Potential applications of engineered bacteria in disease diagnosis and treatment
Abstract
Probiotics are live microorganisms that confer health benefits to the host when administered in appropriate quantities. This beneficial effect has spurred extensive research in the medical and health fields. With rapid advancements in synthetic biology, the genetic and biological characteristics of a broad array of probiotics have been elucidated. Utilizing these insights, genetic editing technologies now enable the precise modification of probiotics, leading to the development of engineered bacteria. Emerging evidence underscores the significant potential of these engineered bacteria in disease management. This review explores the methodologies for creating engineered bacteria, their preliminary applications in healthcare, and the mechanisms underlying their functions. Engineered bacteria are being developed for roles such as in vivo drug delivery systems, biosensors, and mucosal vaccines, thereby contributing to the treatment, diagnosis, and prevention of conditions including inflammatory bowel disease (IBD), metabolic disorders, cancer, and neurodegenerative diseases. The review concludes by assessing the advantages and limitations of engineered bacteria in the context of disease management.
Keywords
INTRODUCTION
Probiotics are defined as “live microorganisms that, when administered in sufficient quantities, provide health benefits to the host”[1]. Research has increasingly focused on exploring the genetic structure and biological properties of diverse probiotics. Especially as the well-deserved hotspot, in-depth exploration of Lactobacillus and Bifidobacterium facilitates the identification and manipulation of crucial metabolic pathways and regulatory mechanisms[2]. Progressively clearer genetic backgrounds and inherent safety help make probiotics excellent vectors for engineered modifications.
Technological innovations in synthetic biology, high-throughput sequencing, omics, synthetic genomics, and other advanced fields have provided novel perspectives for the study of probiotics. Technologies like genetic programming and high-throughput sequencing enable the precise engineering of biological systems, analogous to techniques in electrical engineering[3]. Engineered bacteria are genetically modified to express exogenous genes or execute specific biological functions for treating, preventing, or diagnosing diseases[4]. Animal studies have demonstrated that engineered bacteria can influence a wide range of conditions including tumors[5], inflammatory bowel disease (IBD)[6], diabetes[7], and neurodegenerative disorders[8], spanning various categories such as infections, metabolism, and oncology. Some have progressed to clinical trials, such as Escherichia coli Nissle 1917 (EcN) engineered for colorectal cancer detection and treatment[9]. Engineered bacteria exhibit promising potential in vivo, leveraging both their inherent probiotic traits and engineered functionalities to enhance therapeutic efficacy.
This paper reviews current research on the applications and mechanisms of engineered bacteria for treating IBD, metabolic disorders, neurodegenerative conditions, and cancer. We also review the utilization of biosensors and mucosal vaccines built on engineered bacteria. Finally, we summarize the advantages and drawbacks of engineered bacteria in disease management.
THE PREDECESSOR OF ENGINEERED BACTERIA - PROBIOTICS
Although many food supplements contain active microorganisms, they are categorized as probiotics only if they have been conclusively shown to benefit human health[10]. Common sources of widely used probiotics such as Lactobacillus and Bifidobacterium spp. include fermented foods, the human gut and vagina, and various plants[2]. Numerous animal studies have demonstrated that probiotics can enhance intestinal microecology, modulate host immunity and metabolism, provide resistance against infections, and potentially combat tumors. These effects depend on the probiotic itself or its secretions. For example, in the intestine, probiotics preempt the adhesion sites on intestinal epithelial cells through their adhesion proteins, thereby reducing the colonization of pathogenic bacteria[11]. Probiotics also secrete bacteriocins that inhibit the growth and proliferation of pathogenic bacteria such as Listeria, Clostridium, and Salmonella through mechanisms including pore formation, which disrupts the selective permeability of the target cell membrane[12], interference with cell wall synthesis[13], and enhancement of the host’s immune response[14].
Notably, significant progress has been made in cancer therapy, such as the discovery that Lactobacillus reuteri ATCC BAA-2837 and its metabolite indole-3-aldehyde can enhance the effectiveness of immune checkpoint inhibitors in treating melanoma[15]. Recent clinical trials have also highlighted probiotics’ potential in managing conditions like anxiety and depression[16], irritable bowel syndrome[17], functional dyspepsia[18], and postoperative constipation[19]. These findings underscore the promising role of probiotics in disease management. Strategies for microecological regulation of the human gut commonly include probiotic supplementation and fecal microbiota transplantation (FMT). However, these nonspecific approaches can have serious consequences due to the limited understanding of host-microbiota interactions. For instance, a patient with acute promyelocytic leukemia who consumed probiotic-enriched yogurt following diarrhea developed infectious shock one week later. The strain responsible for this adverse event was Lactobacillus rhamnosus GG[20]. This case is not unique; a study reported 48 clinical safety events related to probiotics between 2019 and 2021, including bacteremia, sepsis, and endocarditis[21]. These reports indicate that the safety of probiotics in immunocompromised hosts requires further evaluation. FMT, due to strict donor restrictions and inconsistent screening criteria across healthcare institutions, complicates the establishment of a unified risk profile, hindering its long-term clinical application[22]. Therefore, supplementation with probiotics and FMT may result in nonspecific adverse events, limiting their broader application. However, the development of engineered bacteria presents potential solutions to these challenges. While probiotics are natural candidates for engineering due to their beneficial properties, additional factors must also be considered. Researchers must also take into account factors such as colonization characteristics, ease of genetic modification, and available tools and techniques. In this context, E. coli, Lactobacillus, and Bifidobacterium spp. are considered promising chassis bacteria for genetic manipulation. For example, probiotic Lactobacillus species have been recognized as enerally recognized as safe (GRAS) after extensive use and investigation, providing a foundation for the subsequent development of food-grade carriers[23]. For instance, inducible plasmid self-destruction (IPSD)-assisted genome engineering can introduce homologous DNA into Lactobacillus and Bifidobacterium spp.[24]. The tetracycline-induced expression system and the CRISPR/Cas9 system facilitate precise control and editing of its genes[25]. These properties enable the continued advancement of probiotics as engineered microorganisms.
To avoid ambiguity, we hereby declare that the probiotics mentioned in this article refer only to strains that are known to be beneficial to the host, rather than including all strains indiscriminately. For example, when referring to “probiotics such as Lactobacillus and Bifidobacterium spp.” we are specifically referring to those species within these genera that have been proven to be beneficial to human health, and not to all species within the genera. Strains that are harmful or have not been confirmed to be beneficial are not within the scope of this discussion.
POTENTIAL APPLICATIONS OF ENGINEERED BACTERIA IN DISEASE MANAGEMENT
The rapid advancement of synthetic biology technologies[26] has facilitated their transition from laboratory settings to clinical trials, driving research toward in vivo therapeutics using engineered bacteria in the 21st century. Our review of engineered bacteria underscores their crucial role in disease management involving treatment, diagnosis, and prevention.
Potential applications of in vivo drug delivery systems developed based on engineered bacteria in disease treatment
As traditional drug delivery systems struggle to match the pace of disease progression, emerging methods are increasingly favored. The short half-life, high toxicity, and expense of certain drugs necessitate novel and more efficient delivery systems. Genetic engineering of probiotics enables precise modulation of heterologous protein expression, facilitating efficient production of target proteins, peptides, and small-molecule metabolites, among others. In vivo drug delivery systems utilizing these engineered bacteria have demonstrated significant potential in treating IBD, metabolic disorders, neurodegenerative disease, cancer, and various other diseases [Figure 1].
Figure 1. Mechanistic insights into the application of engineered bacterial-based in vivo drug delivery systems for disease therapy. (A) Intestinal in situ production of anti-inflammatory mediators; (B) Diabetes therapy in animal models; (C) Antitumor strategy; (D) Neurodegenerative disease intervention.
IBD
IBD encompasses chronic, nonspecific inflammatory conditions affecting the intestines, notably Crohn’s disease (CD) and ulcerative colitis (UC)[27]. IBD is a global health issue with a complex pathogenesis that involves genetic, immunologic, and microbial factors[28]. From a microbiological standpoint, the disrupted intestinal mucosal barrier in UC, caused by an imbalanced intestinal microecology, leads to inappropriate immune system activation and subsequent tissue damage[29]. Additionally, reactive oxygen species (ROS) and protein hydrolases released by neutrophils during intestinal inflammation can disrupt tight junctions between intestinal epithelial cells, further compromising the intestinal barrier[30]. Furthermore, short-chain fatty acids, which are metabolites of intestinal microorganisms, have been shown to regulate the quantity and function of Tregs, thereby modulating the immune status of the gut[31].
Improving the inflammatory state of the intestine is a key focus in the treatment of IBD. Traditional therapies typically involve the use of anti-inflammatory drugs and immunosuppressive agents, such as anti-TNF-α antibodies. Excessive exposure of active drugs to the intestinal lumen can lead to their absorption into the systemic circulation through the intestinal mucosa, potentially triggering adverse reactions[32]. To minimize unnecessary drug exposure and related side effects, an in vivo drug delivery system based on engineered bacteria has been developed, which enables targeted colonization[33]. In 2000, Steidler et al. first reported the use of engineered Lactococcus lactis to deliver mIL-10 for the treatment of IBD in mice[34]. In this study, the mIL-10 gene sequence was cloned into a plasmid and introduced into Lactococcus lactis via electrotransformation, enabling the bacteria to produce mIL-10. However, the potential accumulation of transgenic strains raised biosafety concerns. To address this, Steidler et al. utilized a clever biocontainment strategy: they constructed a homology arm containing the upstream and downstream regions of the thyA gene, which, when introduced into Lactococcus lactis along with an hIL-10 expression cassette, allowed for the replacement of thyA with hIL-10 through double homologous recombination[35]. Subsequent clinical trials confirmed the efficacy of both the biocontainment strategy and the therapeutic approach[36].
To ensure efficient delivery of target molecules to lesions, engineered bacteria often require modifications to their expression plasmids, such as codon optimization and the addition of secretion signal sequences. Codon optimization involves altering codons in exogenous gene sequences to align with the host cell's preferred codon usage, thereby enhancing translation efficiency. This strategy has been widely applied in the design of engineered bacteria[37]. The process relies heavily on bioinformatics in synthetic biology, alongside advances in molecular biology[38]. Another approach to improving the delivery of heterologous proteins by engineered bacteria is the incorporation of a secretion signal sequence at the N-terminal of the target gene. For instance, in a study by Hanson et al., the genes encoding two key subunits of IL-27, IL-27p28 and Ebi3, were codon-optimized and fused by a contiguous sequence, with a secretion signal sequence added at the N-terminus. The engineered Lactococcus lactis (LL-IL-27) exhibited enhanced IL-27 delivery capacity. This modification made LL-IL-27 more effective in treating IBD in mice compared to both LL-IL-10 and systemically administered recombinant IL-27 [Figure 2]. LL-IL-27 showed efficacy by stimulating IL-10 production in T cells, reducing CD4+ T cells among intestinal lymphocytes, and mitigating inflammatory cell infiltration[39].
Figure 2. Mechanism of intestinal delivery of IL-27 by engineered LL-IL-27 for the treatment of IBD (Created with https://BioRender.com). IBD: Inflammatory bowel disease.
The gastrointestinal environment in IBD presents a complex challenge, as even engineered bacteria that are optimized for safety and production efficiency may be compromised before they reach their target site for colonization. To enhance the bioavailability of these bacteria, researchers have combined them with various biomaterials to create effective drug delivery systems. For example, in a study focused on removing ROS from the IBD gut, researchers used two biocompatible and biodegradable materials - chitosan and sodium alginate - to coat engineered EcN expressing catalase (CAT) and superoxide dismutase (SOD) via a layer-by-layer electrostatic self-assembly technique, resulting in EcN-pE(C/A)2. These biomaterials protected the engineered bacteria from digestive enzymes and the acidic conditions of the gastrointestinal tract, while also enhancing their compatibility with other materials. This strategy improved the stability and bioavailability of the engineered bacteria[40]. Interestingly, EcN can also be designed as an inactive carrier for drug delivery. Researchers introduced the lysin E gene from phage φX174 into EcN, inducing its expression to cause the bacterium to lyse into an empty shell that retains only the surface structure. These “EcN ghosts” have demonstrated safety and efficacy in IBD treatment[41].
To conclude, engineered bacteria function as carriers for localized delivery of live immunoreactive substances, effectively bypassing systemic symptoms and addressing the short half-life concerns of direct cytokine delivery. Future advancements in modulating probiotic genes show significant promise for optimizing this in vivo drug delivery system for treating IBD.
Metabolic disease
Diabetes
Type 2 diabetes (T2D) is characterized by insulin resistance initially, leading to impaired glucose utilization by tissue cells and eventual pancreatic β-cell dysfunction. Dysregulation of the host’s gut microbiota has been implicated in T2D development, impacting metabolism, inflammatory responses, and oxidative stress[42,43]. T2D patients often exhibit an imbalance with increased pathogenic bacteria and reduced butyric acid-producing bacteria in the gut, influencing host immune and metabolic functions[42,44]. Further clinical and animal studies have demonstrated that probiotic supplementation with strains such as Lactobacillus acidophilus, Bifidobacterium lactis, and Lactobacillus casei Shirota can modestly lower blood glucose levels in T2D patients[45,46], suggesting a potential role for probiotics in T2D management.
On another front, glucagon-like peptide-1 (GLP-1), a newly discovered gastrointestinal hormone, stimulates insulin secretion and inhibits glucagon secretion, thereby lowering blood glucose levels[47,48]. However, its short in vivo half-life limits clinical efficacy[49]. In vivo drug delivery systems based on engineered bacteria offer a promising solution to this challenge. As mentioned earlier, probiotics have demonstrated significant potential in the treatment of T2D, making them ideal candidates as chassis bacteria for the development of engineered strains. Engineered strains such as Lactococcus lactis (LL-pUBGLP-1)[50] and Lactobacillus plantarum (L. plantarum-pMG36e-GLP-1)[7,51], transformed with plasmids containing GLP-1 cDNA, can effectively produce and deliver GLP-1 orally, significantly enhancing its therapeutic potential. In animal models and clinical trials, oral administration of Lactobacillus plantarum-pMG36e-GLP-1 reduced pathogenic bacteria such as Prevotella and increased beneficial butyrate-producing Alistipes spp. in the gut of spontaneous T2D monkeys, improving intestinal dysbiosis[51] [Figure 1B]. Additionally, Lactobacillus plantarum-pMG36e-GLP-1 has shown efficacy in mitigating high-fat diet-induced obesity in mice by promoting fatty acid oxidation and modulating intestinal flora[52]. In addition, engineered Lactococcus lactis strains for the treatment of type 1 diabetes (T1D) are under development. For example, an engineered strain of Lactococcus lactis that secretes the intact insulin autoantigen and the immunomodulatory cytokine IL-10 has been shown to successfully cure diabetes in non-obese diabetic (NOD) mice when used in conjunction with low-dose systemic anti-CD3 antibody therapy[53].
E. coli Nissle 1917, a probiotic, exerts influence on glycemic responses through intricate host interactions beyond mere glucose uptake[54]. Like probiotic Lactobacilli, E. coli Nissle 1917 can be genetically engineered to produce a GLP-1-like product locally, exerting beneficial effects in managing diabetes. Researchers have proposed expressing a cholera toxin B subunit-insulin-like growth factor 1 fusion protein (CTB-IGF-1) using engineered E. coli Nissle 1917. Here, the cholera toxin B subunit (CTB) serves as a carrier protein facilitating the targeting of insulin-like growth factor 1 (IGF-1), which mimics insulin's physiological function via distinct receptors[55]. While this hypothesis has not been confirmed by subsequent reports, analogous studies have been conducted. For instance, engineered E. coli BL21 expressing a CTB-10×rolGLP-1 fusion protein significantly lowered blood glucose levels in mice with T2D[56]. Moreover, engineered E. coli BL21 (DE3) expressing β-cell expansion factor A (BefA) holds promise for T2D treatment by enhancing pancreatic β-cell proliferation while mitigating inflammation and apoptosis[57,58].
Phenylketonuria
Phenylketonuria (PKU) is an inherited metabolic disorder caused by autosomal recessive inheritance, mainly resulting from a deficiency of phenylalanine hydroxylase (PAH) in patients. Additionally, the PAH-catalyzed conversion of phenylalanine (Phe) to tyrosine (Tyr) requires tetrahydrobiopterin (BH4) as a cofactor. Therefore, patients with impaired BH4 metabolism may also develop PKU[59]. This deficiency leads to the excessive accumulation of phenylalanine, which can damage the nervous system[60]. Historically, a strict low-Phe diet has been the cornerstone of PKU treatment[61]. However, long-term dietary control does not alter the underlying nature of the disease. This approach is not only difficult to implement but may also lead to other complications[62]. Recently, new treatment protocols such as gene therapy[63] and enzyme replacement therapy[64] have been proposed and validated. Engineered bacteria could potentially enhance enzyme replacement therapy for PKU.
Enzymes currently under study for treating PKU include phenylalanine lyase (PAL) and PAH, both crucial for reducing Phe levels via metabolic pathways[64,65]. The Anabaena variabilis phenylalanine ammonia lyase gene (AvPAL) was optimized and cloned into Lactobacillus reuteri 100-23C. The engineered bacterium demonstrated a significant reduction in blood Phe levels in mice within 3 to 4 days of administration[66]. AvPAL functions by breaking down accumulated Phe into cis-cinnamic acid and ammonia, which is excreted through normal metabolic pathways. In contrast, PAH can be secreted into the intestines by engineered Lactobacillus plantarum CM_PUJ411, detected using the fluorescent protein. This strain operates through two primary mechanisms: secreted PAH catalyzes the conversion of L-phenylalanine (L-Phe) to L-tyrosine (L-Tyr), which is then excreted via normal metabolic pathways. Additionally, it expresses a cell-penetrating peptide, the TAT protein transduction domain, which enhances the intestinal uptake efficiency of recombinant PAH and potentially reduces degradation within the intestinal lumen[67]. The construction of the two types of engineered bacteria mentioned above involves two common plasmid-based expression systems: the constitutive expression system and the inducible expression system. Typically, plasmid-based expression systems include key elements such as a replicon, a promoter with a ribosome binding site and regulatory sequences, a terminator, a target gene, and a selection marker. In a previous project, a plasmid-based expression system was constructed in Anabaena variabilis, where the promoter of the AvPAL gene was replaced with a high-yield constitutive promoter from Lactobacillus casei (the erythromycin resistance B gene, ermB), enabling sustained and stable expression of PAL in the host cells. PAL expression in the host cell does not require additional inducible signals. In contrast, the pSIP503 expression vector carrying the PAH gene features a nisin-inducible promoter, which drives the transcription of downstream target genes when nisin levels in the environment are elevated. Both constitutive expression systems (e.g., pUBU[68], pPBT-GFP[69], pNZ2103[70]) and inducible systems (e.g., pMSP3535[71], pULP3-PLDH[72], pMY01[73]) offer distinct advantages. Constitutive systems enable stable and efficient expression of therapeutic proteins in complex intestinal environments, while inducible systems are better suited for industrial-scale recombinant protein production. However, a significant drawback of plasmid-based expression systems is the biosafety concern related to the antibiotic resistance genes they often contain. Both the U.S. Food and Drug Administration (FDA) and the European Food Safety Authority (EFSA) have stated that food-grade vectors must be free of antimicrobial resistance elements. Fortunately, alternative methods exist to eliminate antibiotic resistance genes, such as gene editing using the λ Red recombination system. This system typically involves three key proteins: the Exo protein, which degrades single-stranded DNA; the β protein, which facilitates homologous recombination; and the γ protein, which helps convert linear DNA into a circular form for incorporation into the chromosome. Isabella et al. selected the genes for PAL and L-amino acid deaminase (LAAD) as the target genes for Phe degradation and integrated them into the EcN chromosome using the λ Red system. A special plasmid was then introduced to recognize and excise the expression cassette containing the antibiotic resistance genes, using flippase (FLP) recombinase. The expression of the PAL and LAAD genes was controlled by the hypoxia-inducible promoter PfnrS, allowing for gene expression in the hypoxic conditions of the intestine. The engineered bacterium, SYNB1618, was shown to reduce Phe levels in mice and rhesus monkeys by converting Phe into trans-cinnamic acid, which is subsequently metabolized into uric acid and excreted by the host[74].
Several engineered bacterial strains, including TYS8500[75], SYNB1618[74,76], and SYNB1934[77,78], utilize EcN as a vector to express PAL effectively, thereby improving Phe degradation rates. SYNB1618 demonstrated safety and tolerability in clinical trials, achieving a maximum tolerated dose of 2 × 1011 colony-forming units[76]. SYNB1934, optimized for PAL enzyme activity through directed evolution, exhibited enhanced enzyme efficacy and stability, alongside favorable safety and pharmacokinetic profiles in non-human primate models[78]. These studies propose innovative approaches for PKU treatment using engineered bacteria as a therapeutic strategy.
Cancer
Historically, tumor regression has been observed in association with local infections[79]. Advances in understanding the human microbiome, particularly the concept of intratumoral microbiota, highlight the critical role of bacteria in tumor growth. Previous studies have shown that certain bacteria can target and colonize tumor tissue, playing a role in the formation of the tumor microenvironment. For example, EcN, attenuated Salmonella typhimurium, and Lactobacillus paracasei have demonstrated this ability[80-82]. Tumor colonization can occur passively, such as when vascular disruption, caused by a sudden increase in TNF-α in the tumor vasculature, leads to bacterial influx[83]. Additionally, Salmonella typhimurium strains lacking certain chemotactic receptors (e.g., tar, tsr, trg receptors) lose their ability to colonize tumors, suggesting that specific chemical signals in tumor tissues may guide bacterial colonization[84]. Furthermore, the low-oxygen environment of tumor tissues may attract anaerobic bacteria, supporting their colonization. Bacteria colonizing tumor tissues can enhance the body’s ability to fight tumors by reprogramming the tumor microenvironment, particularly through the modulation of immune cells[85]. For example, Lactococcus lactis subsp. cremoris C60 induces a macrophage inflammatory phenotype via TLR signaling, which promotes antigen-dependent activation of tumor-specific CD8+ T cells, thereby enhancing the immune response against melanoma[86]. Additionally, Lactobacillus plantarum L168 and its metabolite indole-3-lactic acid (ILA) stimulate IL-12 production by dendritic cells (DCs), leading to CD8+ T cell activation and improved outcomes in colorectal cancer.
In summary, the specific mechanisms through which natural bacteria target and colonize tumors remain under investigation. However, these bacteria are already promising candidates for chassis engineering due to their potential therapeutic advantages. Before such applications can be realized, the safety concerns surrounding certain bacteria, such as Salmonella typhimurium, need to be addressed. Salmonella typhimurium is a Gram-negative, foodborne pathogen that causes gastrointestinal symptoms and systemic sepsis[87]. In 1999, Low et al. made the first attempt to modify the virulence of Salmonella typhimurium by removing the msbB gene, which is involved in lipopolysaccharide synthesis[88]. They introduced the knockout vector pDBMS7, which contains an incomplete fragment of the msbB gene, into Salmonella typhimurium strain YS501 and used bacterial homologous recombination to replace the wild-type msbB gene. This resulted in attenuated virulence, which was confirmed in mice and pigs. In a similar approach, Clairmont et al. deleted the purI gene, an essential enzyme in the purine synthesis pathway, from the Salmonella genome, creating a purine-deficient strain, VNP20009. This modification limited the bacteria’s undesirable bioaccumulation and enhanced its safety profile[89]. The VNP20009 strain demonstrated some safety in subsequent Phase I clinical studies, although dose-related toxicity was observed, and no significant antitumor effects were noted[81]. Another strategy involves targeting Salmonella pathogenicity island 1 (SPI1), a key virulence factor of Salmonella, whose expression is regulated by ppGpp. Specifically, ppGpp modulates SPI1 gene expression by activating hilA through the activity-dependent pathway of SpoT[90]. Knocking out the relA and spoT genes in Salmonella typhimurium resulted in the ΔppGpp strain, which proved to be nearly non-toxic in mice, showing potential as a vaccine vector[91].
Once the safety concerns are addressed, it will be essential to improve the tumor-targeting and colonization capabilities of engineered bacteria. Although candidate chassis bacteria have demonstrated significant colonization abilities in their natural states, the underlying mechanisms are still largely unknown and challenging to predict. One promising approach to enhance tumor targeting is the incorporation of tumor-targeting molecules onto the surface of engineered bacteria through synthetic biology. This could significantly increase their ability to home in on tumor tissues[92]. For example, the RGD peptide sequence, a known ligand for αvβ3 integrin, which is overexpressed on various tumor cells and endothelial cells during tumor angiogenesis, has been used to enhance targeting. By inserting the RGD peptide sequence into the outer membrane protein A of the attenuated Salmonella strain ΔppGpp, the resulting ΔppGppRGD strain exhibited strong binding to αvβ3-overexpressing cancer cells and demonstrated efficient targeting of αvβ3-expressing tumor xenografts in vivo[93]. Similarly, an engineered strain called 2G9-Salmonella was designed to specifically infect CD20-positive tumor cells. This was achieved by displaying a single-domain antibody (VHH) targeting the CD20 antigen on the outer membrane of the SL3261 aroA-deficient Salmonella strain[94].
Drug molecules or protein molecules with antitumor effects carried by engineered bacteria can effectively kill tumors. For example, the surface of EcN was coupled with the anticancer drug doxorubicin through pH-sensitive amide bonds, and then ligand-linked with photosensitizer gold nanorods (AuNRs) to form EcN-Dox-Au microrobots. With the assistance of NIR laser, the AuNRs produced a photothermal effect, which enhanced the permeability of the tumor cell membrane and promoted the microrobots to deep tumor. The micro-robots penetrated into the deep tumor tissues. Then, doxorubicin was released under the acidic condition of the tumor microenvironment to kill the tumor[95]. In another type of scenario, the engineered bacteria are designed to express proteins with antitumor effects that act in various ways, including activating the host immune system, inducing apoptosis, and interfering with tumor cell metabolism. For example, the Flt3L and OX40L fusion protein expressed by FOLactis promotes DC maturation and T cell activation, converting cold tumors into hot tumors[96]. In addition, attenuated Salmonella typhimurium VNP20009 activates T cells by displaying mPD-1 at the membrane and relieves tumor cells from immune escape[97]. Lactococcus lactis has been genetically modified to carry the gene encoding tumor necrosis factor-related apoptosis-inducing ligand (TRAIL), overcoming its biological half-life limitations and demonstrating apoptosis induction in colon cancer cells in vitro[98]. In addition, engineered Salmonella typhimurium exhibited inhibitory effects on a variety of cancers, including melanoma, colon and pancreatic cancers, by delivering molecules such as shRNA targeting the inhibin α-subunit (INHA)[99], heterotrimeric flagellin FlaB[100], and cytolysin A (ClyA)[101] to the tumor tissues.
In a colon cancer mouse model, a combination therapy involving L-arginine and anti-PD-1 antibodies significantly improved survival rates[102]. To enhance the precise and efficient delivery of L-arginine to tumor tissues, an engineered strain of EcN was developed, demonstrating high efficiency in L-arginine production and synergistically enhancing the effects of anti-PD-1 antibody treatment[103].
Neurodegenerative disease
Neurodegenerative diseases such as Alzheimer’s disease (AD) and Parkinson’s disease (PD) are characterized by progressive neuronal loss in the brain and spinal cord, leading to chronic neurological dysfunction and cognitive or motor decline. Their pathogenesis encompasses intricate factors including neuroinflammation, insulin resistance, mitochondrial dysfunction, and protein misfolding and aggregation[104]. GLP-1, originally recognized for its therapeutic role in metabolic disorders such as diabetes and obesity, has now gained prominence in the realm of neurodegenerative diseases. Studies have demonstrated its ability to mitigate neuroinflammation[105], improve insulin sensitivity[106], confer neuroprotective effects[107], and preserve mitochondrial function[108]. Engineered bacteria represent a promising strategy to tackle the obstacles of GLP-1 therapy. By synthesizing GLP-1 in situ within the gut, they can circumvent its limited half-life. Moreover, probiotics exert influence on the central nervous system by modulating metabolism and immunity via the gut-brain axis[109]. Therefore, the combination of probiotics and GLP-1 is anticipated to play an increasingly significant role in the treatment of neurodegenerative diseases.
In a previous study, we constructed the engineered bacterial plasmid pMG36e-GLP-1 containing the GLP-1 gene, which was subsequently transformed into Lactococcus lactis to generate the engineered strain MG1363-pMG36e-GLP-1. Administering MG1363-pMG36e-GLP-1 orally to mice afflicted with LPS-induced systemic inflammation led to decreased inflammation, substantial enhancements in spatial learning and memory, and suppression of glial cell activation and Aβ accumulation[110]. Subsequent investigations utilized MG1363-pMG36e-GLP-1 in a murine model of MPTP-induced PD, revealing significant enhancements in motor function, reduced dopaminergic neuron loss, diminished α-synuclein aggregation, and suppression of ferroptosis through activation of the Keap1/Nrf2/GPX4 pathway[111,112]. Moreover, MG1363-pMG36e-GLP-1 modulates the TLR4/NF-κB and AKT/GSK3β pathways to reduce the expression of inflammatory factors and boost neuroprotective factor activity, thereby notably improving spatial learning and memory in AD mice[8].
Potential applications of biosensors developed based on engineered bacteria for disease diagnosis
Engineered bacteria designed as biosensors represent an innovative approach in biotechnology, particularly for detecting pathogenic bacteria via their quorum sensing (QS) systems. QS involves bacterial communication via chemical signaling molecules, facilitating coordinated behavioral changes based on population density and surrounding species[113]. These biosensors utilize QS molecules as input modules, computational gene networks for processing, and output modules, such as reporter genes (e.g., β-galactosidase, fluorescent proteins, β-glucuronidase, β-lactamase), for detection [Figure 3].
For instance, researchers integrated the agrQS system from Staphylococcus aureus into Lactobacillus reuteri DSM20016 to detect autoinducing peptide I (AIP-I), a QS molecule produced by Staphylococcus aureus[114]. This engineered bacterium can sense AIP-I concentration-dependently by employing reporter genes such as GusA, which is linked to the production of a yellow pigment. Upon AIP-I binding to AgrC, it initiates AgrA phosphorylation, resulting in GusA expression repression and thereby indicating AIP-I presence indirectly through pigment production[115]. Li et al. expanded on this concept by engineering Lactobacillus plantarum WCSF I with an agrQS system for detecting AIP-I. Initially employing GFP as a reporter for fluorescence-based detection, they subsequently modified the system to secrete lysozyme upon AIP-I detection, creating a dual-function biosensor that detects and inhibits S. aureus growth[116].
Additionally, Mao et al. engineered Lactococcus lactis to detect Vibrio cholerae using a hybrid receptor HR capable of sensing V. cholerae’s population sensing molecule CAI-1. They utilized fluorescent and colorimetric reporter genes such as mCherry and β-lactamase for in vitro and in vivo detection, showcasing the versatility of engineered bacteria in diverse detection environments[117]. While the sex pheromone of certain Gram-negative bacteria can serve as a detection signal, Borrero et al. utilized the Enterococci sex pheromone, cCF10, to construct a pCF10-based expression vector introduced into Lactococcus lactis NZ9000 for detecting Enterococci presence[118]. The development of these biosensors illustrates the significant potential of engineered Lactococcus lactis in pathogenic bacteria monitoring.
It is evident that mere detection of pathogenic bacteria is insufficient; there is a desire to extend biosensor capabilities to detect biomarkers that hold greater significance in disease diagnosis. Biomarkers encompass various characteristics (such as cells, proteins, molecules, and genes) measurable from biological samples. Their presence or fluctuation can indicate the status or progression of specific diseases, playing pivotal roles in disease prevention, diagnosis, treatment, detection, and prognosis assessment[119]. This expectation has been partially realized in biosensors utilizing probiotics as chassis, exemplified by Mimee et al. They integrated hemoglobin-sensing, genetically modified EcN with ultra-low-power microelectronics to develop an ingestible micro-bio-electronic system (IMBED). This system detects luminescence signals from bacteria and wirelessly transmits them to external devices, effectively detecting gastrointestinal hemorrhage in mouse and pig models[120]. In the context of gut diseases like IBD, genetically engineered EcN, known as i-ROBOT, has been pivotal. Leveraging CRISPR-Cas9 gene editing technology, i-ROBOT detects inflammatory marker thiosulfate levels, expressing associated fluorescent proteins to diagnose IBD sensitively[121]. Similarly, calreticulin serves as another non-invasive IBD marker[122]. For tumor diagnostics, engineered EcN expressing tumor biomarker nitroreductase (NTR) has shown promise. Exploiting its natural tumor tropism, EcN stably expresses NTR in tumor tissues, activating fluorescent probes for tumor imaging, thereby aiding in tumor diagnosis and surgical resection[123]. Similarly, EcN was engineered to express β-galactosidase in tumor cells, which subsequently degrades the injected substrate LuGal, resulting in the production of fluorescein detectable in the urine[124]. These studies have demonstrated non-invasive, non-radioactive approaches for the early detection of tumors. These engineered bacteria are referred to as bacterial whole-cell biosensors (BWCBs)[125]. In the context of disease detection, BWCBs offer advantages such as reduced reliance on expensive equipment, faster detection, greater specificity, and lower costs, which facilitate the early diagnosis of certain diseases. Furthermore, engineered bacteria can be designed as imaging vectors to improve the sensitivity and specificity of medical imaging. Additionally, integrated diagnostic and therapeutic engineered bacteria are being developed to concurrently release therapeutic drugs while diagnosing disease markers[116].
Potential application of mucosal vaccines developed based on engineered bacteria in disease prevention
Mucosal surfaces in the respiratory, digestive, and genitourinary systems serve as crucial entry points for pathogens from the environment. The mucosal immune system plays a pivotal role as the body’s initial defense barrier against these pathogens. Therefore, effective vaccines that stimulate immune responses at mucosal sites are vital.
Research dating back to the early 1990s has underscored the potential of Lactobacillus casei in enhancing mucosal immune responses. For example, studies have shown that Lactobacillus casei can protect against Salmonella typhimurium infection by boosting the production of sIgA in intestinal mucosal secretions[126]. This highlights the ability of natural Lactobacillus casei to serve as an immune adjuvant for preventing intestinal infections.
Recent advancements in probiotic genomics and synthetic biology have significantly advanced the design of engineered bacteria. These probiotics are engineered to express antigenic components from various pathogens such as bacteria[127], viruses[128], and parasites[129]. Administering these engineered bacteria mucosally can stimulate immune responses within the mucosal immune system, thereby exerting anti-infective effects targeted against specific pathogens.
Several recent animal studies have demonstrated the superior immunoprotective effects of engineered bacteria against pathogens such as Streptococcus pneumoniae[127], Trichinella spiralis[129], Helicobacter pylori[130,131], Avian influenza A virus[132], and COVID-19[133]. Various factors influence the immunization efficacy of engineered bacteria, including chassis type, antigen expression site, administration route, and target antigen type. A study examined the impact of varying antigen anchoring positions on immunization efficacy. They introduced two plasmids, pPG1 (surface display) and pPG2 (secretion), into the probiotic strain Lactobacillus casei CC16 for oral administration to carp, aiming to stimulate mucosal immune responses against Aeromonas hydrophilia. They discovered that surface-displayed Lc-pPG1-Aha1 induced higher levels of specific antibodies and enhanced leukocyte phagocytosis[134]. Additionally, related studies have explored different administration routes. For instance, in a study by Zhang et al., the peptide hormone IP-673 promoted the use of recombinant probiotic strain L. plantarum strains expressing the HA1 protein, which were administered both orally and intranasally. Mice challenged with a lethal dose of the H1N1 virus showed improved immunization when the administration was intranasal[135]. Huynh et al. demonstrated HA1 subunit and Bacillus subtilis poly gamma-glutamate synthetase A (pgsA) fusion proteins on the surface of Lactobacillus casei L525 and they compared immune response intensities between intranasal and oral administration routes, finding both routes provided similar immune protection, with intranasal administration proving more effective. Several factors, including genetic diversity among Lactobacilli[136], antigen characteristics, and mucosal immunization sites, complicate the interpretation of immune outcomes and hinder comparative studies. Table 1 provides a summary of selected probiotics engineered for use as mucosal vaccines [Table 1].
Some probiotics are designed as mucosal vaccines
Strains | Expression vector | Route of vaccination | Targeting pathogens | Antigen | Response method | Ref. |
Lactococcus lactis ATCC 11454 | pMG36e | Oral | Clostridioides difficile | Non-toxic C-terminal receptor binding domains of the toxins TcdA and TcdB | The immunized mice all produced high levels of IgG and IgA antibodies | [137] |
Lactococcus lactis F17847 | pTnis | Endonasal | Streptococcus pneumoniae | PspA | Associated with a shift to a Th1-mediated immune response characterized by reduced PspA antibody potency | [127] |
Lactococcus lactis NZ3900 | pNZ8110 | Oral | Helicobacter pylori | Lpp20 antigen | Significantly elevated serum Lpp20-specific IgG antibody levels | [130] |
Lactobacillus plantarum NC8 | pSIP409 | Oral | Trichinella, Trichinella spiralis | Tsgal | Triggered significant intestinal mucosal sIgA responses and specific systemic Th1/Th2 immune responses | [129] |
Lactococcus lactis NZ9000 | pNZ8148-SAM,plSAM-WAE | Oral | Helicobacter pylori | Urease, HpaA, HSP60, NAP | Showed increased levels of antibodies against Helicobacter pylori, including IgG and sIgA, and significantly reduced Helicobacter pylori colonization | [131] |
EcN | pNZ8148 | Oral, endonasal | Birch and pollen allergens | Bet v 1 Phl p 1, Phl p 5 | Intra-application of EcN-Chim significantly reduced lung inflammation, decreased specific IgE levels, and increased specific IgA and IgG2a levels | [138] |
EcN | pEHLYA2-SD | Oral, rectal | HIV | gp41 protein | Secretion of C52-HlyA218 peptide inhibits HIV infection of human peripheral blood mononuclear cells in vitro | [139] |
EcN ΔdapB | pKT-5M2e-HlyABD | Endonasal | Influenza A virus | 5M2e antigen | EcN-5M2e induces effective humoral, mucosal, and T cell responses | [140] |
Viral infections like high-risk HPV-16 are closely associated with tumorigenesis, implying that recombinant Lactobacillus vaccines targeting these viruses may exert antitumor effects. This hypothesis was validated in an experimental animal study conducted by Mohseni et al.[141]. They utilized the vector pNZ8123-HPV16-optiE7 to express the HPV-16 E7 antigen in the Lactococcus lactis NZ9000 strain, which was orally administered to female mice. This approach induced high levels of E7-specific antibodies, along with a robust presence of E7-specific CD4+ T helper cells and CD8+ T cell precursors, thereby demonstrating potent protection against an E7-expressing tumor cell line (TC-1)[141]. In a related study, Li et al. engineered Lactococcus lactis to co-express HPV-16 E7 protein and IL-12, thereby enhancing mucosal immune activation through intranasal administration. IL-12 functions as an immune adjuvant, stimulating a Th1-type immune response and enhancing IFN-γ production, which in turn boosts cytotoxic T-lymphocyte (CTL) activity and enhances tumor cell killing[142].
In addition to probiotic Lactococcus lactis, EcN has demonstrated significant potential for mucosal vaccine development. As early as the last century, the non-fimbrial adhesin AIDA-I associated with diffuse adhesion of enteropathogenic Escherichia coli (EPEC) was successfully cloned and expressed, contributing to the AIDA autotransporter system[143]. Subsequent studies explored integrating this system into EcN’s cell membrane using synthetic biology, incorporating virulence factor antigens to display heterologous polypeptides on the bacterial surface. This approach aims to activate the host mucosal immune system upon mucosal administration, potentially producing immunoprotective effects[144]. On this basis, the plasmid pAIDA1-SP, containing the SARS-CoV-2 spike protein (SP) gene, was designed and transfected into EcN. This innovation resulted in the development of a mucosal vaccine against COVID-19, which induced time-dependent increases in specific IgG and IgA antibodies in mice following both oral and intranasal administration[133,145]. Antigen presentation on the bacterial surface, facilitated by an AIDA autotransporter or an anchoring matrix, is crucial as it enhances stimulation of the local mucosa-associated lymphoid tissue (lamina propria). In comparable oral administration settings, engineered Lactobacillus casei utilized PgsA as an anchoring matrix to fuse K99 and K88 fimbrial proteins, achieving higher levels of specific IgA and IgG antibodies and eliciting effective T cell immune responses. This outperformance contrasts with engineered EcN, where the plasmid was directly inserted into the K88 fimbrial adhesin gene[146,147]. In both studies, Lactobacillus casei demonstrated higher immunogenicity compared to EcN, highlighting that the choice of chassis bacteria can significantly impact the efficacy of mucosal vaccines.
Advantages and deficiencies of engineered bacteria in disease treatment
Engineered bacteria play a pivotal role as carriers in drug delivery systems, providing a promising platform to enhance the precise delivery of drugs or target molecules to lesions for targeted therapy. This development is particularly significant in treating hypoxic solid tumors such as melanoma, where conventional radiotherapy and chemotherapy often struggle due to their low tumor-targeting efficiency and limited tissue penetration. Engineered attenuated Salmonella typhimurium, capitalizing on its facultative anaerobic nature, exhibits a remarkable ability to target and colonize hypoxic tumor tissues. Moreover, releasing mPD-1 modulates immunity, leading to a substantial reduction in tumor volume[97]. Additionally, strategies such as surface modification, ecological niche competition, and genetic engineering further augment the efficacy of these targeting approaches[148].
In the treatment of IBD, conventional high-dose administration of anti-inflammatory factors such as IL-10, IL-27, or IL-35 is expensive and often results in significant systemic side effects[149]. However, engineered Lactococcus lactis can locally produce IL-10 in the intestine upon topical application, effectively reducing systemic side effects associated with high-dose treatments[35]. Moreover, probiotics themselves can modulate intestinal microecology and promote mucosal repair, thereby enhancing the effectiveness of engineered bacteria[150]. Engineered bacteria also address challenges related to the short half-life of certain drug molecules. For example, in the treatment of neurodegenerative diseases and diabetes mellitus, engineered EcN and Lactobacillus lactis can consistently and efficiently express GLP-1, which reduces drug dosage, minimizes side effects, and improves patient adherence[111,151]. Furthermore, patients with PKU often face a reduced quality of life due to strict Phe restrictions. Traditional treatments involve daily subcutaneous injections of recombinant PAL, which are effective in some patients but can induce severe allergic reactions[152]. Engineered bacterium SYNB1618, expressing PAL, presents a promising solution with demonstrated safety and tolerability in clinical trials[76].
While natural and engineered bacteria hold promise for future disease treatments, studies have highlighted safety concerns associated with these live drugs. Engineered bacterial therapies must address both biosafety and ethical concerns to ensure that live therapeutic bacteria do not spread or undergo unintended recombination in the environment. Antibiotic resistance genes can be carried on mobile genetic elements such as plasmids and transposons, facilitating their exchange through conjugative transfer between bacteria. This horizontal transfer of resistance genes poses a significant risk. For instance, vancomycin resistance genes have been observed to transfer from Enterococci to Lactobacillus acidophilus even without antibiotic selection pressure, potentially occurring within the human gastrointestinal tract[153]. In a study assessing eight commercially available probiotic preparations containing Lactobacillus acidophilus, various resistance genes - including those for vancomycin, ciprofloxacin, broad-spectrum β-lactamases, and tetracycline - were detected[154]. Without stringent screening, these probiotics could inadvertently serve as vectors for antibiotic resistance genes.
When developing engineered bacteria using plasmid expression systems, the introduction of plasmids carrying exogenous DNA into host bacteria typically involves screening for successful transformation using antibiotic resistance genes. However, the inclusion of these resistance genes in engineered bacteria poses a barrier to their clinical application. To address this issue, researchers are exploring alternative selection markers such as sugar utilization genes, phage resistance factors, and stress tolerance mechanisms. Additionally, emerging gene editing technologies like the CRISPR-Cas system offer a promising avenue for genome editing in bacteria, enabling the creation of antibiotic-free engineered bacteria[155]. Currently, most synthetic biology tools are primarily developed for EcN and Lactobacillus, with relatively few engineering tools available for strict anaerobic bacteria found in the colon[156]. Additionally, the regulatory framework for engineered bacteria as novel therapeutics remains incomplete, and regulatory requirements may vary across countries and regions, presenting a challenge for global clinical trials and product launches.
SYNTHETIC BIOLOGY AND CRISPR/CAS SYSTEM
The synthesis of proteins and DNA, combined with the establishment of biocentric laws, laid the foundation for the idea of creating living organisms from scratch, leading to the rapid development of synthetic biology. Synthetic biology is an interdisciplinary field that integrates concepts and techniques from biology, engineering, computer science, and molecular biology. It is typically defined as the redesign or engineering of existing biological components and systems using specific technologies to achieve a desired function[157]. In 2010, Gibson et al. created the first synthetic genomic cell, employing a bottom-up approach in which chemically synthesized DNA fragments were assembled to form a minimal genome necessary for the basic functioning of an organism[158]. This minimal genome offers advantages in stability and efficiency, but challenges remain, such as the difficulty of synthesizing long DNA sequences from scratch[159]. In contrast, the field of engineered bacteria often adopts a top-down strategy, which involves modifying existing organisms by removing non-essential genes from their genomes. This process frees up space and energy to optimize the expression of target genes[160]. For example, studies have shown that heterologous protein production in Lactobacillus lactis NZ9000 is significantly improved when 2.83% of the non-essential genome is deleted using the Cre-loxP deletion system[161]. Plasmid-based expression systems are commonly used in engineered bacteria, but they face limitations for clinical applications due to issues with genetic instability and the potential for biological contamination. As a result, gene-editing technologies, particularly those based on the CRISPR-Cas system, are emerging as powerful tools. These technologies have the potential to stabilize and modify probiotic genomes, enabling the alteration of their metabolic pathways and biological properties for more precise and reliable applications.
The CRISPR/Cas system is an acquired immune mechanism found in most archaea and approximately half of all bacteria, where it functions to recognize and excise invading viral DNA[162]. This system consists of two main components: the sequence responsible for encoding Cas-related proteins and the CRISPR array, which contains a series of repeats interspersed with spacer sequences. The spacer sequences store fragments of foreign genetic material, allowing the organism to “remember” previous invaders[163]. The mechanism operates through three main stages: Adaptation: The complex formed by the Cas1 and Cas2 proteins recognizes foreign genetic material and integrates it into the spacer sequences of the CRISPR array. Expression: The CRISPR array is transcribed into pre-crRNA, which is then processed into mature crRNA by Cas proteins. Interference: The crRNA binds to the Cas protein to form a complex that identifies and cleaves foreign DNA sequences that are complementary to the spacer sequence[164]. This recognition occurs only when the crRNA binds to the protospacer adjacent motif (PAM) sequence, a short DNA motif located adjacent to the target sequence[165]. Based on this mechanism, the CRISPR/Cas system has been developed into a powerful tool for gene editing. Unlike conventional technologies such as zinc finger nucleases (ZFNs) and transcription activator-like effector nucleases (TALENs), which rely on protein-DNA recognition, CRISPR/Cas utilizes base-pairing between complementary RNA and DNA to target specific sequences. This makes it more accurate and efficient[166]. Furthermore, CRISPR/Cas technology only requires the design of guide RNAs (gRNAs) to target specific DNA sequences, making it simpler than designing the specific proteins needed for ZFNs and TALENs[167]. Once the designed gRNA-Cas complex creates a DNA double-strand break (DSB) at the target site, the host cell can repair the break through either the non-homologous end joining (NHEJ) or homology-directed repair (HDR) pathways[168]. Recently, DSB-independent CRISPR/Cas technologies have also been developed, including base editing (BE), prime editing (PE), and CRISPR-associated transposases (CAST), which offer more precise methods for genome modification without causing DSBs[169-171].
Unlike plasmid-based overexpression systems, CRISPR/Cas gene editing allows for precise deletions, additions, and replacements of a strain’s genome, offering a solution to the genetic instability associated with plasmids and the contamination from antibiotic resistance genes. This approach is poised to become a focal point in the development of engineered probiotics. We first examined the application of CRISPR/Cas technology in two major genera of probiotics - Lactobacillus and Bifidobacterium. Our genomic analysis revealed that CRISPR repeats were present in 59.7% of Lactobacillus genomes and 57% of Bifidobacterium genomes[172,173]. Specific subtypes and sequences were identified, laying the groundwork for future gene editing efforts. For example, Song et al. designed a plasmid, pLCNICK, which carries the Cas9D10A enzyme and a sgRNA expression cassette, along with the homology arms of the target gene. This plasmid was introduced into Lactobacillus casei via electrotransformation. Upon guiding Cas9D10A to induce a double-stranded break at the target site, homologous recombination repair allowed for the replacement or knockout of the target gene. The efficiency of this gene knockout ranged from 25% to 62%[174]. Alternatively, gene knockout can be achieved by obstructing RNA polymerase function with sgRNAs and partially inactivated dCas9 (which cannot cleave DNA), thus creating a physical barrier to transcription[175].
Endogenous CRISPR/Cas systems, in contrast to exogenous ones, offer higher specificity and lower cytotoxicity, which could make them more suitable for use in probiotics[176]. Numerous endogenous CRISPR/Cas systems are being developed for application in probiotics. As an example, researchers reprogrammed the endogenous type I-G CRISPR-Cas system in Bifidobacterium animalis subsp. lactis to identify naturally occurring large deletions and generate a 500-bp deletion in the tetW gene, thereby eliminating tetracycline resistance[173]. The translation of uridine phosphate ribose transferase in Bifidobacterium breve FJSWX38M7 was successfully terminated by a single base substitution and the insertion of three stop codons, using the endogenous Type I-C CRISPR-Cas system of Bifidobacterium breve[177]. The endogenous Type I-E CRISPR-Cas system was identified in Lactobacillus crispatus NCK1350, with Cas3 as its signature protein. After identifying 5’-AAA-3’ as the PAM sequence, the researchers designed the pTRK1183 plasmid containing the specific crRNA. This plasmid successfully facilitated gene deletion (100% efficiency), stop codon insertion (36% efficiency), and single nucleotide substitution (19% efficiency) in Lactobacillus crispatus NCK1350[176]. Although CRISPR/Cas-based gene editing is still in its developmental phase, significant progress is being made, and several tools are under development. Notably, there are few reports on the direct introduction of large heterologous protein segments into probiotic genomes. A recent study, however, demonstrated successful expression of the HIV-1 membrane-proximal external region (MPER) on the surface of EcN using CRISPR/Cas9. This breakthrough suggests the potential of engineered probiotics to enhance mucosal immune responses[178]. As research into CRISPR/Cas continues, it will deepen our understanding of the genetic characteristics of probiotics and open new avenues for their application. Table 2 summarizes the application of CRISPR-Cas-based gene editing technology in some probiotics [Table 2].
CRISPR-Cas system in some probiotics
Probiotics | Gene editing tools | Application | Ref. |
Lactococcus lactis NZ9000 | Cas9 protein, sgRNA, Red/ET recombinase system, pMG36e plasmid | Constructed pMG-Cas9-ldh recombinant plasmid to knock out lactate dehydrogenase gene; developed a food-grade gene editing system to avoid the introduction of antibiotics and exogenous genes | [179] |
Lactobacillus rhamnosus GG | Cas9, sgRNA, HMME, ZIF-8 | Self-driving CRISPR/Cas9 nanosystems can reprogram TIME through multiple pathways | [180] |
Lactobacillus paracasei NCBIO01-M2 | Cas9, sgRNA, pNcas | Obtained a thermotolerant strain NCBIO01-M2-ldhL1-HT capable of efficiently producing L-lactic acid at 45 ℃ | [181] |
Lactobacillus reuteri ATCC PTA 6475 | Cas9, tracrRNA, RecT, crRNA, pVPL3017, pVPL3004 | CRISPR-Cas9-assisted recombination techniques can facilitate targeted codon saturation mutations. Additionally, CRISPR-Cas9-based selection enables the identification of mutations that occur at low frequencies, such as those resulting from oligonucleotide-mediated chromosomal deletions | [182] |
Bifidobacterium animalis subsp. lactis | CRISPR-CBE | A large segment deletion was produced in Bifidobacterium animalis subsp. lactis using the endogenous CRISPR-Cas system; the CRISPR-cytosine base editor was able to introduce SNPs in B. lactis | [173] |
EcN | Cas3, crRNA, λ-RED recombination system, cascade protein | Engineered EcN is effective in removing multiple ARGs under in vitro and in vivo conditions | [183] |
EcN | Cas9, sgRNA, pCas9-KT | Removal of two cryptic plasmids, pMUT1 and pMUT2, from EcN reduces metabolic burden; used for GABA production in an antibiotic-free system | [184] |
EcN | CRISPR-Cas9 plasmid, pDA, ROS-responsive linker | For targeted delivery of the CRISPR-Cas9 system to deep-seated tumors for photothermal sensitization immunotherapy, liposomes (Lipo-P) loaded with CRISPR-Cas9 plasmid were used to reduce the thermotolerance of tumor cells through gene editing | [185] |
Advances in artificial intelligence (AI) are expected to significantly impact research in the field of engineered bacteria, particularly in the areas of editing, screening, and optimizing engineered strains. For instance, the development of the GEDpm-cg platform offers an efficient, user-friendly, and flexible tool for genome editing in C. glutamicum, which is anticipated to enable large-scale mutation analysis through robot- and software-assisted systems[186]. This platform aims to improve the understanding and engineering of cellular metabolism. Moreover, structural biology plays a key role in the optimization of gene editing tools. In particular, understanding the high-resolution structure of nucleases such as Cas9 is crucial for elucidating how these enzymes recognize and cleave DNA. By analyzing the structure of Cas9, modifications can be made to enhance its specificity and reduce off-target effects.
CONCLUSION
Natural probiotics have demonstrated beneficial properties such as improving intestinal microbiota, regulating metabolism, and exhibiting antitumor effects. However, nonspecific probiotic supplementation and FMT may lead to variable efficacy and potential safety concerns, making the development of engineered bacteria for more targeted treatments a more sensible approach. Leveraging synthetic biology, researchers are genetically engineering these probiotics to carry out specific functions beneficial to human health. Engineered bacteria show promising potential for treating conditions such as IBD, metabolic disorders, neurodegenerative diseases, and cancer. Despite successful results in animal models, clinical trials are limited, with few products like SYNB1618 demonstrating safety and tolerability[76]. None have yet been approved for market use. Advancements in gene editing tools enable the design of complex genetic circuits to finely tune the biological capabilities of engineered bacteria. This includes enhancing their targeting specificity[187] and optimizing the secretion of desired products[188]. Despite their therapeutic promise, challenges remain, such as improving safety profiles, refining clinical trial methodologies, and advancing technological innovations. Exploring new applications within the realm of synthetic biology offers avenues for further development and application. In the future, advanced technologies emerging from synthetic biology, structural biology, and AI are expected to significantly contribute to research in the field of engineered bacteria.
DECLARATIONS
Acknowledgments
The authors greatly appreciate https://scidraw.io/, https://www.vecteezy.com/, and https://BioRender.com, three excellent sites that provide authors with some image material.
Authors’ contributions
Literature searching and manuscript writing: Luo Z, Qi Z
Article revisions: Qi Z, Chen T
Theme formulation and procedure supervision: Luo Z, Qi Z, Luo J, Chen T
All authors have checked and agreed to the submitted version.
Availability of data and materials
Not applicable.
Financial support and sponsorship
None.
Conflicts of interest
Chen T is a Junior Editorial Board member of the journal Microbiome Research Reports. Chen T was not involved in any steps of editorial processing, notably including reviewers’ selection, manuscript handling, and decision-making. The other authors declare that there are no conflicts of interest.
Ethical approval and consent to participate
Not applicable.
Consent for publication
Not applicable.
Copyright
© The Author(s) 2024.
REFERENCES
1. Society of The Chinese Institute of Food Science A, Technology. Scientific consensus on probiotics (2020). J Chin Inst Food Sci Technol 2020;20:303-7.
2. Suez J, Zmora N, Segal E, Elinav E. The pros, cons, and many unknowns of probiotics. Nat Med 2019;25:716-29.
3. Chowdhury S, Castro S, Coker C, Hinchliffe TE, Arpaia N, Danino T. Programmable bacteria induce durable tumor regression and systemic antitumor immunity. Nat Med 2019;25:1057-63.
5. Gurbatri CR, Lia I, Vincent R, et al. Engineered probiotics for local tumor delivery of checkpoint blockade nanobodies. Sci Transl Med 2020;12:eaax0876.
6. Li M, Liu N, Zhu J, et al. Engineered probiotics with sustained release of interleukin-2 for the treatment of inflammatory bowel disease after oral delivery. Biomaterials 2024;309:122584.
7. Hu H, Luo J, Liu Y, et al. Improvement effect of a next-generation probiotic L. plantarum-pMG36e-GLP-1 on type 2 diabetes mellitus via the gut-pancreas-liver axis. Food Funct 2023;14:3179-95.
8. Fang X, Zhou X, Miao Y, Han Y, Wei J, Chen T. Therapeutic effect of GLP-1 engineered strain on mice model of Alzheimer’s disease and Parkinson’s disease. AMB Express 2020;10:80.
9. Gurbatri CR, Radford GA, Vrbanac L, et al. Engineering tumor-colonizing E. coli Nissle 1917 for detection and treatment of colorectal neoplasia. Nat Commun 2024;15:646.
10. Britton RA, Hoffmann DE, Khoruts A. Probiotics and the microbiome-how can we help patients make sense of probiotics? Gastroenterology 2021;160:614-23.
11. Yadav AK, Tyagi A, Kumar A, et al. Adhesion of Lactobacilli and their anti-infectivity potential. Crit Rev Food Sci Nutr 2017;57:2042-56.
12. Breukink E, Wiedemann I, van Kraaij C, Kuipers OP, Sahl HG, de Kruijff B. Use of the cell wall precursor lipid II by a pore-forming peptide antibiotic. Science 1999;286:2361-4.
13. Hols P, Ledesma-García L, Gabant P, Mignolet J. Mobilization of microbiota commensals and their bacteriocins for therapeutics. Trends Microbiol 2019;27:690-702.
14. Tiwari SK. Bacteriocin-producing probiotic lactic acid bacteria in controlling dysbiosis of the gut microbiota. Front Cell Infect Microbiol 2022;12:851140.
15. Bender MJ, McPherson AC, Phelps CM, et al. Dietary tryptophan metabolite released by intratumoral Lactobacillus reuteri facilitates immune checkpoint inhibitor treatment. Cell 2023;186:1846-62.e26.
16. Martin FP, Cominetti O, Berger B, et al. Metabolome-associated psychological comorbidities improvement in irritable bowel syndrome patients receiving a probiotic. Gut Microbes 2024;16:2347715.
17. Srivastava S, Basak U, Naghibi M, et al. A randomized double-blind, placebo-controlled trial to evaluate the safety and efficacy of live Bifidobacterium longum CECT 7347 (ES1) and heat-treated Bifidobacterium longum CECT 7347 (HT-ES1) in participants with diarrhea-predominant irritable bowel syndrome. Gut Microbes 2024;16:2338322.
18. Zhang Q, Li G, Zhao W, et al. Efficacy of Bifidobacterium animalis subsp. lactis BL-99 in the treatment of functional dyspepsia: a randomized placebo-controlled clinical trial. Nat Commun 2024;15:227.
19. Han Y, Zhou Y, Xu X, et al. Improvement of post-surgery constipation in patients with fractures by Lactobacillus rhamnosus JYLR-127: a single-blind randomized controlled trial. Nutrients 2024;16:1505.
20. Koyama S, Fujita H, Shimosato T, et al; Yokohama Cooperative Study Group for Hematology (YACHT). Septicemia from Lactobacillus rhamnosus GG, from a probiotic enriched yogurt, in a patient with autologous stem cell transplantation. Probiotics Antimicro Prot 2019;11:295-8.
21. Rossi F, Amadoro C, Gasperi M, Colavita G. Lactobacilli infection case reports in the last three years and safety implications. Nutrients 2022;14:1178.
22. Kassam Z, Dubois N, Ramakrishna B, et al. Donor screening for fecal microbiota transplantation. N Engl J Med 2019;381:2070-2.
23. Nasrollahzadeh A, Mokhtari S, Khomeiri M, Saris PEJ. Antifungal preservation of food by lactic acid bacteria. Foods 2022;11:395.
24. Zuo F, Zeng Z, Hammarström L, Marcotte H. Inducible plasmid self-destruction (IPSD) assisted genome engineering in lactobacilli and bifidobacteria. ACS Synth Biol 2019;8:1723-9.
25. Bober JR, Beisel CL, Nair NU. Synthetic biology approaches to engineer probiotics and members of the human microbiota for biomedical applications. Annu Rev Biomed Eng 2018;20:277-300.
26. Wu J, Xin Y, Kong J, Guo T. Genetic tools for the development of recombinant lactic acid bacteria. Microb Cell Fact 2021;20:118.
27. Seyedian SS, Nokhostin F, Malamir MD. A review of the diagnosis, prevention, and treatment methods of inflammatory bowel disease. J Med Life 2019;12:113-22.
28. Kaplan GG. The global burden of IBD: from 2015 to 2025. Nat Rev Gastroenterol Hepatol 2015;12:720-7.
29. Alipour M, Zaidi D, Valcheva R, et al. Mucosal barrier depletion and loss of bacterial diversity are primary abnormalities in paediatric ulcerative colitis. J Crohns Colitis 2016;10:462-71.
30. Danne C, Skerniskyte J, Marteyn B, Sokol H. Neutrophils: from IBD to the gut microbiota. Nat Rev Gastroenterol Hepatol 2024;21:184-97.
31. Smith PM, Howitt MR, Panikov N, et al. The microbial metabolites, short-chain fatty acids, regulate colonic Treg cell homeostasis. Science 2013;341:569-73.
32. Lautenschläger C, Schmidt C, Fischer D, Stallmach A. Drug delivery strategies in the therapy of inflammatory bowel disease. Adv Drug Deliv Rev 2014;71:58-76.
33. Yasmin F, Najeeb H, Shaikh S, et al. Novel drug delivery systems for inflammatory bowel disease. World J Gastroenterol 2022;28:1922-33.
34. Steidler L, Hans W, Schotte L, et al. Treatment of murine colitis by Lactococcus lactis secreting interleukin-10. Science 2000;289:1352-5.
35. Steidler L, Neirynck S, Huyghebaert N, et al. Biological containment of genetically modified Lactococcus lactis for intestinal delivery of human interleukin 10. Nat Biotechnol 2003;21:785-9.
36. Braat H, Rottiers P, Hommes DW, et al. A phase I trial with transgenic bacteria expressing interleukin-10 in Crohn’s disease. Clin Gastroenterol Hepatol 2006;4:754-9.
37. Jiang R, Yuan S, Zhou Y, et al. Strategies to overcome the challenges of low or no expression of heterologous proteins in Escherichia coli. Biotechnol Adv 2024;75:108417.
38. Plotkin JB, Kudla G. Synonymous but not the same: the causes and consequences of codon bias. Nat Rev Genet 2011;12:32-42.
39. Hanson ML, Hixon JA, Li W, et al. Oral delivery of IL-27 recombinant bacteria attenuates immune colitis in mice. Gastroenterology 2014;146:210-21.e13.
40. Zhou J, Li M, Chen Q, et al. Programmable probiotics modulate inflammation and gut microbiota for inflammatory bowel disease treatment after effective oral delivery. Nat Commun 2022;13:3432.
41. Chen H, Lei P, Ji H, et al. Escherichia coli Nissle 1917 ghosts alleviate inflammatory bowel disease in zebrafish. Life Sci 2023;329:121956.
42. Qin J, Li Y, Cai Z, et al. A metagenome-wide association study of gut microbiota in type 2 diabetes. Nature 2012;490:55-60.
43. Zhou W, Sailani MR, Contrepois K, et al. Longitudinal multi-omics of host-microbe dynamics in prediabetes. Nature 2019;569:663-71.
45. Rittiphairoj T, Pongpirul K, Janchot K, Mueller NT, Li T. Probiotics contribute to glycemic control in patients with type 2 diabetes mellitus: a systematic review and meta-analysis. Adv Nutr 2021;12:722-34.
46. Wang Y, Dilidaxi D, Wu Y, Sailike J, Sun X, Nabi XH. Composite probiotics alleviate type 2 diabetes by regulating intestinal microbiota and inducing GLP-1 secretion in db/db mice. Biomed Pharmacother 2020;125:109914.
47. Holst JJ. Glucagonlike peptide 1: a newly discovered gastrointestinal hormone. Gastroenterology 1994;107:1848-55.
48. Sandoval DA, D’Alessio DA. Physiology of proglucagon peptides: role of glucagon and GLP-1 in health and disease. Physiol Rev 2015;95:513-48.
49. Grandl G, Novikoff A, Dimarchi R, Tschöp MH, Müller TD. Gut peptide agonism in the treatment of obesity and diabetes. Compr Physiol 2019;10:99-124.
50. Agarwal P, Khatri P, Billack B, Low WK, Shao J. Oral delivery of glucagon like peptide-1 by a recombinant Lactococcus lactis. Pharm Res 2014;31:3404-14.
51. Luo J, Zhang H, Lu J, Ma C, Chen T. Antidiabetic effect of an engineered bacterium Lactobacillus plantarum-pMG36e -GLP-1 in monkey model. Synth Syst Biotechnol 2021;6:272-82.
52. Wang L, Chen T, Wang H, et al. Engineered bacteria of MG1363-pMG36e-GLP-1 attenuated obesity-induced by high fat diet in mice. Front Cell Infect Microbiol 2021;11:595575.
53. Takiishi T, Korf H, Van Belle TL, et al. Reversal of autoimmune diabetes by restoration of antigen-specific tolerance using genetically modified Lactococcus lactis in mice. J Clin Invest 2012;122:1717-25.
54. Chavkin TA, Pham LD, Kostic A. E. coli Nissle 1917 modulates host glucose metabolism without directly acting on glucose. Sci Rep 2021;11:23230.
55. Bazi Z, Jalili M, Hekmatdoost A. The long term oral regulation of blood glucose in diabetic patients by using of Escherichia coli Nissle 1917 expressing CTB-IGF-1 hybrid protein. Med Hypotheses 2013;81:961-2.
56. Tu P, Ma Z, Wang H, et al. Expression of CTB-10×rolGLP-1 in E. coli and its therapeutic effect on type 2 diabetes. Curr Pharm Biotechnol 2015;16:564-72.
57. Qin Q, Chen Y, Li Y, et al. Intestinal microbiota play an important role in the treatment of type I diabetes in mice with BefA protein. Front Cell Infect Microbiol 2021;11:719542.
58. Wang H, Wei J, Hu H, et al. Oral administration of bacterial β cell expansion factor A (BefA) alleviates diabetes in mice with type 1 and type 2 diabetes. Oxid Med Cell Longev 2022;2022:9206039.
59. Blau N, Hennermann JB, Langenbeck U, Lichter-Konecki U. Diagnosis, classification, and genetics of phenylketonuria and tetrahydrobiopterin (BH4) deficiencies. Mol Genet Metab 2011;104 Suppl:S2-9.
60. Spronsen FJ, Blau N, Harding C, Burlina A, Longo N, Bosch AM. Phenylketonuria. Nat Rev Dis Primers 2021;7:36.
61. Lichter-Konecki U, Vockley J. Phenylketonuria: current treatments and future developments. Drugs 2019;79:495-500.
62. van Spronsen FJ, van Wegberg AM, Ahring K, et al. Key European guidelines for the diagnosis and management of patients with phenylketonuria. Lancet Diabetes Endocrinol 2017;5:743-56.
63. Martinez M, Harding CO, Schwank G, Thöny B. State-of-the-art 2023 on gene therapy for phenylketonuria. J Inherit Metab Dis 2024;47:80-92.
64. Kim W, Erlandsen H, Surendran S, et al. Trends in enzyme therapy for phenylketonuria. Mol Ther 2004;10:220-4.
65. Levy HL, Sarkissian CN, Scriver CR. Phenylalanine ammonia lyase (PAL): from discovery to enzyme substitution therapy for phenylketonuria. Mol Genet Metab 2018;124:223-9.
66. Durrer KE, Allen MS, Hunt von Herbing I. Genetically engineered probiotic for the treatment of phenylketonuria (PKU); assessment of a novel treatment in vitro and in the PAHenu2 mouse model of PKU. PLoS One 2017;12:e0176286.
67. Ramírez AM, Rodriguez-López A, Ardila A, et al. Production of human recombinant phenylalanine hydroxylase in Lactobacillus plantarum for gastrointestinal delivery. Eur J Pharm Sci 2017;109:48-55.
68. Phumkhachorn P, Rattanachaikunsopon P. A broad host range food-grade cloning vector for lactic acid bacteria. Biologia 2016;71:457-63.
69. Kaur T, Balgir PP, Kaur B. Correction to: construction of a shuttle expression vector for lactic acid bacteria. J Genet Eng Biotechnol 2020;18:38.
70. Platteeuw C, van Alen-Boerrigter I, van Schalkwijk S, de Vos WM. Food-grade cloning and expression system for Lactococcus lactis. Appl Environ Microbiol 1996;62:1008-13.
71. Kim JH, Mills DA. Improvement of a nisin-inducible expression vector for use in lactic acid bacteria. Plasmid 2007;58:275-83.
72. Chae JP, Pajarillo EA, Hwang IC, Kang DK. Construction of a Bile-responsive expression system in Lactobacillus plantarum. Food Sci Anim Resour 2019;39:13-22.
73. Guan C, Yuan Y, Ma Y, et al. Development of a novel expression system in lactic acid bacteria controlled by a broad-host-range promoter PsrfA. Microb Cell Fact 2022;21:23.
74. Isabella VM, Ha BN, Castillo MJ, et al. Development of a synthetic live bacterial therapeutic for the human metabolic disease phenylketonuria. Nat Biotechnol 2018;36:857-64.
75. Jiang Y, Sun B, Qian F, et al. Expression of phenylalanine ammonia lyase as an intracellularly free and extracellularly cell surface-immobilized enzyme on a gut microbe as a live biotherapeutic for phenylketonuria. Sci China Life Sci 2023;66:127-36.
76. Puurunen MK, Vockley J, Searle SL, et al. Safety and pharmacodynamics of an engineered E. coli Nissle for the treatment of phenylketonuria: a first-in-human phase 1/2a study. Nat Metab 2021;3:1125-32.
77. Adolfsen KJ, Callihan I, Monahan CE, et al. Improvement of a synthetic live bacterial therapeutic for phenylketonuria with biosensor-enabled enzyme engineering. Nat Commun 2021;12:6215.
78. Triassi AJ, Fields BD, Monahan CE, et al. Redesign of an Escherichia coli Nissle treatment for phenylketonuria using insulated genomic landing pads and genetic circuits to reduce burden. Cell Syst 2023;14:512-24.e12.
79. Heineman HS, Jensen WN, Cooper WM, Braude AI. Hodgkin’s disease and salmonella typhimurium infection. JAMA 1964;188:632-4.
80. Stritzker J, Weibel S, Hill PJ, Oelschlaeger TA, Goebel W, Szalay AA. Tumor-specific colonization, tissue distribution, and gene induction by probiotic Escherichia coli Nissle 1917 in live mice. Int J Med Microbiol 2007;297:151-62.
81. Toso JF, Gill VJ, Hwu P, et al. Phase I study of the intravenous administration of attenuated Salmonella typhimurium to patients with metastatic melanoma. J Clin Oncol 2002;20:142-52.
82. Chondrou P, Karapetsas A, Kiousi DE, et al. Lactobacillus paracasei K5 displays adhesion, anti-proliferative activity and apoptotic effects in human colon cancer cells. Benef Microbes 2018;9:975-83.
83. Leschner S, Westphal K, Dietrich N, et al. Tumor invasion of Salmonella enterica serovar Typhimurium is accompanied by strong hemorrhage promoted by TNF-alpha. PLoS One 2009;4:e6692.
84. Kasinskas RW, Forbes NS. Salmonella typhimurium lacking ribose chemoreceptors localize in tumor quiescence and induce apoptosis. Cancer Res 2007;67:3201-9.
85. Kwon SY, Thi-Thu Ngo H, Son J, Hong Y, Min JJ. Exploiting bacteria for cancer immunotherapy. Nat Rev Clin Oncol 2024;21:569-89.
86. Saito S, Cao DY, Maekawa T, Tsuji NM, Okuno A. Lactococcus lactis subsp. cremoris C60 upregulates macrophage function by modifying metabolic preference in enhanced anti-tumor immunity. Cancers 2024;16:1928.
87. Herrero-Fresno A, Olsen JE. Salmonella Typhimurium metabolism affects virulence in the host - a mini-review. Food Microbiol 2018;71:98-110.
88. Low KB, Ittensohn M, Le T, et al. Lipid A mutant Salmonella with suppressed virulence and TNFalpha induction retain tumor-targeting in vivo. Nat Biotechnol 1999;17:37-41.
89. Clairmont C, Lee KC, Pike J, et al. Biodistribution and genetic stability of the novel antitumor agent VNP20009, a genetically modified strain of Salmonella typhimurium. J Infect Dis 2000;181:1996-2002.
90. Song M, Kim HJ, Kim EY, et al. ppGpp-dependent stationary phase induction of genes on Salmonella pathogenicity island 1. J Biol Chem 2004;279:34183-90.
91. Na HS, Kim HJ, Lee HC, Hong Y, Rhee JH, Choy HE. Immune response induced by Salmonella typhimurium defective in ppGpp synthesis. Vaccine 2006;24:2027-34.
92. Sieow BF, Wun KS, Yong WP, Hwang IY, Chang MW. Tweak to treat: reprograming bacteria for cancer treatment. Trends Cancer 2021;7:447-64.
93. Park SH, Zheng JH, Nguyen VH, et al. RGD peptide cell-surface display enhances the targeting and therapeutic efficacy of attenuated salmonella-mediated cancer therapy. Theranostics 2016;6:1672-82.
94. Massa PE, Paniccia A, Monegal A, de Marco A, Rescigno M. Salmonella engineered to express CD20-targeting antibodies and a drug-converting enzyme can eradicate human lymphomas. Blood 2013;122:705-14.
95. Wu D, Zhao Z, Liu H, et al. Escherichia coli Nissle 1917-driven microrobots for effective tumor targeted drug delivery and tumor regression. Acta Biomater 2023;169:477-88.
96. Zhu J, Ke Y, Liu Q, et al. Engineered Lactococcus lactis secreting Flt3L and OX40 ligand for in situ vaccination-based cancer immunotherapy. Nat Commun 2022;13:7466.
97. Zhou DX, Wang XH, Xu X, et al. Anti-tumor effects of engineered VNP20009-Abvec-Igκ-mPD-1 strain in melanoma mice via combining the oncolytic therapy and immunotherapy. Pharmaceutics 2022;14:2789.
98. Ciaćma K, Więckiewicz J, Kędracka-Krok S, et al. Secretion of tumoricidal human tumor necrosis factor-related apoptosis-inducing ligand (TRAIL) by recombinant Lactococcus lactis: optimization of in vitro synthesis conditions. Microb Cell Fact 2018;17:177.
99. Yoon W, Yoo Y, Chae YS, Kee SH, Kim BM. Therapeutic advantage of genetically engineered Salmonella typhimurium carrying short hairpin RNA against inhibin alpha subunit in cancer treatment. Ann Oncol 2018;29:2010-7.
100. Zheng JH, Nguyen VH, Jiang SN, et al. Two-step enhanced cancer immunotherapy with engineered Salmonella typhimurium secreting heterologous flagellin. Sci Transl Med 2017;9:eaak9537.
101. Tan W, Duong MT, Zuo C, et al. Targeting of pancreatic cancer cells and stromal cells using engineered oncolytic Salmonella typhimurium. Mol Ther 2022;30:662-71.
102. Satoh Y, Kotani H, Iida Y, Taniura T, Notsu Y, Harada M. Supplementation of l-arginine boosts the therapeutic efficacy of anticancer chemoimmunotherapy. Cancer Sci 2020;111:2248-58.
103. Canale FP, Basso C, Antonini G, et al. Metabolic modulation of tumours with engineered bacteria for immunotherapy. Nature 2021;598:662-6.
104. Reich N, Hölscher C. The neuroprotective effects of glucagon-like peptide 1 in Alzheimer’s and Parkinson’s disease: an in-depth review. Front Neurosci 2022;16:970925.
105. Diz-Chaves Y, Mastoor Z, Spuch C, González-Matías LC, Mallo F. Anti-inflammatory effects of GLP-1 receptor activation in the brain in neurodegenerative diseases. Int J Mol Sci 2022;23:9583.
106. Nowell J, Blunt E, Edison P. Incretin and insulin signaling as novel therapeutic targets for Alzheimer’s and Parkinson’s disease. Mol Psychiatry 2023;28:217-29.
107. Batista AF, Bodart-Santos V, De Felice FG, Ferreira ST. Neuroprotective actions of glucagon-like peptide-1 (GLP-1) analogues in Alzheimer’s and Parkinson’s diseases. CNS Drugs 2019;33:209-23.
108. Wang RF, Xue GF, Hölscher C, et al. Post-treatment with the GLP-1 analogue liraglutide alleviate chronic inflammation and mitochondrial stress induced by Status epilepticus. Epilepsy Res 2018;142:45-52.
109. Loh JS, Mak WQ, Tan LKS, et al. Microbiota-gut-brain axis and its therapeutic applications in neurodegenerative diseases. Signal Transduct Target Ther 2024;9:37.
110. Chen T, Tian P, Huang Z, et al. Engineered commensal bacteria prevent systemic inflammation-induced memory impairment and amyloidogenesis via producing GLP-1. Appl Microbiol Biotechnol 2018;102:7565-75.
111. Yue M, Wei J, Chen W, Hong D, Chen T, Fang X. Neurotrophic role of the next-generation probiotic strain L. lactis MG1363-pMG36e-GLP-1 on Parkinson’s disease via inhibiting ferroptosis. Nutrients 2022;14:4886.
112. Fang X, Tian P, Zhao X, Jiang C, Chen T. Neuroprotective effects of an engineered commensal bacterium in the 1-methyl-4-phenyl-1, 2, 3, 6-tetrahydropyridine Parkinson disease mouse model via producing glucagon-like peptide-1. J Neurochem 2019;150:441-52.
113. Mukherjee S, Bassler BL. Bacterial quorum sensing in complex and dynamically changing environments. Nat Rev Microbiol 2019;17:371-82.
114. Gupta RK, Luong TT, Lee CY. RNAIII of the Staphylococcus aureus agr system activates global regulator MgrA by stabilizing mRNA. Proc Natl Acad Sci U S A 2015;112:14036-41.
115. Lubkowicz D, Ho CL, Hwang IY, Yew WS, Lee YS, Chang MW. Reprogramming probiotic Lactobacillus reuteri as a biosensor for staphylococcus aureus derived AIP-I detection. ACS Synth Biol 2018;7:1229-37.
116. Li H, Jia M, Qi Q, Wang Q. Engineered probiotic Lactobacillus plantarum WCSF I for monitoring and treatment of Staphylococcus aureus infection. Microbiol Spectr 2023;11:e0182923.
117. Mao N, Cubillos-Ruiz A, Cameron DE, Collins JJ. Probiotic strains detect and suppress cholera in mice. Sci Transl Med 2018;10:eaao2586.
118. Borrero J, Chen Y, Dunny GM, Kaznessis YN. Modified lactic acid bacteria detect and inhibit multiresistant enterococci. ACS Synth Biol 2015;4:299-306.
120. Mimee M, Nadeau P, Hayward A, et al. An ingestible bacterial-electronic system to monitor gastrointestinal health. Science 2018;360:915-8.
121. Zou ZP, Du Y, Fang TT, Zhou Y, Ye BC. Biomarker-responsive engineered probiotic diagnoses, records, and ameliorates inflammatory bowel disease in mice. Cell Host Microbe 2023;31:199-212.e5.
122. Xia JY, Hepler C, Tran P, Waldeck NJ, Bass J, Prindle A. Engineered calprotectin-sensing probiotics for IBD surveillance in humans. Proc Natl Acad Sci U S A 2023;120:e2221121120.
123. Meng T, Ma W, Fan M, Tang W, Duan X. Enhancing the contrast of tumor imaging for image-guided surgery using a tumor-targeting probiotic with the continuous expression of a biomarker. Anal Chem 2022;94:10109-17.
124. Danino T, Prindle A, Kwong GA, et al. Programmable probiotics for detection of cancer in urine. Sci Transl Med 2015;7:289ra84.
125. Tanniche I, Behkam B. Engineered live bacteria as disease detection and diagnosis tools. J Biol Eng 2023;17:65.
126. Perdigon G, Alvarez S, Nader DE Macias ME, Roux ME, de Ruiz Holgado AP. The oral administration of lactic acid bacteria increase the mucosal intestinal immunity in response to enteropathogens. J Food Prot 1990;53:404-10.
127. Hanniffy SB, Carter AT, Hitchin E, Wells JM. Mucosal delivery of a pneumococcal vaccine using Lactococcus lactis affords protection against respiratory infection. J Infect Dis 2007;195:185-93.
128. Lee JS, Poo H, Han DP, et al. Mucosal immunization with surface-displayed severe acute respiratory syndrome coronavirus spike protein on Lactobacillus casei induces neutralizing antibodies in mice. J Virol 2006;80:4079-87.
129. Xu YXY, Zhang XZ, Weng MM, et al. Oral immunization of mice with recombinant Lactobacillus plantarum expressing a Trichinella spiralis galectin induces an immune protection against larval challenge. Parasit Vectors 2022;15:475.
130. Zhang R, Peng X, Duan G, et al. An engineered Lactococcus lactis strain exerts significant immune responses through efficient expression and delivery of Helicobacter pylori Lpp20 antigen. Biotechnol Lett 2016;38:2169-75.
131. Zhang F, Ni L, Zhang Z, et al. Recombinant L. lactis vaccine LL-plSAM-WAE targeting four virulence factors provides mucosal immunity against H. pylori infection. Microb Cell Fact 2024;23:61.
132. Huynh DT, Chathuranga WAG, Chathuranga K, Lee JS, Kim CJ. Mucosal administration of Lactobacillus casei surface-displayed HA1 induces protective immune responses against avian influenza A virus in mice. J Microbiol Biotechnol 2024;34:735-45.
133. Sarnelli G, Del Re A, Palenca I, et al. Intranasal administration of Escherichia coli Nissle expressing the spike protein of SARS-CoV-2 induces long-term immunization and prevents spike protein-mediated lung injury in mice. Biomed Pharmacother 2024;174:116441.
134. Zhao Z, Wang H, Zhang D, et al. Oral vaccination with recombinant Lactobacillus casei expressing Aeromonas hydrophila Aha1 against A. hydrophila infections in common carps. Virulence 2022;13:794-807.
135. Zhang Y, Yang L, Zhang J, et al. Oral or intranasal immunization with recombinant Lactobacillus plantarum displaying head domain of Swine Influenza A virus hemagglutinin protects mice from H1N1 virus. Microb Cell Fact 2022;21:185.
136. Qiao N, Du G, Zhong X, Sun X. Recombinant lactic acid bacteria as promising vectors for mucosal vaccination. Exploration 2021;1:20210026.
137. Guo S, Yan W, McDonough SP, et al. The recombinant Lactococcus lactis oral vaccine induces protection against C. difficile spore challenge in a mouse model. Vaccine 2015;33:1586-95.
138. Sarate PJ, Heinl S, Poiret S, et al. E. coli Nissle 1917 is a safe mucosal delivery vector for a birch-grass pollen chimera to prevent allergic poly-sensitization. Mucosal Immunol 2019;12:132-44.
139. Rao S, Hu S, McHugh L, et al. Toward a live microbial microbicide for HIV: commensal bacteria secreting an HIV fusion inhibitor peptide. Proc Natl Acad Sci U S A 2005;102:11993-8.
140. Huang L, Tang W, He L, et al. Engineered probiotic Escherichia coli elicits immediate and long-term protection against influenza A virus in mice. Nat Commun 2024;15:6802.
141. Mohseni AH, Razavilar V, Keyvani H, Razavi MR, Khavari-Nejad RA. Oral immunization with recombinant Lactococcus lactis NZ9000 expressing human papillomavirus type 16 E7 antigen and evaluation of its immune effects in female C57BL/6 mice. J Med Virol 2019;91:296-307.
142. Li Y, Li X, Liu H, Zhuang S, Yang J, Zhang F. Intranasal immunization with recombinant Lactococci carrying human papillomavirus E7 protein and mouse interleukin-12 DNA induces E7-specific antitumor effects in C57BL/6 mice. Oncol Lett 2014;7:576-82.
143. Benz I, Schmidt MA. Cloning and expression of an adhesin (AIDA-I) involved in diffuse adherence of enteropathogenic Escherichia coli. Infect Immun 1989;57:1506-11.
144. Buddenborg C, Daudel D, Liebrecht S, Greune L, Humberg V, Schmidt MA. Development of a tripartite vector system for live oral immunization using a gram-negative probiotic carrier. Int J Med Microbiol 2008;298:105-14.
145. Sarnelli G, Del Re A, Pesce M, et al. Oral Immunization with Escherichia coli Nissle 1917 expressing SARS-CoV-2 spike protein induces mucosal and systemic antibody responses in mice. Biomolecules 2023;13:569.
146. Remer KA, Bartrow M, Roeger B, Moll H, Sonnenborn U, Oelschlaeger TA. Split immune response after oral vaccination of mice with recombinant Escherichia coli Nissle 1917 expressing fimbrial adhesin K88. Int J Med Microbiol 2009;299:467-78.
147. Wen LJ, Hou XL, Wang GH, et al. Immunization with recombinant Lactobacillus casei strains producing K99, K88 fimbrial protein protects mice against enterotoxigenic Escherichia coli. Vaccine 2012;30:3339-49.
148. Huang Y, Lin X, Yu S, Chen R, Chen W. Intestinal engineered probiotics as living therapeutics: chassis selection, colonization enhancement, gene circuit design, and biocontainment. ACS Synth Biol 2022;11:3134-53.
149. Li MC, He SH. IL-10 and its related cytokines for treatment of inflammatory bowel disease. World J Gastroenterol 2004;10:620-5.
150. Yang M, Gu Y, Li L, et al. Bile acid-gut microbiota axis in inflammatory bowel disease: from bench to bedside. Nutrients 2021;13:3143.
151. Wang Y, Shi Y, Peng X, et al. Biochemotaxis-oriented engineering bacteria expressing GLP-1 enhance diabetes therapy by regulating the balance of immune. Adv Healthc Mater 2024;13:e2303958.
152. Hausmann O, Daha M, Longo N, et al. Pegvaliase: Immunological profile and recommendations for the clinical management of hypersensitivity reactions in patients with phenylketonuria treated with this enzyme substitution therapy. Mol Genet Metab 2019;128:84-91.
153. Mater DD, Langella P, Corthier G, Flores MJ. A probiotic Lactobacillus strain can acquire vancomycin resistance during digestive transit in mice. J Mol Microbiol Biotechnol 2008;14:123-7.
154. Anisimova E, Gorokhova I, Karimullina G, Yarullina D. Alarming antibiotic resistance of Lactobacilli isolated from probiotic preparations and dietary supplements. Antibiotics 2022;11:1557.
155. Castro CP, Drumond MM, Batista VL, Nunes A, Mancha-Agresti P, Azevedo V. Vector development timeline for mucosal vaccination and treatment of disease using Lactococcus lactis and design approaches of next generation food grade plasmids. Front Microbiol 2018;9:1805.
156. Charbonneau MR, Isabella VM, Li N, Kurtz CB. Developing a new class of engineered live bacterial therapeutics to treat human diseases. Nat Commun 2020;11:1738.
157. Yan X, Liu X, Zhao C, Chen GQ. Applications of synthetic biology in medical and pharmaceutical fields. Signal Transduct Target Ther 2023;8:199.
158. Gibson DG, Glass JI, Lartigue C, et al. Creation of a bacterial cell controlled by a chemically synthesized genome. Science 2010;329:52-6.
159. Sung BH, Choe D, Kim SC, Cho BK. Construction of a minimal genome as a chassis for synthetic biology. Essays Biochem 2016;60:337-46.
160. Calero P, Nikel PI. Chasing bacterial chassis for metabolic engineering: a perspective review from classical to non-traditional microorganisms. Microb Biotechnol 2019;12:98-124.
161. Zhu D, Fu Y, Liu F, Xu H, Saris PE, Qiao M. Enhanced heterologous protein productivity by genome reduction in Lactococcus lactis NZ9000. Microb Cell Fact 2017;16:1.
162. Hille F, Richter H, Wong SP, Bratovič M, Ressel S, Charpentier E. The biology of CRISPR-Cas: backward and forward. Cell 2018;172:1239-59.
163. Hryhorowicz M, Lipiński D, Zeyland J, Słomski R. CRISPR/Cas9 immune system as a tool for genome engineering. Arch Immunol Ther Exp 2017;65:233-40.
164. Xiao Y, Ng S, Nam KH, Ke A. How type II CRISPR-Cas establish immunity through Cas1-Cas2-mediated spacer integration. Nature 2017;550:137-41.
165. Kleinstiver BP, Prew MS, Tsai SQ, et al. Engineered CRISPR-Cas9 nucleases with altered PAM specificities. Nature 2015;523:481-5.
166. Kim H, Kim JS. A guide to genome engineering with programmable nucleases. Nat Rev Genet 2014;15:321-34.
167. Gaj T, Gersbach CA, Barbas CF 3rd. ZFN, TALEN, and CRISPR/Cas-based methods for genome engineering. Trends Biotechnol 2013;31:397-405.
168. Ren J, Lee J, Na D. Recent advances in genetic engineering tools based on synthetic biology. J Microbiol 2020;58:1-10.
169. Gaudelli NM, Komor AC, Rees HA, et al. Programmable base editing of A•T to G•C in genomic DNA without DNA cleavage. Nature 2017;551:464-71.
170. Anzalone AV, Randolph PB, Davis JR, et al. Search-and-replace genome editing without double-strand breaks or donor DNA. Nature 2019;576:149-57.
172. Crawley AB, Henriksen ED, Stout E, Brandt K, Barrangou R. Characterizing the activity of abundant, diverse and active CRISPR-Cas systems in lactobacilli. Sci Rep 2018;8:11544.
173. Pan M, Morovic W, Hidalgo-Cantabrana C, et al. Genomic and epigenetic landscapes drive CRISPR-based genome editing in Bifidobacterium. Proc Natl Acad Sci U S A 2022;119:e2205068119.
174. Song X, Huang H, Xiong Z, Ai L, Yang S. CRISPR-Cas9D10A Nickase-assisted genome editing in Lactobacillus casei. Appl Environ Microbiol 2017;83:e01259-17.
175. Myrbråten IS, Wiull K, Salehian Z, et al. CRISPR interference for rapid knockdown of essential cell cycle genes in Lactobacillus plantarum. mSphere 2019;4:e00007-19.
176. Hidalgo-Cantabrana C, Goh YJ, Pan M, Sanozky-Dawes R, Barrangou R. Genome editing using the endogenous type I CRISPR-Cas system in Lactobacillus crispatus. Proc Natl Acad Sci U S A 2019;116:15774-83.
177. Han X, Chang L, Chen H, et al. Harnessing the endogenous Type I-C CRISPR-Cas system for genome editing in Bifidobacterium breve. Appl Environ Microbiol 2024;90:e0207423.
178. Ninyio N, Schmitt K, Sergon G, Nilsson C, Andersson S, Scherbak N. Stable expression of HIV-1 MPER extended epitope on the surface of the recombinant probiotic bacteria Escherichia Coli Nissle 1917 using CRISPR/Cas9. Microb Cell Fact 2024;23:39.
179. Zhou Y, Song F, Yang H, et al. Construction of a food-grade gene editing system based on CRISPR-Cas9 and its application in Lactococcus lactis NZ9000. Biotechnol Lett 2023;45:955-66.
180. Yu J, Zhou B, Zhang S, et al. Design of a self-driven probiotic-CRISPR/Cas9 nanosystem for sono-immunometabolic cancer therapy. Nat Commun 2022;13:7903.
181. Tian X, Liu X, Zhang Y, et al. Metabolic engineering coupled with adaptive evolution strategies for the efficient production of high-quality L-lactic acid by Lactobacillus paracasei. Bioresour Technol 2021;323:124549.
182. Oh JH, van Pijkeren JP. CRISPR-Cas9-assisted recombineering in Lactobacillus reuteri. Nucleic Acids Res 2014;42:e131.
183. Fang M, Zhang R, Wang C, et al. Engineering probiotic Escherichia coli Nissle 1917 to block transfer of multiple antibiotic resistance genes by exploiting a type I CRISPR-Cas system. Appl Environ Microbiol 2024;90:e0081124.
184. Lan Y, Tan S, Cheng S, et al. Development of Escherichia coli Nissle 1917 derivative by CRISPR/Cas9 and application for gamma-aminobutyric acid (GABA) production in antibiotic-free system. Biochem Eng J 2021;168:107952.
185. Luo W, Zhang Z, Zhou D, et al. Deep tumor penetration of CRISPR-Cas system for photothermal-sensitized immunotherapy via probiotics. Nano Lett 2023;23:8081-90.
186. Yang Y, Mao Y, Liu Y, et al. GEDpm-cg: genome editing automated design platform for point mutation construction in corynebacterium glutamicum. Front Bioeng Biotechnol 2021;9:768289.
187. Chien T, Harimoto T, Kepecs B, et al. Enhancing the tropism of bacteria via genetically programmed biosensors. Nat Biomed Eng 2022;6:94-104.
Cite This Article
How to Cite
Luo, Z.; Qi, Z.; Luo, J.; Chen, T. Potential applications of engineered bacteria in disease diagnosis and treatment. Microbiome. Res. Rep. 2025, 4, 10. http://dx.doi.org/10.20517/mrr.2024.57
Download Citation
Export Citation File:
Type of Import
Tips on Downloading Citation
Citation Manager File Format
Type of Import
Direct Import: When the Direct Import option is selected (the default state), a dialogue box will give you the option to Save or Open the downloaded citation data. Choosing Open will either launch your citation manager or give you a choice of applications with which to use the metadata. The Save option saves the file locally for later use.
Indirect Import: When the Indirect Import option is selected, the metadata is displayed and may be copied and pasted as needed.
About This Article
Special Issue
Copyright
Data & Comments
Data
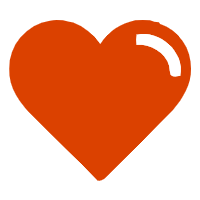
Comments
Comments must be written in English. Spam, offensive content, impersonation, and private information will not be permitted. If any comment is reported and identified as inappropriate content by OAE staff, the comment will be removed without notice. If you have any queries or need any help, please contact us at support@oaepublish.com.