic thermoelectrics, the air stability for n-type doped organic semiconductors is a challenge, and hence, the design of proper encapsulation is necessary[37]. However, in the context of flexible Peltier cooling devices, novel encapsulation methods would need to be applied to allow flexibility and minimal thermal insulation. One possibility would be using the multilayer laminate for flexible organic light-emitting diode applications[38].
CONCLUSION AND OUTLOOK
The rapid development of flexible and wearable electronics necessitates complementary thermal regulation technology where flexible Peltier cooling devices would be a promising solution. Organic Peltier cooling devices possess the potential for fulfilling flexible and wearable cooling requirements due to their intrinsic mechanical flexibility, low thermal conductivity, light weight and ease of processing. However, despite the steady progress in organic thermoelectrics for flexible energy harvesting applications, research on these devices is far from satisfactory. This review summarized the key developments in the organic Peltier cooling devices. We discussed potential approaches to advance the cooling performance of organic Peltier devices from materials design and processing perspective and methods to ensure the reliability of organic Peltier cooling devices for practical applications.
DECLARATIONS
Authors’ contributions
The author contributed solely to the article.
Availability of data and materials
Not applicable.
Financial support and sponsorship
Wang SJ acknowledges the start-up fund (Nos. 41.4561.179500 and 21.4561.162996) provided by Hong Kong Baptist University.
Conflicts of interest
The author declared that there are no conflicts of interest.
Ethical approval and consent to participate
Not applicable.
Consent for publication
Not applicable.
Copyright
© The Author(s) 2024.
REFERENCES
1. Ding J, Zhao W, Jin W, Di C, Zhu D. Advanced thermoelectric materials for flexible cooling application. Adv Funct Mater 2021;31:2010695.
2. Gómez J, Ferreiro Garcia R, De Miguel Catoira A, Romero Gómez M. Magnetocaloric effect: a review of the thermodynamic cycles in magnetic refrigeration. Renew Sustain Energy Rev 2013;17:74-82.
4. Zhao D, Tan G. A review of thermoelectric cooling: materials, modeling and applications. Appl Therm Eng 2014;66:15-24.
5. Goldsmid HJ, Douglas RW. The use of semiconductors in thermoelectric refrigeration. Br J Appl Phys 1954;5:386-90.
6. Wang Y, Yang L, Shi XL, et al. Flexible thermoelectric materials and generators: challenges and innovations. Adv Mater 2019;31:e1807916.
7. Reeder JT, Xie Z, Yang Q, et al. Soft, bioresorbable coolers for reversible conduction block of peripheral nerves. Science 2022;377:109-15.
8. Kishore RA, Nozariasbmarz A, Poudel B, Sanghadasa M, Priya S. Ultra-high performance wearable thermoelectric coolers with less materials. Nat Commun 2019;10:1765.
9. Zhang Q, Deng K, Wilkens L, Reith H, Nielsch K. Micro-thermoelectric devices. Nat Electron 2022;5:333-47.
10. Hong S, Gu Y, Seo JK, et al. Wearable thermoelectrics for personalized thermoregulation. Sci Adv 2019;5:eaaw0536.
11. Jin W, Liu L, Yang T, et al. Exploring Peltier effect in organic thermoelectric films. Nat Commun 2018;9:3586.
12. Russ B, Glaudell A, Urban JJ, Chabinyc ML, Segalman RA. Organic thermoelectric materials for energy harvesting and temperature control. Nat Rev Mater 2016;1:16050.
15. He J, Tritt TM. Advances in thermoelectric materials research: looking back and moving forward. Science 2017;357:eaak9997.
16. Liu J, van der Zee B, Alessandri R, et al. N-type organic thermoelectrics: demonstration of ZT > 0.3. Nat Commun 2020;11:5694.
17. Sun Y, Qiu L, Tang L, et al. Flexible n-type high-performance thermoelectric thin films of poly(nickel-ethylenetetrathiolate) prepared by an electrochemical method. Adv Mater 2016;28:3351-8.
18. Bubnova O, Khan ZU, Malti A, et al. Optimization of the thermoelectric figure of merit in the conducting polymer poly(3,4-ethylenedioxythiophene). Nat Mater 2011;10:429-33.
19. Kim GH, Shao L, Zhang K, Pipe KP. Engineered doping of organic semiconductors for enhanced thermoelectric efficiency. Nat Mater 2013;12:719-23.
20. Zeng YJ, Wu D, Cao XH, Zhou WX, Tang LM, Chen KQ. Nanoscale organic thermoelectric materials: measurement, theoretical models, and optimization strategies. Adv Funct Mater 2020;30:1903873.
21. Hu E, Kaynak A, Li Y. Development of a cooling fabric from conducting polymer coated fibres: proof of concept. Synth Met 2005;150:139-43.
22. Wang S, Wohlrab S, Reith H, et al. Doped organic micro-thermoelectric coolers with rapid response time. Adv Elect Mater 2022;8:2200629.
23. Cui L, Miao R, Wang K, et al. Peltier cooling in molecular junctions. Nat Nanotechnol 2018;13:122-7.
24. Wang SJ, Panhans M, Lashkov I, et al. Highly efficient modulation doping: a path toward superior organic thermoelectric devices. Sci Adv 2022;8:eabl9264.
25. Walzer K, Maennig B, Pfeiffer M, Leo K. Highly efficient organic devices based on electrically doped transport layers. Chem Rev 2007;107:1233-71.
26. Salzmann I, Heimel G, Oehzelt M, Winkler S, Koch N. Molecular electrical doping of organic semiconductors: fundamental mechanisms and emerging dopant design rules. Acc Chem Res 2016;49:370-8.
27. Yamashita Y, Tsurumi J, Ohno M, et al. Efficient molecular doping of polymeric semiconductors driven by anion exchange. Nature 2019;572:634-8.
28. Skrypnychuk V, Wetzelaer GJ, Gordiichuk PI, et al. Ultrahigh mobility in an organic semiconductor by vertical chain alignment. Adv Mater 2016;28:2359-66.
29. Sawatzki-Park M, Wang SJ, Kleemann H, Leo K. Highly ordered small molecule organic semiconductor thin-films enabling complex, high-performance multi-junction devices. Chem Rev 2023;123:8232-50.
30. Zhan S, Hong T, Qin B, et al. Realizing high-ranged thermoelectric performance in PbSnS2 crystals. Nat Commun 2022;13:5937.
31. Bounioux C, Díaz-chao P, Campoy-quiles M, et al. Thermoelectric composites of poly(3-hexylthiophene) and carbon nanotubes with a large power factor. Energy Environ Sci 2013;6:918.
32. Jiang Q, Yang J, Hing P, Ye H. Recent advances, design guidelines, and prospects of flexible organic/inorganic thermoelectric composites. Mater Adv 2020;1:1038-54.
33. Blackburn JL, Ferguson AJ, Cho C, Grunlan JC. Carbon-nanotube-based thermoelectric materials and devices. Adv Mater 2018;30:1704386.
34. Kim C, Lopez DH. Energy filtering and phonon scattering effects in Bi2Te3-PEDOT:PSS composite resulting in enhanced n-type thermoelectric performance. Appl Phys Lett 2022;120:063903.
35. Borchert JW, Weitz RT, Ludwigs S, Klauk H. A critical outlook for the pursuit of lower contact resistance in organic transistors. Adv Mater 2022;34:e2104075.
36. Gao W, Lin W, Lu E. Numerical study on natural convection inside the channel between the flat-plate cover and sine-wave absorber of a cross-corrugated solar air heater. Energy Convers Manag 2000;41:145-51.
37. Yuan D, Liu W, Zhu X. Efficient and air-stable n-type doping in organic semiconductors. Chem Soc Rev 2023;52:3842-72.
Cite This Article
Export citation file: BibTeX | EndNote | RIS
OAE Style
Wang SJ. Organic flexible thermoelectrics for thermal control. Soft Sci 2024;4:25. http://dx.doi.org/10.20517/ss.2024.14
AMA Style
Wang SJ. Organic flexible thermoelectrics for thermal control. Soft Science. 2024; 4(3): 25. http://dx.doi.org/10.20517/ss.2024.14
Chicago/Turabian Style
Shu-Jen Wang. 2024. "Organic flexible thermoelectrics for thermal control" Soft Science. 4, no.3: 25. http://dx.doi.org/10.20517/ss.2024.14
ACS Style
Wang, S.J. Organic flexible thermoelectrics for thermal control. Soft. Sci. 2024, 4, 25. http://dx.doi.org/10.20517/ss.2024.14
About This Article
Special Issue
Copyright
Data & Comments
Data
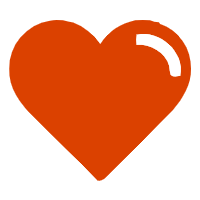
Comments
Comments must be written in English. Spam, offensive content, impersonation, and private information will not be permitted. If any comment is reported and identified as inappropriate content by OAE staff, the comment will be removed without notice. If you have any queries or need any help, please contact us at support@oaepublish.com.