Mobile genetic elements: the hidden puppet masters underlying infant gut microbiome assembly?
Abstract
The gut microbiota is important for healthy infant development. Part of the initial colonizing microbial strains originate from the maternal gut, and undergo a selective event, termed the “colonization bottleneck”. While vertical mother-to-infant inheritance and subsequent colonization of bacteria have previously been studied, the role of mobile genetic elements (MGEs) in the infant gut microbiota assembly is unclear. In this perspective article, we discuss how horizontally and vertically transmitted phages and conjugative elements potentially have important roles in infant gut microbiota assembly and colonization through parasitic and mutualistic interactions with their bacterial hosts. While some of these MGEs are likely to be detrimental to their host survival, in other contexts, they may help bacteria colonize new niches, antagonize other bacteria, or protect themselves from other parasitic MGEs in the infant gut. As a result, the horizontal transfer of MGEs likely occurs at high rates in the infant gut, contributing to gene transfer between bacteria and affecting which bacteria can pass the colonization bottleneck. We conclude by highlighting the potential in silico, in vitro, and in vivo methodological approaches that could be employed to study the transmission and colonization dynamics of MGEs and bacteria in the infant gut.
Keywords
INTRODUCTION
The human gut microbiota is a complex community of microorganisms, of which the most predominant and most studied are the bacteria. In the infant gut, this microbiota is vital for infant healthy development as it plays a part in immune system development, energy metabolism, and exclusion of pathogenic microbes[1-8]. Development of the microbiota starts at birth when the infants are first exposed to a wide diversity of bacteria from their mothers and the surrounding environment[9,10]. This initial community goes through a colonization bottleneck as the population starts at low diversity, with only a subset of bacterial taxa from the mother being vertically inherited to the infant gut[11-13] [Figure 1]. Although the specific ecological processes influencing this colonization event are not entirely understood, prior work suggests that bacterial competition and environmental factors such as oxygen concentrations affect bacterial colonization in infants[14-16]. While these advances are important in understanding the assembly of the infant gut microbiota, the influence of mobile genetic elements (MGEs) carried by colonizing bacteria, including plasmids and bacteriophages (phages for short)[17,18], on infant gut microbiome development has been rarely studied[19-21].
Figure 1. A schematic illustration of the colonization bottleneck and its effect on the diversity and abundance of bacteria and MGEs inherited from mother to infant. Only a subset of bacteria and associated MGEs might be able to pass the colonization bottleneck due to selection and stochastic chance events. The different bacterial taxa and MGEs are represented by different colors and symbols. MGEs: Mobile genetic elements.
In general, MGEs contribute to variable accessory genomes of bacteria and can drive horizontal gene transfer and recombination between different bacterial strains at the population level[22]. In the context of crossing the colonization bottleneck, MGEs could help their bacterial hosts adapt and survive by encoding fitness-enhancing genes. On the other hand, MGEs, especially phages, can be antagonistic to bacteria, preventing the colonization of bacteria by lysing them. Furthermore, MGEs often have the potential to move between different bacteria, which could create conflict between different MGEs within the same bacterial cells.
In this perspective, we will first define and examine the colonization bottleneck in the context of infant gut microbiome assembly, explaining how it might impact the vertical inheritance and subsequent colonization of three types of maternal MGEs: phages, conjugative elements, and phage plasmids (P-Ps). We then theorize how different MGEs affect their transmission dynamics and bacterial gene content and how they interact with their host bacteria and other MGE within the infant microbiome. Finally, we highlight the challenges of studying MGEs in gut microbiomes and suggest methodological tools and approaches to tackle these complex interactions in the future.
INFANT GUT MICROBIOME ASSEMBLY IS AFFECTED BY EARLY COLONIZATION BOTTLENECK
In ecology, a bottleneck is a loss in diversity caused by a large reduction in population size, as seen during the microbial colonization of the infant gut. The low population size makes the infant gut subject to chance events observed, such as when several bacterial strains are either vertically inherited at birth or acquired later on[11,12]. However, the population is also subject to unique selection pressures of the infant gut. For example, the relatively high oxygen concentration in the neonatal gut favors facultative anaerobes, such as Escherichia coli and Enterococcus faecalis[14,23]. Additionally, the complex sugars unique to breast milk (human milk oligosaccharides, HMOs) select for bacterial species that metabolize them, such as Bifidobacterium longum subsp. infantis[24].
While MGEs are intimately dependent on their hosts for survival, an MGE can employ two different lifestyles to colonize the infant gut: horizontal or vertical transfer between hosts [Figure 2]. In active horizontal transfer through conjugation or lysis, the MGE may hitchhike on any bacteria successfully passing the bottleneck. Alternatively, an MGE can rely on vertical transfer along with their bacterial hosts[25,26]. This can be driven by co-selection, where MGEs that reside in bacterial cells are vertically transferred to the daughter bacterial cells upon cell division along with colonizing bacteria. Very little is known about how common these two strategies are for MGE colonization. Nevertheless, they are likely to have significant consequences for the infant gut microbiome assembly as they provide different fitness benefits and create selection pressures on their host bacteria.
Figure 2. Two alternative routes of MGE inheritance enable them to cross the colonization bottleneck. In the vertical transmission scenario, MGEs are inherited together with their bacterial host during the cell division and this relationship could be beneficial for both (mutualism). In the horizontal transmission scenario, MGEs could move to any successfully colonizing bacterial host, breaking taxonomic associations between the bacterial hosts and their MGEs. This relationship could be considered parasitic as the fitness interests of MGEs are not aligned with those of their host. Most MGEs are capable of both modes of transmission and could hence be considered as facultative mutualists (or parasites)[25,26]. MGE: Mobile genetic element.
In the case of MGEs that use horizontal transfer to pass the colonization bottleneck, the fitness interests of the original host and MGE are not aligned, as an MGE may survive by hitchhiking onto a different host. It has been proposed that such relationships could be considered “selfish” or parasitic as the MGE would be selected for better transmission at the expense of its host[27-29]. Specifically, polyvalent phages that infect a range of bacterial taxa should be favored during the infant gut colonization, given the high and partly stochastic bacterial turnover increases the benefit of their ability to infect multiple hosts[11,13,30]. Consequently, horizontally transmitted MGEs could increase their own success in crossing the colonization bottleneck. However, this success can be constrained by the fitness costs they impose on their hosts, as seen with phages, which tend to spread after lysing their bacterial hosts[31].
If an MGE were to transfer vertically along with a bacterial host, we would expect to see co-selection where the host and MGE lineages persist together when transmitted from mothers to infants [Figure 2]. This could be enforced by “mutualistic” MGEs, which provide hosts with genes that improve their fitness[32-35]. However, MGEs often create genetic baggage, incurring metabolic costs that render their hosts less fit than the strains that do not carry MGEs[26,36]. Consequently, MGEs that primarily transfer vertically are expected to incur low fitness costs and/or compensate for them by providing fitness-enhancing genes to their hosts.
While vertical and horizontal transmission are considered important drivers in the parasitism-mutualist continuum, most MGEs likely fall between these two extremes with the ability to move horizontally or vertically depending on environmental conditions[25,26]. Moreover, obligate lytic phages could transmit along with their hosts when in pseudolysogeny, which is a poorly defined state where phages are temporarily dormant in the infected bacterium[31]. From this perspective, most MGEs can be seen as facultative mutualists, capable of both vertical and horizontal transmission between bacteria during colonization, which might be beneficial in highly dynamic and unpredictable environments such as the infant gut. We will next present current evidence of how the colonization bottleneck could select three groups of MGEs that are capable of vertical and horizontal transmission between bacterial cells: phages, conjugative elements, and P-Ps [Figure 3].
Figure 3. Three types of MGEs and how they are transmitted vertically or horizontally between bacterial cells that are inherited from the mother. The red color (left) represents conjugative elements (e.g., plasmids and ICEs), which can be maintained as extrachromosomal elements or integrated into the host genome and be transferred horizontally between bacterial cells via conjugation. The magenta color (center) represents P-Ps, which can be maintained as plasmids in host cells and transferred via viral lysis and potentially by conjugation. The dark blue color (right) represents phages that can be maintained integrated in the host genome or transferred between bacterial cells by viral lysis. MGEs: Mobile genetic elements; ICEs: integrative and conjugative elements; P-Ps: phage plasmids.
A PORTION OF PHAGES, CONJUGATIVE ELEMENTS AND P-PS ARE MATERNALLY INHERITED
Phages are bacterial viruses and the most abundant biological entity on earth[37]. Most phages are either obligately lytic or temperate. The lifestyle of obligately lytic phages involves infection of the host cell, production of new viral particles, lysis of the host cell, and release of new viral progeny [Figure 3]. In contrast, a temperate phage can additionally maintain its DNA integrated within its host’s genome as a prophage and transmit vertically to daughter bacterial cells[31]. Both phage types can move genetic material horizontally between bacteria, facilitating the transfer of potentially beneficial accessory genes.
Overall, phage and bacterial mother-to-infant inheritance follow similar patterns. Specifically, infant phage richness and diversity are lower compared to the maternal gut, and the infant gut phage turnover rate is extremely high[25,38,39], demonstrating how phages also pass the colonization bottleneck. In addition, approximately 15%-30% of the infant bacteriophage community (phageome) is composed of phages from the mother’s gut[40,41]. It is currently thought that most of these phages initially colonize the infant gut as prophages that enter the gut with their hosts[25]. There is increased prophage activity throughout infancy that peaks at 1-8 months after birth, with some studies predicting that over 75% of the free phages in the stool are temperate phages[41-45]. This induction spike may be an adaptation where phages attempt to establish themselves after the colonization bottleneck by transferring onto more fit hosts, especially given the high bacterial turnover during infancy. Supporting this, Vatanen et al. demonstrated that a maternal prophage was found in a different bacterial genome in their infant’s gut[19]. Importantly, such dynamics may increase the rate of horizontal gene transfer in the infant gut, as they transfer potential fitness-enhancing genes through processes such as lateral transduction[46].
Conjugative elements are well-studied MGEs composed of integrative and conjugative elements (ICEs, or conjugative transposons) and conjugative plasmids, which both move horizontally between bacterial species through conjugation [Figure 3]. This transfer mechanism does not depend on the lysis of their bacterial hosts but requires close contact between bacterial cells. One key difference between ICEs and plasmids is that the former are typically maintained as integrated into the host chromosome, while the latter are maintained as extrachromosomal elements in the cytoplasm.
Plasmid and ICE compositions tend to be more similar between familial mother-infant pairs and differ between vaginally and Cesarean section delivered infants[21,47,48]. For example, a highly prevalent plasmid called pBI143 is frequently identical between mothers and their infants, suggestive of mother-to-infant transmission[49]. While studies on conjugation activity in the infant gut are lacking, plasmid groups that are maintained for longer in the infant gut tend to encode conjugation-related genes[21]. In addition to this, some conjugative elements have been shown to increase conjugation rate in response to reactive oxygen species and/or the SOS response[50-53], signals that also trigger phage induction[54]. The conjugation rate can even be regulated by phage induction itself[33]. It is, therefore, possible that conjugative elements and phages may cross the colonization bottleneck by promoting their own conjugation and horizontal transfer.
P-Ps are hybrids between the MGEs they are named after, as they tend to encode both phage and plasmid genes[55-57]. While their diversity is only beginning to be uncovered, they mostly follow a lifestyle similar to temperate phages: they can enter lytic or temperate life cycle, but differ in that as a prophage, they form an extrachromosomal plasmid. While cultured P-Ps are only known to transfer horizontally through lysis, similar to phages, a subset of P-Ps encode genes necessary for conjugation and may therefore be able to transmit via conjugation [Figure 3].
Even though the abundance and diversity of P-Ps have only recently been studied[55,58], evidence suggests that infant gut P-Ps might also be maternally inherited. First, P1-like P-Ps, relatives of the well-studied P1 phage[59], were found in clinical and porcine-derived E. coli isolates, with the latter being isolated from the stool of a healthy 4-month-old piglet[60,61]. Additionally, some of the plasmids predicted to be N15-like P-Ps, relatives of the well-studied N15 phage[62], were isolated from bacterial hosts in dogs, giant pandas, and humans[56]. Finally, at least a fraction of phages of the family Crassvirales, the most abundant phage family in the human gut, appears to be P-P[63] and members of this phage family can be transmitted from mother to child[64]. While this evidence is intriguing, targeted studies confirming the presence and transmission of P-Ps between the mother and infant guts are required.
High bacterial turnover, stress, and phage induction levels suggest that MGEs can transmit horizontally between bacteria during infant gut colonization. While such dynamics may be necessary for MGEs in crossing the colonization bottleneck, they may also come at a fitness cost for their bacterial hosts, given that MGE spread, excess conjugation, and phage lysis can inhibit bacterial growth[31,65], emphasizing a parasitic interaction. However, such fitness costs are dependent on the ecological context. For example, while conjugative plasmids and phages can be costly to maintain, they can also provide fitness benefits to their bacterial hosts even in the absence of obvious selection pressures[66-68]. Most commonly, however, MGEs are thought to be maintained by carrying genes that can increase the fitness of their hosts[68]. In the next section, we explore the fitness-enhancing genes carried by MGEs and investigate how they could aid bacterial hosts in crossing the colonization bottleneck.
MGES CONTRIBUTE TO BACTERIAL COLONIZATION SUCCESS IN THE INFANT GUT
Some bacterial taxa only temporarily colonize the sparsely seeded infant gut and are subsequently outcompeted by other bacterial taxa[11,13,30]. Thus, the ability to aid in host metabolism, inhibit competitor growth, and protect oneself from competitors and parasites is important in influencing the successful crossing of the colonization bottleneck [Figure 4][69]. Fitness-enhancing genes providing such functions can be encoded by MGEs and could, therefore, be co-selected with their hosts.
Figure 4. Range of mechanisms of how the MGE-encoded beneficial functions might help their host bacteria to cross the colonization bottleneck. These functions include (A) aiding host metabolism and growth by encoding genes such as AMGs and CAZymes; (B) antagonizing competitor bacteria by encoding type VI secretion systems and bacteriocins; (C) resisting antibiosis competition by encoding ARGs, such as reuterin resistance genes[69]; and (D) defending the host from parasitic MGEs through Inc types, superinfection exclusion, and plasmid and phage defense systems. MGE: Mobile genetic element; AMGs: auxiliary metabolic genes; CAZymes: carbohydrate-active enzymes; ARGs: antibiotic resistance genes.
While direct evidence of MGEs aiding their hosts to increase their metabolic capacity through novel genes is lacking, metagenomic studies provide some tentative evidence. Specifically, prophages in the infant gut encode several auxiliary metabolic genes (AMGs)[70], including carbohydrate-active enzymes (CAZymes) related to carbon, amino acid, and energy metabolism[71-74] [Figure 4A]. These genes are likely involved in processes such as HMO and mucin attachment and degradation, as well as increasing growth rate by encoding more copies of rate-limiting genes[75]. Regarding conjugative elements, a recent study found that plasmids in the infant gut encode proportionally more unique metabolism-related genes compared to their bacterial hosts[21]. Additional bioinformatic and functional evidence suggests that conjugative elements can provide metabolic benefits to bacterial strains found in the gut microbiome[18,35,76-78]. In addition to this, most phages and plasmids encode a wide range of hypothetical proteins with no known functions, which could potentially provide important, novel functions to their hosts.
Besides aiding their hosts to increase their metabolic potential, MGEs could help their hosts colonize the infant gut by antagonizing or protecting against competitor bacteria. Specifically, phages and conjugative elements have been observed to carry bacteriocins and different types of growth-inhibiting genes which can provide a competitive edge against other bacterial strains[34,79-84] [Figure 4B]. Such genes have been suggested to be important for bacterial infant gut colonization[16,85] and may promote the transfer of MGEs, as in the case of Pseudomonas aeruginosa phages in an insect model[86]. Antibiotic resistance genes (ARGs) are another example of well-known genes that provide defense against competitor bacteria [Figure 4C]. In support of their importance, the infant gut harbors more ARGs compared to adults[48,87], and these are mostly carried by conjugative elements but not phages[88]. Additionally, P-Ps carry ARGs more often than phages, which indicates that they may contribute to the gut resistome[56]. More research is hence needed to explore if ARG carriage in MGEs provides a colonization benefit in the infant gut due to antibiotic usage or competition between other bacteria.
Finally, the fitness costs of the increased MGE horizontal transfer during the induction spike could constrain the prevalence of MGEs such as phages. Indeed, a few studies have proposed that the infant phageome follows the kill-the-winner (KtW) or kill-the-competitor (KtC) population dynamics as a result of selective phage predation targeting a subset of bacterial taxa[89,90]. Perhaps counterintuitively, various phage types, such as cryptic prophages, have been shown to encode an enormous diversity of functional phage defense genes that protect their bacterial hosts from both closely related and highly distinct phages[91-97]. Several of these defense systems can also target plasmids[93,96] [Figure 4D]. Although many defense systems seem to be exclusively encoded in phage genomes, Restriction-Modification (R-M), Toxin-Antitoxin (T-A), and clustered regularly interspaced short palindromic repeats (CRISPR) systems, for example, are also encoded in conjugative elements suggesting that to inhibit phage replication[33,98-102]. Plasmids can also exclude other related plasmids based on incompatibility (inc) types[47,103]. Although inc types are not traditionally thought of as a form of MGE defense, plasmid populations can be driven into extinction due to incompatibility[104,105]. It is, therefore, plausible that P-Ps have the greatest genetic potential in MGE defense since they can both exclude plasmids of the same inc type and also potentially protect bacteria against phages[106,107].
CURRENT CHALLENGES AND SOLUTIONS
While accumulating evidence suggests that MGEs can have a profound influence on the bacterial colonization of the infant gut, deciphering the roles and significance of different MGEs is challenging due to the inherent genomic characteristics of MGEs. First, the genome mosaicism and large amount of unknown open reading frames make MGEs and their functions difficult to quantify and characterize in metagenomic datasets. In particular, robust methodologies to identify and characterize novel P-Ps are urgently needed. Second, it is technically difficult to ascertain a host for an MGE in metagenomic datasets if the MGE is integrated into multiple sites or exists extrachromosomally, which is required for tracking its vertical and horizontal transmission between bacterial taxa.
While finding such signals in metagenomic datasets is challenging, promising sequencing and bioinformatic approaches are emerging. First, long-read sequencing technologies, such as PacBio and Oxford Nanopore Technologies (ONT), provide reads long enough to cover both an MGE and an associated bacterial genome. Additionally, ONT can provide an adaptive sequencing option that could be leveraged to selectively deplete non-MGE DNA or amplify MGEs that are at low abundance[108]. Using state-of-the-art bioinformatic tools combining machine learning and homology-based approaches, such as PlasX, VirSorter, VirFinder, and geNomad, to identify MGEs can also be used[18,109-111].
While these technologies enable the identification of several groups of MGEs, establishing the physical association of extrachromosomal MGEs with their hosts remains challenging. A technique called Hi-C, which involves fixing and cross-linking DNA molecules with proximity (both chromosomal and extrachromosomal DNA) followed by sequencing, could be used to resolve this. Moreover, microfluidics and single-cell sequencing have been used to successfully link extracellular elements, such as phages undergoing lytic infection and plasmids, to their hosts[112-114].
Unfortunately, the abovementioned techniques do not provide direct evidence for the identification of P-Ps. For example, Carjivirus communis phage was only recently confirmed to be a P-P through rigorous experimentation[63]. Thus, culturing remains a highly relevant technique for identifying and characterizing MGEs, as isolating gut bacteria, phages, and other MGEs enables direct experiments in lab cultures and the creation of synthetic model bacterial communities[115,116], which could be used to unravel the ecological and evolutionary roles of MGEs in model infant gut microbiomes. Finally, organoid and in vivo animal models with humanized gut microbiomes could provide opportunities to incorporate host cells and immune systems with controlled community experiments[117].
While this perspective focused only on a subset of MGEs, further work should also consider other smaller MGEs, such as “hitchers”, which are genetic elements that require other MGEs to transfer horizontally between bacterial hosts[118]. For example, phage satellites can lower the amount of phage particles that their helper phage produces upon lytic infection and can encode a wide array of phage defense genes[119-121]. To fully understand the impact of MGEs in the infant gut, we must consider the whole mobilome, including hitchers and other smaller MGEs that are incapable of transferring between bacterial cells.
CONCLUSIONS AND FUTURE PERSPECTIVES
In summary, there is currently a lack of evidence regarding the roles of MGE in infant gut bacterial assembly and colonization. Understanding what MGEs are maternally inherited and the underlying selection pressures and stochastic factors that affect MGE colonization are likely extremely important for understanding the infant gut microbiome assembly. We hypothesize that the stressors involved in crossing the colonization bottleneck are important drivers for MGE movement, which in turn is likely to affect the phylogenetic and functional composition of the bacteriome. Specifically, the temperate phage induction spike in infancy reflects the potential importance of horizontally transmitted MGEs in the infant gut. MGE-encoded accessory genes might lead to co-selection with associated bacteria, while defense systems targeting MGEs might limit their horizontal transmission and recombination. As more research is performed on viral dark matter and undiscovered MGEs, we will start better understanding the potential role of MGEs as puppet masters behind infant gut microbiome assembly.
Future research should focus on developing more robust methodologies for identifying and characterizing MGEs, especially novel P-Ps, in infant metagenomic datasets from diverse geographic regions. This is important to accurately assess the prevalence, abundance, and genetic content of MGEs in different populations. It would also be beneficial to collect more dense data, optimally with daily sampling, to understand early in vivo MGE dynamics in the infant gut in higher resolution. Further bioinformatic and experimental evidence into the functional roles of MGE-encoded genes in the infant gut, particularly those involved in metabolism, antagonism, and MGE defense, is required. Additionally, controlled experiments using multi-species synthetic bacterial communities and animal models could help elucidate the impact of environmental stressors on MGE transmission dynamics and the relative fitness benefits and costs they may impart to their hosts. Finally, expanding research to encompass a wider array of MGEs, including smaller elements like “hitchers”, will provide a more comprehensive understanding of the infant gut mobilome and its influence on microbial community assembly.
Glossary:
Vertical inheritance: The inheritance of microbes or MGEs from mother to child upon birth.
Vertical MGE transmission: The transmission of MGEs to the daughter cells of the same bacteria by cell division.
Horizontal MGE transmission: The transmission of mobile MGEs between different bacterial cells independent of cell division.
MGE/phage defense genes: Genes dedicated to interfering with the replication or maintenance of MGEs or phages that have infected the cell.
Colonization bottleneck: A process that limits what microbes can colonize the infant gut microbiome, resulting in a decrease in genetic and taxonomic diversity.
Kill-the-winner (KtW) dynamics: A predator-prey type dynamic where phages control populations of the most successful bacteria through lysis.
Kill-the-competitor (KtC) dynamics: A predator-prey type dynamic where phages protect their original host bacteria by lysing competitive bacteria.
Core genome: The part of the bacterial genome that is conserved in all strains within the same taxa.
Accessory genome: The variable part of the bacterial genome that is not found in all strains within the same taxon.
DECLARATIONS
Authors’ contributions
Conception, content, and interpretation of this article: Kreuze K, Friman VP, Vatanen T
Availability of data and materials
Not applicable.
Financial support and sponsorship
Kreuze K and Vatanen T were supported by the Research Council of Finland grants 346950 and 353513.
Conflicts of interest
All authors declared that there are no conflicts of interest.
Ethical approval and consent to participate
Not applicable.
Consent for publication
Not applicable.
Copyright
© The Author(s) 2024.
REFERENCES
1. Charbonneau MR, O’Donnell D, Blanton LV, et al. Sialylated milk oligosaccharides promote microbiota-dependent growth in models of infant undernutrition. Cell 2016;164:859-71.
2. Riva A, Borgo F, Lassandro C, et al. Pediatric obesity is associated with an altered gut microbiota and discordant shifts in Firmicutes populations. Environ Microbiol 2017;19:95-105.
3. Kim YG, Sakamoto K, Seo SU, et al. Neonatal acquisition of Clostridia species protects against colonization by bacterial pathogens. Science 2017;356:315-9.
4. Henrick BM, Rodriguez L, Lakshmikanth T, et al. Bifidobacteria-mediated immune system imprinting early in life. Cell 2021;184:3884-98.e11.
5. Yao Y, Cai X, Ye Y, Wang F, Chen F, Zheng C. The role of microbiota in infant health: from early life to adulthood. Front Immunol 2021;12:708472.
6. Yassour M, Vatanen T, Siljander H, et al; DIABIMMUNE Study Group. Natural history of the infant gut microbiome and impact of antibiotic treatment on bacterial strain diversity and stability. Sci Transl Med 2016;8:343ra81.
7. Mitchell CM, Mazzoni C, Hogstrom L, et al. Delivery mode affects stability of early infant gut microbiota. Cell Rep Med 2020;1:100156.
8. Yang R, Wang Y, Ying Z, et al. Inspecting mother-to-infant microbiota transmission: disturbance of strain inheritance by cesarian section. Front Microbiol 2024;15:1292377.
9. Lou YC, Olm MR, Diamond S, et al. Infant gut strain persistence is associated with maternal origin, phylogeny, and traits including surface adhesion and iron acquisition. Cell Rep Med 2021;2:100393.
10. Bogaert D, van Beveren GJ, de Koff EM, et al. Mother-to-infant microbiota transmission and infant microbiota development across multiple body sites. Cell Host Microbe 2023;31:447-60.e6.
11. Ferretti P, Pasolli E, Tett A, et al. Mother-to-infant microbial transmission from different body sites shapes the developing infant gut microbiome. Cell Host Microbe 2018;24:133-45.e5.
12. Yassour M, Jason E, Hogstrom LJ, et al. Strain-level analysis of mother-to-child bacterial transmission during the first few months of life. Cell Host Microbe 2018;24:146-54.e4.
13. Chen DW, Garud NR. Rapid evolution and strain turnover in the infant gut microbiome. Genome Res 2022;32:1124-36.
14. Scholtens PA, Oozeer R, Martin R, Amor KB, Knol J. The early settlers: intestinal microbiology in early life. Annu Rev Food Sci Technol 2012;3:425-47.
15. Mäklin T, Thorpe HA, Pöntinen AK, et al. Strong pathogen competition in neonatal gut colonisation. Nat Commun 2022;13:7417.
16. Ormaasen I, Rudi K, Diep DB, Snipen L. Metagenome-mining indicates an association between bacteriocin presence and strain diversity in the infant gut. BMC Genomics 2023;24:295.
17. Gregory AC, Zablocki O, Zayed AA, Howell A, Bolduc B, Sullivan MB. The gut virome database reveals age-dependent patterns of virome diversity in the human gut. Cell Host Microbe 2020;28:724-40.e8.
18. Yu MK, Fogarty EC, Eren AM. Diverse plasmid systems and their ecology across human gut metagenomes revealed by PlasX and MobMess. Nat Microbiol 2024;9:830-47.
19. Vatanen T, Jabbar KS, Ruohtula T, et al. Mobile genetic elements from the maternal microbiome shape infant gut microbial assembly and metabolism. Cell 2022;185:4921-36.e15.
20. Shah SA, Deng L, Thorsen J, et al. Expanding known viral diversity in the healthy infant gut. Nat Microbiol 2023;8:986-98.
21. He W, Russel J, Klincke F, Nesme J, Sørensen SJ. Insights into the ecology of the infant gut plasmidome. Nat Commun 2024;15:6924.
22. Hall JPJ, Harrison E, Baltrus DA. Introduction: the secret lives of microbial mobile genetic elements. Philos Trans R Soc Lond B Biol Sci 2022;377:20200460.
23. Bittinger K, Zhao C, Li Y, et al. Bacterial colonization reprograms the neonatal gut metabolome. Nat Microbiol 2020;5:838-47.
24. Vatanen T, Ang QY, Siegwald L, et al. A distinct clade of Bifidobacterium longum in the gut of Bangladeshi children thrives during weaning. Cell 2022;185:4280-97.e12.
25. Liang G, Zhao C, Zhang H, et al. The stepwise assembly of the neonatal virome is modulated by breastfeeding. Nature 2020;581:470-4.
26. Bethke JH, Ma HR, Tsoi R, Cheng L, Xiao M, You L. Vertical and horizontal gene transfer tradeoffs direct plasmid fitness. Mol Syst Biol 2023;19:e11300.
27. Ewald PW. Transmission modes and evolution of the parasitism-mutualism continuum. Ann N Y Acad Sci 1987;503:295-306.
28. Turner PE, Cooper VS, Lenski RE. Tradeoff between horizontal and vertical modes of transmission in bacterial plasmids. Evolution 1998;52:315-29.
29. Rankin DJ, Rocha EP, Brown SP. What traits are carried on mobile genetic elements, and why? Heredity 2011;106:1-10.
30. Niu J, Xu L, Qian Y, et al. Evolution of the gut microbiome in early childhood: a cross-sectional study of Chinese children. Front Microbiol 2020;11:439.
31. Mäntynen S, Laanto E, Oksanen HM, Poranen MM, Díaz-Muñoz SL. Black box of phage-bacterium interactions: exploring alternative phage infection strategies. Open Biol 2021;11:210188.
32. Davray D, Deo D, Kulkarni R. Plasmids encode niche-specific traits in Lactobacillaceae. Microb Genom 2021;7:mgen000472.
33. LeGault KN, Hays SG, Angermeyer A, et al. Temporal shifts in antibiotic resistance elements govern phage-pathogen conflicts. Science 2021;373:eabg2166.
34. Dragoš A, Andersen AJC, Lozano-Andrade CN, Kempen PJ, Kovács ÁT, Strube ML. Phages carry interbacterial weapons encoded by biosynthetic gene clusters. Curr Biol 2021;31:3479-89.e5.
35. Dineen RL, Bottacini F, O’Connell-Motherway M, van Sinderen D. Transcriptional landscape of the pMP7017 megaplasmid and its impact on the Bifidobacterium breve UCC2003 transcriptome. Microb Biotechnol 2024;17:e14405.
36. Martínez Arbas S, Narayanasamy S, Herold M, et al. Roles of bacteriophages, plasmids and CRISPR immunity in microbial community dynamics revealed using time-series integrated meta-omics. Nat Microbiol 2021;6:123-35.
38. Tisza M, Lloyd R, Hoffman K, et al; TEDDY Study Group. Phage-bacteria dynamics during the first years of life revealed by trans-kingdom marker gene analysis. bioRxiv 2023.
39. Lou YC, Chen L, Borges AL, et al. Infant gut DNA bacteriophage strain persistence during the first 3 years of life. Cell Host Microbe 2024;32:35-47.e6.
40. Maqsood R, Rodgers R, Rodriguez C, et al. Discordant transmission of bacteria and viruses from mothers to babies at birth. Microbiome 2019;7:156.
41. Garmaeva S, Sinha T, Gulyaeva A, et al; Lifelines NEXT cohort study. Transmission and dynamics of mother-infant gut viruses during pregnancy and early life. Nat Commun 2024;15:1945.
42. Mathieu A, Dion M, Deng L, et al. Virulent coliphages in 1-year-old children fecal samples are fewer, but more infectious than temperate coliphages. Nat Commun 2020;11:378.
43. Redgwell TA, Thorsen J, Petit MA, et al. Prophages in the infant gut are largely induced, and may be functionally relevant to their hosts. Available from: https://www.biorxiv.org/content/10.1101/2021.06.25.449885v1. [Last accessed on 8 Nov 2024].
44. Beller L, Deboutte W, Vieira-Silva S, et al. The virota and its transkingdom interactions in the healthy infant gut. Proc Natl Acad Sci U S A 2022;119:e2114619119.
45. Zeng S, Almeida A, Li S, et al. A metagenomic catalog of the early-life human gut virome. Nat Commun 2024;15:1864.
46. Humphrey S, Fillol-Salom A, Quiles-Puchalt N, et al. Bacterial chromosomal mobility via lateral transduction exceeds that of classical mobile genetic elements. Nat Commun 2021;12:6509.
47. Ravi A, Valdés-Varela L, Gueimonde M, Rudi K. Transmission and persistence of IncF conjugative plasmids in the gut microbiota of full-term infants. FEMS Microbiol Ecol 2018;94:fix158.
48. Pärnänen K, Karkman A, Hultman J, et al. Maternal gut and breast milk microbiota affect infant gut antibiotic resistome and mobile genetic elements. Nat Commun 2018;9:3891.
49. Fogarty EC, Schechter MS, Lolans K, et al. A cryptic plasmid is among the most numerous genetic elements in the human gut. Cell 2024;187:1206-22.e16.
50. Beaber JW, Hochhut B, Waldor MK. SOS response promotes horizontal dissemination of antibiotic resistance genes. Nature 2004;427:72-4.
51. Chen X, Yin H, Li G, et al. Antibiotic-resistance gene transfer in antibiotic-resistance bacteria under different light irradiation: implications from oxidative stress and gene expression. Water Res 2019;149:282-91.
52. Yu Z, Wang Y, Lu J, Bond PL, Guo J. Nonnutritive sweeteners can promote the dissemination of antibiotic resistance through conjugative gene transfer. ISME J 2021;15:2117-30.
53. Yu Z, Henderson IR, Guo J. Non-caloric artificial sweeteners modulate conjugative transfer of multi-drug resistance plasmid in the gut microbiota. Gut Microbes 2023;15:2157698.
54. Hu J, Ye H, Wang S, Wang J, Han D. Prophage activation in the intestine: insights into functions and possible applications. Front Microbiol 2021;12:785634.
55. Pfeifer E, Moura de Sousa JA, Touchon M, Rocha EPC. Bacteria have numerous distinctive groups of phage-plasmids with conserved phage and variable plasmid gene repertoires. Nucleic Acids Res 2021;49:2655-73.
56. Pfeifer E, Bonnin RA, Rocha EPC. Phage-plasmids spread antibiotic resistance genes through infection and lysogenic conversion. mBio 2022;13:e0185122.
57. Pfeifer E, Rocha EPC. Phage-plasmids promote recombination and emergence of phages and plasmids. Nat Commun 2024;15:1545.
58. Piligrimova EG, Kazantseva OA, Kazantsev AN, et al. Putative plasmid prophages of Bacillus cereus sensu lato may hold the key to undiscovered phage diversity. Sci Rep 2021;11:7611.
59. Łobocka MB, Rose DJ, Plunkett G 3rd, et al. Genome of bacteriophage P1. J Bacteriol 2004;186:7032-68.
60. Carattoli A. Resistance plasmid families in Enterobacteriaceae. Antimicrob Agents Chemother 2009;53:2227-38.
61. Venturini C, Zingali T, Wyrsch ER, et al. Diversity of P1 phage-like elements in multidrug resistant Escherichia coli. Sci Rep 2019;9:18861.
63. Schmidtke DT, Hickey AS, Liachko I, Sherlock G, Bhatt AS. Analysis and culturing of the prototypic crAssphage reveals a phage-plasmid lifestyle. Available from: https://www.biorxiv.org/content/10.1101/2024.03.20.585998v2. [Last accessed on 8 Nov 2024].
64. Siranosian BA, Tamburini FB, Sherlock G, Bhatt AS. Acquisition, transmission and strain diversity of human gut-colonizing crAss-like phages. Nat Commun 2020;11:280.
65. Porse A, Schønning K, Munck C, Sommer MO. Survival and evolution of a large multidrug resistance plasmid in new clinical bacterial hosts. Mol Biol Evol 2016;33:2860-73.
66. Shapiro JW, Williams ES, Turner PE. Evolution of parasitism and mutualism between filamentous phage M13 and Escherichia coli. PeerJ 2016;4:e2060.
67. Shapiro JW, Turner PE. The impact of transmission mode on the evolution of benefits provided by microbial symbionts. Ecol Evol 2014;4:3350-61.
68. Carroll AC, Wong A. Plasmid persistence: costs, benefits, and the plasmid paradox. Can J Microbiol 2018;64:293-304.
69. Moraïs S, Mazor M, Tovar-Herrera O, et al. Plasmid-encoded toxin defence mediates mutualistic microbial interactions. Nat Microbiol 2024;9:108-19.
70. Breitbart M, Thompson L, Suttle C, Sullivan M. Exploring the vast diversity of marine viruses. Oceanog 2007;20:135-9.
71. Dikareva E, Matharu D, Lahtinen E, et al. An extended catalog of integrated prophages in the infant and adult fecal microbiome shows high prevalence of lysogeny. Front Microbiol 2023;14:1254535.
72. Reyes A, Haynes M, Hanson N, et al. Viruses in the faecal microbiota of monozygotic twins and their mothers. Nature 2010;466:334-8.
73. Monaghan TM, Sloan TJ, Stockdale SR, et al. Metagenomics reveals impact of geography and acute diarrheal disease on the Central Indian human gut microbiome. Gut Microbes 2020;12:1752605.
74. Shaffer M, Borton MA, McGivern BB, et al. DRAM for distilling microbial metabolism to automate the curation of microbiome function. Nucleic Acids Res 2020;48:8883-900.
75. Hurwitz BL, U’Ren JM. Viral metabolic reprogramming in marine ecosystems. Curr Opin Microbiol 2016;31:161-8.
76. Desmond C, Ross RP, Fitzgerald G, Stanton C. Sequence analysis of the plasmid genome of the probiotic strain Lactobacillus paracasei NFBC338 which includes the plasmids pCD01 and pCD02. Plasmid 2005;54:160-75.
77. Coyne MJ, Zitomersky NL, McGuire AM, Earl AM, Comstock LE. Evidence of extensive DNA transfer between bacteroidales species within the human gut. mBio 2014;5:e01305-14.
79. Kommineni S, Bretl DJ, Lam V, et al. Bacteriocin production augments niche competition by enterococci in the mammalian gastrointestinal tract. Nature 2015;526:719-22.
80. Chassaing B, Cascales E. Antibacterial weapons: targeted destruction in the microbiota. Trends Microbiol 2018;26:329-38.
81. Hols P, Ledesma-García L, Gabant P, Mignolet J. Mobilization of microbiota commensals and their bacteriocins for therapeutics. Trends Microbiol 2019;27:690-702.
82. Peñil-Celis A, Garcillán-Barcia MP. Crosstalk between type VI secretion system and mobile genetic elements. Front Mol Biosci 2019;6:126.
83. García-Bayona L, Coyne MJ, Comstock LE. Mobile type VI secretion system loci of the gut Bacteroidales display extensive intra-ecosystem transfer, multi-species spread and geographical clustering. PLoS Genet 2021;17:e1009541.
84. Takala TM, Mokhtari S, Ahonen SL, Wan X, Saris PEJ. Wild-type Lactococcus lactis producing bacteriocin-like prophage lysins. Front Microbiol 2023;14:1219723.
85. Verster AJ, Ross BD, Radey MC, et al. The landscape of type VI secretion across human gut microbiomes reveals its role in community composition. Cell Host Microbe 2017;22:411-9.e4.
86. Burns N, James CE, Harrison E. Polylysogeny magnifies competitiveness of a bacterial pathogen in vivo. Evol Appl 2015;8:346-51.
87. Li X, Brejnrod A, Thorsen J, et al. Differential responses of the gut microbiome and resistome to antibiotic exposures in infants and adults. Nat Commun 2023;14:8526.
88. Takeuchi N, Hamada-Zhu S, Suzuki H. Prophages and plasmids can display opposite trends in the types of accessory genes they carry. Proc Biol Sci 2023;290:20231088.
89. Mirzaei MK, Maurice CF. Ménage à trois in the human gut: interactions between host, bacteria and phages. Nat Rev Microbiol 2017;15:397-408.
90. Brown TL, Charity OJ, Adriaenssens EM. Ecological and functional roles of bacteriophages in contrasting environments: marine, terrestrial and human gut. Curr Opin Microbiol 2022;70:102229.
91. Melderen L, Saavedra De Bast M. Bacterial toxin-antitoxin systems: more than selfish entities? PLoS Genet 2009;5:e1000437.
92. Bondy-Denomy J, Qian J, Westra ER, et al. Prophages mediate defense against phage infection through diverse mechanisms. ISME J 2016;10:2854-66.
93. Doron S, Melamed S, Ofir G, et al. Systematic discovery of antiphage defense systems in the microbial pangenome. Science 2018;359:eaar4120.
94. Hussain FA, Dubert J, Elsherbini J, et al. Rapid evolutionary turnover of mobile genetic elements drives bacterial resistance to phages. Science 2021;374:488-92.
95. LeRoux M, Srikant S, Teodoro GIC, et al. The DarTG toxin-antitoxin system provides phage defence by ADP-ribosylating viral DNA. Nat Microbiol 2022;7:1028-40.
96. Hochhauser D, Millman A, Sorek R. The defense island repertoire of the Escherichia coli pan-genome. PLoS Genet 2023;19:e1010694.
97. Shaw LP, Rocha EPC, MacLean RC. Restriction-modification systems have shaped the evolution and distribution of plasmids across bacteria. Nucleic Acids Res 2023;51:6806-18.
98. Oliveira PH, Touchon M, Rocha EP. The interplay of restriction-modification systems with mobile genetic elements and their prokaryotic hosts. Nucleic Acids Res 2014;42:10618-31.
99. Picton DM, Luyten YA, Morgan RD, et al. The phage defence island of a multidrug resistant plasmid uses both BREX and type IV restriction for complementary protection from viruses. Nucleic Acids Res 2021;49:11257-73.
100. Bleriot I, Blasco L, Pacios O, et al. The role of PemIK (PemK/PemI) type II TA system from Klebsiella pneumoniae clinical strains in lytic phage infection. Sci Rep 2022;12:4488.
101. Pinilla-Redondo R, Russel J, Mayo-Muñoz D, et al. CRISPR-Cas systems are widespread accessory elements across bacterial and archaeal plasmids. Nucleic Acids Res 2022;50:4315-28.
102. Botelho J. Defense systems are pervasive across chromosomally integrated mobile genetic elements and are inversely correlated to virulence and antimicrobial resistance. Nucleic Acids Res 2023;51:4385-97.
104. Hale L, Lazos O, Haines A, Thomas C. An efficient stress-free strategy to displace stable bacterial plasmids. Biotechniques 2010;48:223-8.
105. Kamruzzaman M, Shoma S, Thomas CM, Partridge SR, Iredell JR. Plasmid interference for curing antibiotic resistance plasmids in vivo. PLoS One 2017;12:e0172913.
106. Hedges RW, Jacob AE, Barth PT, Grinter NJ. Compatibility properties of P1 and PhiAMP prophages. Mol Gen Genet 1975;141:263-7.
107. Kliem M, Dreiseikelmann B. The superimmunity gene sim of bacteriophage P1 causes superinfection exclusion. Virology 1989;171:350-5.
108. Martin S, Heavens D, Lan Y, Horsfield S, Clark MD, Leggett RM. Nanopore adaptive sampling: a tool for enrichment of low abundance species in metagenomic samples. Genome Biol 2022;23:11.
109. Camargo AP, Roux S, Schulz F, et al. Identification of mobile genetic elements with geNomad. Nat Biotechnol 2024;42:1303-12.
110. Guo J, Bolduc B, Zayed AA, et al. VirSorter2: a multi-classifier, expert-guided approach to detect diverse DNA and RNA viruses. Microbiome 2021;9:37.
111. Ren J, Ahlgren NA, Lu YY, Fuhrman JA, Sun F. VirFinder: a novel k-mer based tool for identifying viral sequences from assembled metagenomic data. Microbiome 2017;5:69.
112. Kent AG, Vill AC, Shi Q, Satlin MJ, Brito IL. Widespread transfer of mobile antibiotic resistance genes within individual gut microbiomes revealed through bacterial Hi-C. Nat Commun 2020;11:4379.
113. Marbouty M, Thierry A, Millot GA, Koszul R. MetaHiC phage-bacteria infection network reveals active cycling phages of the healthy human gut. Elife 2021;10:e60608.
114. Lawrence D, Campbell DE, Schriefer LA, et al. Single-cell genomics for resolution of conserved bacterial genes and mobile genetic elements of the human intestinal microbiota using flow cytometry. Gut Microbes 2022;14:2029673.
115. Browne HP, Forster SC, Anonye BO, et al. Culturing of ‘unculturable’ human microbiota reveals novel taxa and extensive sporulation. Nature 2016;533:543-6.
116. Fitzgerald CB, Shkoporov AN, Upadrasta A, Khokhlova EV, Ross RP, Hill C. Probing the “dark matter” of the human gut phageome: culture assisted metagenomics enables rapid discovery and host-linking for novel bacteriophages. Front Cell Infect Microbiol 2021;11:616918.
117. Jin Z, Ng A, Maurice CF, Juncker D. The mini colon model: a benchtop multi-bioreactor system to investigate the gut microbiome. Gut Microbes 2022;14:2096993.
118. Ares-Arroyo M, Coluzzi C, de Sousa JAM, Rocha EPC. Hijackers, hitchhikers, or co-drivers? The mysteries of microbial mobilizable genetic elements. EcoEvoRxiv 2024.
119. Ibarra-Chávez R, Brady A, Chen J, Penadés JR, Haag AF. Phage-inducible chromosomal islands promote genetic variability by blocking phage reproduction and protecting transductants from phage lysis. PLoS Genet 2022;18:e1010146.
120. Rousset F, Depardieu F, Miele S, et al. Phages and their satellites encode hotspots of antiviral systems. Cell Host Microbe 2022;30:740-53.e5.
Cite This Article
How to Cite
Download Citation
Export Citation File:
Type of Import
Tips on Downloading Citation
Citation Manager File Format
Type of Import
Direct Import: When the Direct Import option is selected (the default state), a dialogue box will give you the option to Save or Open the downloaded citation data. Choosing Open will either launch your citation manager or give you a choice of applications with which to use the metadata. The Save option saves the file locally for later use.
Indirect Import: When the Indirect Import option is selected, the metadata is displayed and may be copied and pasted as needed.
About This Article
Special Issue
Copyright
Data & Comments
Data
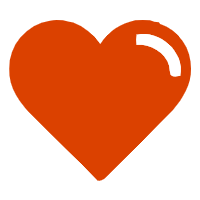
Comments
Comments must be written in English. Spam, offensive content, impersonation, and private information will not be permitted. If any comment is reported and identified as inappropriate content by OAE staff, the comment will be removed without notice. If you have any queries or need any help, please contact us at [email protected].