Wavelength-tunable deep blue emission from pure bromide-based colloidal perovskite nanocrystals
Abstract
Metal halide perovskites are promising light emitters due to their tunable and highly pure emission color in visible light. However, achieving deep blue emission remains a major challenge due to low stability and intrinsic defects. Traditional methods for synthesizing blue-emitting colloidal perovskite nanocrystals (PNCs) involve organic ammonium engineering and halide engineering, which often suffer from problems such as ion migration and color instability. In this study, we demonstrate a novel central metal engineering approach that achieves deep blue emission with a wavelength of 435.8 nm from pure bromide-based PNCs at room temperature. To synthesize deep blue-emitting pure-bromide-based PNCs, we incorporate manganese bromide (MnBr2) to the formamidinium-guanidinium lead bromide (FA0.9GA0.1PbBr3) PNCs. Mn2+ suppresses the growth of FA0.9GA0.1PbBr3 crystals during the synthesis, resulting in decreases in both particle size and dimensionality and deep blue emission by the quantum confinement effect. The emission wavelength of pure-bromide-based PNCs is controlled by varying the amount of MnBr2. This study provides an effective and simple method for achieving deep blue emission from pure bromide-based PNCs, offering significant advantages for display technologies such as light-emitting diodes.
Keywords
INTRODUCTION
Metal halide perovskites (MHPs) consist of three ions in an ABX3 structure, where A is an organic ammonium {e.g., methylammonium (MA; CH3NH3+), formamidinium [FA; CH(NH2)2+]} or an alkali-metal cation (e.g., Cs+), B is a transition-metal cation (e.g., Pb2+, Au2+, Sn2+, Mn2+), and X is a halide anion
Conventionally, X-site anion engineering has been attempted to synthesize blue-emitting PNCs[19]. While the conduction band is formed by antibonding mixing of the 6p6 orbitals of lead metal and the np6 orbitals of halide (n = 3, 4, and 5 for Cl, Br, and I, respectively), the valence band is formed primarily by mixing of the np6 orbitals of halide and the 6s2 orbitals of lead metal[20]. The energy of the np6 orbitals of halide decreases as the halide moves from I (5p6) to Br (4p6) to Cl (3p6), shifting the valence band maximum to higher positive potentials. Thus, a systematic change in the halide composition of CsPbX3 PNCs from CsPbI3 and CsPbBr3 to CsPbCl3 results in an increase in the bandgap and blue-shift in the emitting wavelength. For example, Protesescu et al. demonstrated blue light with tunable wavelengths from 410 nm to 460 nm using Cl-based mixed halides in inorganic lead halide perovskites (CsPbX3)[19]. However, in mixed-halide PNCs developed through X-site engineering, an applied electric field to the PNC films in PeLEDs can induce ion migration and the change of luminescence color because halide ions have low activation energy for ion migration[3]. Furthermore, addition of chloride induces deep-level trap states in the bandgap, which reduces the device efficiency[21]. Therefore, it is important to achieve blue emission from pure halide PNCs.
A-site cation engineering has been tried to achieve blue emission from pure bromide PNCs[21]. Large-sized A-site cations induce smaller-sized PNCs by suppressing their growth[22] or lead to low-dimensional PNCs by restricting their expansion in the orthogonal direction[23-25]. In these PNCs, an increased bandgap and blue-shifted photoluminescence (PL) are observed due to quantum-confinement effect[25] or dielectric confinement effect[26] although A-site cations do not directly participate in the band edge[21]. Despite the successful blue emission from pure-halide PNCs through A-site engineering, A-site engineered
Recently, B-site engineering has been attempted to achieve blue emission from pure halide PNCs. Wang et al. reported that incorporating Zn2+ into the precursor solution retards the growth of perovskite crystals, leading to FA lead bromide (FAPbBr3) PNCs with smaller size and thus achieving blue emission[27]. The wavelength of emitted light can be tuned by controlling the amount of Zn2+; however, the wavelength is limited to above 486 nm, resulting in a sky-blue color. To demonstrate high color purity displays and cover the wide range in International Commission on Illumination (CIE) chromaticity coordinate, new B-site engineering methods that enable deep blue emission (Royal blue color) with a wavelength below 450 nm from pure-halide PNCs should be developed.
Here, we report a new B-site engineering strategy that enables deep blue emission with a controllable wavelength from Br-based PNCs. We employ manganese cation (Mn2+) during the synthesis of PNCs. Mn2+ inhibits crystal growth, resulting in the formation of smaller particles. Additionally, as the Mn concentration increases, Mn2+ ions inhibit the growth of the [PbBr6]4- octahedra along the orthogonal direction, leading to the formation of two-dimensional (2D) structures and allowing the surface amine-based ligand to diffuse between 2D octahedral layers [Figure 1A]. The reduction in both particle size and dimensionality induces a significant blue shift in the emission spectrum, down to 435.8 nm, from pure-Br-based PNCs. The wavelength can be controlled by varying the Mn2+ concentration. These results demonstrate the potential of B-site engineering as a promising approach for developing deep blue emitters in display technologies.
Figure 1. (A) A schematic illustration of blue-emitting FA0.9GA0.1Pb1-xMnxBr3 PNCs (x = input ratio) with increasing Mn2+ concentration; (B) Photograph and (C) measured PL spectra of colloidal FA0.9GA0.1Pb1-xMnxBr3 PNC solutions under a UV lamp (excitation wavelength λex = 365 nm); (D) Photoluminescence quantum yield (PLQY) and emission wavelength of FA0.9GA0.1Pb1-xMnxBr3 PNCs (x = 0.4 and 0.9) and previously reported blue-emitting pure bromide-based PNCs[28-35]; (E) CIE coordinates of FA0.9GA0.1Pb1-xMnxBr3 PNCs with different x (black dot points) plotted on CIE chromaticity coordinates. PNCs: Perovskite nanocrystals; PL: photoluminescence; CIE: international commission on illumination.
METHODS
Synthesis
All FA0.9GA0.1Pb1-xMnxBr3 PNCs were synthesized in the air at room temperature. Precursor solutions were prepared by dissolving FABr (Dyesol), guanidinium bromide (GABr) (Dyesol), PbBr2 (TCI, 99.999%), and MnBr2 (Aldrich 98%), with the PbBr2:MnBr2 input ratio changing from 1:0 (x = 0) to 0.1:0.9 (x = 0.9) while maintaining the molarity of MnBr2:PbBr2 at 0.1 mmol and the molarity of FABr:GABr at 0.2 mmol each in 0.5 mL of anhydrous N,N-dimethylformamide (DMF) (Aldrich, 99.8%); 0.15 mL of precursor solution was then dropped into a crystallization-inducing solution comprising 5 mL of toluene (Aldrich, 99.8%), 2 mL of 1-butanol (SAMCHUN, 99.5%), 0.3 mL of oleic acid (Aldrich, 90%) and 20.4 μL of n-decylamine (Aldrich, 99.0%), which was mixed for 10 min under vigorous stirring. The resulting colloidal PNCs were washed by sequential centrifugation and then collected in 2 mL of toluene (Aldrich, 99.8%).
Characterization
X-ray diffraction (XRD) patterns were measured using a Rigaku Ultima with target Cu Kα radiation (λ = 1.54 Å) at 40 kV and 40 mA. Electronic absorption spectra were measured using A JASCO V-730 ultraviolet-visible (UV-Vis) spectrophotometer. The PL and PLQYs of PNCs were measured using a spectrofluorometer (FP-8550. Jasco Inc., Japan). PLQYs were measured using an absolute PLQY spectrometer with an integrating sphere (ILF-135) [Supplementary Scheme 1]. PL spectra time-correlated single photon counting (TCSPC) was measured using a PicoQuant FluoTime 300 EasyTau with a 405 nm pulsed laser diode. To measure the high-resolution transmission electron microscopy (HR-TEM) images of the PNCs, 0.05 mL of PNC solutions were dropped onto a copper grid and measured using a JEOL-JEM 2100F with an acceleration voltage of 200 kV.
RESULTS
Synthesis of wavelength-controllable blue-emitting FA0.9GA0.1Pb1-xMnxBr3 PNCs
We synthesized FA-GA lead bromide (FA0.9GA0.1PbBr3) PNCs and FA0.9GA0.1Pb1-xMnxBr3 PNCs by the ligand-assisted reprecipitation (LARP) method. Here, we selected FA0.9GA0.1PbBr3 PNCs because we reported that FA0.9GA0.1PbBr3 PNCs can achieve extremely high PLQY (> 90%) and high electroluminescence efficiency [external quantum efficiency (EQE) > 23.4%] in PeLEDs in our previous works[9-10]. To synthesize the PNCs, we dissolved all the precursors in the DMF solution with different Mn input molar ratios (x = 0, 0.1, 0.2, 0.4, 0.6, 0.8, and 0.9) and then injected the solutions into a toluene dispersion containing the ligands. After crystallization, we changed the solvent to toluene by sequential centrifugation (Figure 1B, see Experimental for more information)
As x increases from 0 to 0.9, PL spectra of FA0.9GA0.1Pb1-xMnxBr3 PNCs gradually blue-shift from 528.9 nm to 435.8 nm [Figure 1C]. The appearance of new peaks at the PL spectrum at ~410 nm and ~440 nm is attributed to dimensionality reduction. This will be discussed in more detail later in Section 3.3. The PL wavelength of 435.8 nm is highly blue-shifted, representing the deep blue (Royal blue) emission characteristic of pure bromide-based PNCs [Supplementary Table 1]. We also confirmed the stability and reproducibility of the deep-blue-emitting FA0.9GA0.1Pb0.1Mn0.9Br3 PNCs [Supplementary Figures 1 and 2]. Figure 1D shows the PL performance of FA0.9GA0.1Pb1-xMnxBr3 PNCs compared with previously reported literature [Supplementary Table 2]. FA0.9GA0.1Pb1-xMnxBr3 PNCs cover a wide range in the CIE diagram from pure green light to deep blue light [Figure 1E].
Size distribution and emission mechanism of FA0.9GA0.1Pb1-xMnxBr3 PNCs
Next, we investigate the mechanism that incorporation of Mn induces a huge blue-shift in PL from
Figure 2. HR-TEM images of FA0.9GA0.1Pb1-xMnxBr3 PNCs at (A) x = 0, (B) x = 0.4, and (C) x = 0.6; The size distribution histograms of FA0.9GA0.1Pb1-xMnxBr3 PNCs at (D) x = 0, (E) x = 0.4, and (F) x = 0.6. PNCs: Perovskite nanocrystals; HR-TEM: high-resolution transmission electron microscopy.
Conventionally, Mn doping introduces a d-state energy level of the Mn2+ ions to the bandgap of perovskites, inducing a transition for exciton-to-dopant energy transfer and resulting in orange-red emission from PNCs[36,37]. We also observed that Mn2+ ions produce orange emission with a PL peak at around 600 nm from Mn2+ doped CsPbCl3 PNCs [Supplementary Figure 4]. Liu et al. suggest that isovalent ions with similar bond energy can be doped into the lattices while those with different bond energy cannot be[38]. For example, the bond dissociation energy of Mn-Cl (338 kJ/mol) is similar to that of Pb-Cl (301 kJ/mol) and therefore Mn2+ is well-doped in the CsPbCl3 host. Moreover, the large bandgap (~3.1 eV) of the CsPbCl3 PNCs facilitates energy transfer from host energy level to the energy level of Mn2+, resulting in an orange light emission from d-state energy level of Mn2+.
In contrast, in Br-based PNCs, Liu et al. reported that the bond dissociation energy of the Mn-Br bond (314 kJ/mol) is much stronger than that of the Pb-Br bond (249 kJ/mol), leading to the formation of extended MnBr2 domains within PbBr2 lattices rather than doping of Mn2+ within the perovskite lattice[38]. Furthermore, FA0.9GA0.1PbBr3 PNCs have a smaller bandgap of ~2.31 eV, with a conduction band minimum (CBM) close to the d-state energy level of Mn2+, preventing energy transfer to Mn2+ and light emission from the d-state energy level of Mn2+ [Supplementary Figures 5 and 6][36,39]. Hou et al. also reported that when Mn2+ is incorporated into bromide-chloride mixed halide (CsMnyPb1-yBrxCl3-x) PNCs, orange light is not emitted while blue light is maintained due to the dominance of Br over Cl[40]. Therefore, we hypothesize that incorporation of Mn-based precursors during the growth of FA0.9GA0.1PbBr3 PNCs does not induce Mn2+-doped FA0.9GA0.1PbBr3 PNCs; rather, it forms smaller or low-dimensional FA0.9GA0.1PbBr3 crystals. In this case, Mn2+, which were not doped into FA0.9GA0.1PbBr3 crystals, suppress the growth of the PNCs, reduce the PNC size and result in blue-shifted PL.
Structural and optical properties of FA0.9GA0.1Pb1-xMnxBr3 PNCs
To investigate the effects of Mn doping on the photophysical properties, we measured the time-resolved PL (TRPL) of FA0.9GA0.1Pb1-xMnxBr3 PNCs. At x = 0, FA0.9GA0.1PbBr3 PNCs showed a PL lifetime of 58.43 ns [Figure 3A]. As x increases to 0.4, the PL lifetime decreases to 23.17 ns because smaller PNCs cause a reduction in PL lifetime[41]. This phenomenon can be attributed to enhanced spatial confinement of charge carriers and the boost of their radiative recombination[42]. FA0.9GA0.1Pb1-xMnxBr3 PNCs also showed a blue-shifted absorption spectrum, indicating that Mn doping decreases the PNC size [Figure 3B].
Figure 3. (A) PL decay profiles and (B) absorption spectra of FA0.9GA0.1Pb1-xMnxBr3 PNCs (x = 0, 0.2, 0.4); (C) PLQY and emission wavelength of FA0.9GA0.1Pb1-xMnxBr3 PNCs at 0 ≤ x ≤ 0.9. (D) XRD patterns of series of the FA0.9GA0.1Pb1-xMnxBr3 PNCs at 0 ≤ x ≤ 0.8. PL: Photoluminescence; PNCs: perovskite nanocrystals; PLQY: photoluminescence quantum yield; XRD: X-ray diffraction.
Next, we measured the PLQY of FA0.9GA0.1Pb1-xMnxBr3 PNCs with different x values (0, 0.1, 0.2, 0.4, 0.6, 0.8, and 0.9) [Figure 3C]. As x increases to 0.4, PLQY slightly decreases from 87.46% to 71.90% but remains relatively high. We attribute this decrease in PLQY to the increased surface defects due to a higher surface-to-volume ratio and reduced PNC size[42]. When x increases > 0.4, FA0.9GA0.1Pb1-xMnxBr3 PNCs showed a significant drop in PLQY (43.94% for x = 0.6, 19.03% for x = 0.8, and 6.64% for x = 0.9). The rapid reduction in PLQY is likely attributed to the increased z-axis defects, which arise from the decreased dimensionality along this axis and introduce non-radiative recombination pathways[25]. A more detailed discussion on the impact of dimensionality reduction is provided in a later section. The trend of the sudden decrease in PLQY for x > 0.4 is different from that for x < 0.4.
To investigate the reason for a significant decrease in PLQY at x > 0.4, we measured the XRD peaks of
At x ≥ 0.4, FA0.9GA0.1Pb1-xMnxBr3 PNCs exhibited sharp XRD patterns with ≈ 3.6 two theta degree spacing (10.7°, 14.3°, 17.9°, 21.5°, 25.1° and 28.7°, corresponding to the (0 0 l) planes), which are notably different from those of FA0.9GA0.1Pb1-xMnxBr3 PNCs for x < 0.4. (0 0 l) indices with sharp peaks in the PNCs indicate that crystals with low dimensionality are synthesized when a large amount of Mn2+ is incorporated during the synthesis. However, at x = 1 without Pb, FA0.9GA0.1MnBr3 PNCs did not show a sharp peak in XRD. The absence of XRD peaks and absorption/PL spectrum indicate that FA0.9GA0.1MnBr3 PNCs were not well synthesized by our LARP method. According to Bragg’s law, the interlayer spacing d of low-dimensional PNCs for x ≥ 0.4 was calculated to be 2.45 nm[44]. Using the relationship between d and alkyl chain length n of the organic ligand between Br-based low-dimensional octahedral layers [d (Å) = 8.06 + 1.59 × n][45], the value of n is calculated to be ≈ 10. This indicates that among organic ligands [decylamine (C10H21NH2), oleic acid (C18H33O2H)] added into the precursor solution, decylamine is located between the 2D PNC layers, suppressing the growth of crystals along the out of plane direction and resulting in low-dimensional PNCs.
Zhang et al. suggested that preferential binding of amine ligands to the certain facet of the perovskite crystals results in low-dimensional CsPbX3 nanowires[46]. Pan et al. revealed that ammonium ions effectively tune the growth of anisotropic perovskite structures, and shorter chain ammonium ions diffuse faster than longer chain ones, resulting in thinner platelets[47]. Therefore, we attribute the insertion of decylamine between the 2D PNC layers rather than oleic acid to the fact that decylamine has shorter alkyl length than longer oleic acid, amine-group has higher mobility than carboxylic acid, and thus decylamine diffuses between the 2D layers more easily[47,48]. In addition to the change in XRD patterns, new peaks in the PL spectrum at ~410 nm (Peak 3) and ~440 nm (Peak 2) started to appear from x = 0.4 [Figure 1C and Supplementary Figure 8]. The absorption spectrum at a short wavelength corroborates that
Mechanism of size reduction and dimensionality control
Next, we consider mechanisms by which the incorporation of Mn2+ plays a critical role in controlling the size and dimensionality of PNCs [Figure 4]. First, Mn2+ cannot be doped into the FA0.9GA0.1PbBr3 PNCs because the Mn-Br has higher bond energy than the Pb-Br bond (249 kJ/mol). Mn2+ ions, which were not doped into FA0.9GA0.1PbBr3 crystals, coordinate more strongly with n-decylammonium bromide ligands or oleate ligands than do Pb2+ ions, following the principle of the soft acid-base theory. At x < 0.4, this stronger coordination retards the diffusion of Br- ions from precursor solutions to crystals and suppresses the growth of FA0.9GA0.1PbBr3 crystals, resulting in small-sized FA0.9GA0.1PbBr3 PNCs. At x ≥ 0.4, excess Mn2+-species
CONCLUSIONS
In summary, we demonstrated highly deep blue emission with a wavelength of 435.8 nm from pure-Br-based PNCs. To achieve this, we utilized Mn2+ as a dopant of FA0.9GA0.1PbBr3 PNCs. Mn2+ inhibits crystal growth and results in the formation of smaller particles. At high Mn concentration, Mn2+ ions also inhibit the growth of the [PbBr6]4- octahedra along the orthogonal direction, allowing the organic ligands to diffuse on the low-dimensional [PbBr6]4- octahedra and resulting in low-dimensional PNCs. These both reduced particle size and dimensionality induce a huge blue shift in pure-Br-based PNCs. The emission wavelength can be controlled from 528.9 nm to 435.8 nm by varying the input Mn concentration. These findings highlight the potential of B-site engineering as a viable approach for synthesizing deep blue emitters for display technologies such as light-emitting diodes.
DECLARATIONS
Authors’ contributions
Data acquistion and analysis: Lee, S. H.; Cho, S.
Design, writing review and editing: Lee, S. H.; Cho. S.; Kim, Y. H.
Supervised the project: Yeom, B.; Kim, Y.H.
Availability of data and materials
Some results of supporting the study are presented in the Supplementary Materials. Other raw data that support the findings of this study are available from the corresponding author upon reasonable request.
Financial support and sponsorship
This work was supported by the National Research Foundation of Korea (NRF) (No. RS-2024-00408951). This research was supported by the Digital Research Innovation Institution Program Through the NRF funded by the Ministry of Science and ICT (RS-2023-00283597).
Conflicts of interest
All authors declared that there are no conflicts of interest.
Ethical approval and consent to participate
Not applicable.
Consent for publication
Not applicable.
Copyright
© The Author(s) 2025.
Supplementary Materials
REFERENCES
1. Schmidt, L. C.; Pertegás, A.; González-Carrero, S.; et al. Nontemplate synthesis of CH3NH3PbBr3 perovskite nanoparticles. J. Am. Chem. Soc. 2014, 136, 850-3.
2. Kim, Y. H.; Cho, H.; Lee, T. W. Metal halide perovskite light emitters. Proc. Natl. Acad. Sci. U. S. A. 2016, 113, 11694-702.
3. Kim, Y. H.; Kim, J. S.; Lee, T. W. Strategies to improve luminescence efficiency of metal-halide perovskites and light-emitting diodes. Adv. Mater. 2019, 31, 1804595.
4. Koscher, B. A.; Swabeck, J. K.; Bronstein, N. D.; Alivisatos, A. P. Essentially trap-free CsPbBr3 colloidal nanocrystals by postsynthetic thiocyanate surface treatment. J. Am. Chem. Soc. 2017, 139, 6566-9.
5. De, R. J.; Ibáñez, M.; Geiregat, P.; et al. Highly dynamic ligand binding and light absorption coefficient of cesium lead bromide perovskite nanocrystals. ACS. Nano. 2016, 10, 2071-81.
6. Wang, H.; Wang, W.; Tang, A.; et al. High-performance CsPb1-xSnxBr3 perovskite quantum dots for light-emitting diodes. Angew. Chem. Int. Ed. 2017, 129, 13838-42.
7. Chiba, T.; Hayashi, Y.; Ebe, H.; et al. Anion-exchange red perovskite quantum dots with ammonium iodine salts for highly efficient light-emitting devices. Nature. Photon. 2018, 12, 681-7.
8. Song, J.; Fang, T.; Li, J.; et al. Organic-inorganic hybrid passivation enables perovskite QLEDs with an EQE of 16.48%. Adv. Mater. 2018, 30, e1805409.
9. Kim, Y. H.; Park, J.; Kim, S.; et al. Exploiting the full advantages of colloidal perovskite nanocrystals for large-area efficient light-emitting diodes. Nat. Nanotechnol. 2022, 17, 590-7.
10. Kim, Y.; Kim, S.; Kakekhani, A.; et al. Comprehensive defect suppression in perovskite nanocrystals for high-efficiency light-emitting diodes. Nat. Photonics. 2021, 15, 148-55.
11. Kong, L.; Sun, Y.; Zhao, B.; et al. Fabrication of red-emitting perovskite LEDs by stabilizing their octahedral structure. Nature 2024, 631, 73-9.
12. Li, G.; Huang, J.; Zhu, H.; Li, Y.; Tang, J.; Jiang, Y. Surface ligand engineering for near-unity quantum yield inorganic halide perovskite QDs and high-performance QLEDs. Chem. Mater. 2018, 30, 6099-107.
13. Dong, Y.; Wang, Y. K.; Yuan, F.; et al. Bipolar-shell resurfacing for blue LEDs based on strongly confined perovskite quantum dots. Nat. Nanotechnol. 2020, 15, 668-74.
14. Chen, C.; Xuan, T.; Bai, W.; et al. Highly stable CsPbI3:Sr2+ nanocrystals with near-unity quantum yield enabling perovskite light-emitting diodes with an external quantum efficiency of 17.1%. Nano. Energy. 2021, 85, 106033.
15. Ren, Z.; Wang, K.; Sun, X. W.; Choy, W. C. H. Strategies toward efficient blue perovskite light‐emitting diodes. Adv. Funct. Mater. 2021, 31, 2100516.
16. Bi, C.; Wang, S.; Li, Q.; Kershaw, S. V.; Tian, J.; Rogach, A. L. Thermally stable copper(II)-doped cesium lead halide perovskite quantum dots with strong blue emission. J. Phys. Chem. Lett. 2019, 10, 943-52.
17. Liu, R.; Xu, K. Blue perovskite light-emitting diodes (LEDs): a minireview. Instrum. Sci. Technol. 2020, 48, 616-36.
18. Shao, H.; Zhai, Y.; Wu, X.; et al. High brightness blue light-emitting diodes based on CsPb(Cl/Br)3 perovskite QDs with phenethylammonium chloride passivation. Nanoscale 2020, 12, 11728-34.
19. Protesescu, L.; Yakunin, S.; Bodnarchuk, M. I.; et al. Nanocrystals of cesium lead halide perovskites (CsPbX3, X = Cl, Br, and I): novel optoelectronic materials showing bright emission with wide color gamut. Nano. Lett. 2015, 15, 3692-6.
20. He, X.; Qiu, Y.; Yang, S. Fully-inorganic trihalide perovskite nanocrystals: a new research frontier of optoelectronic materials. Adv. Mater. 2017, 29.
21. Yang, X.; Ma, L.; Yu, M.; et al. Focus on perovskite emitters in blue light-emitting diodes. Light. Sci. Appl. 2023, 12, 177.
22. Polavarapu, L.; Nickel, B.; Feldmann, J.; Urban, A. S. Advances in quantum-confined perovskite nanocrystals for optoelectronics. Adv. Energy. Mater. 2017, 7, 1700267.
23. Sichert, J. A.; Tong, Y.; Mutz, N.; et al. Quantum size effect in organometal halide perovskite nanoplatelets. Nano. Lett. 2015, 15, 6521-7.
24. Weidman, M. C.; Seitz, M.; Stranks, S. D.; Tisdale, W. A. Highly tunable colloidal perovskite nanoplatelets through variable cation, metal, and halide composition. ACS. Nano. 2016, 10, 7830-9.
25. Kumar, S.; Jagielski, J.; Yakunin, S.; et al. Efficient blue electroluminescence using quantum-confined two-dimensional perovskites. ACS. Nano. 2016, 10, 9720-9.
26. Kumar, S.; Jagielski, J.; Kallikounis, N.; et al. Ultrapure green light-emitting diodes using two-dimensional formamidinium perovskites: achieving recommendation 2020 Color coordinates. Nano. Lett. 2017, 17, 5277-84.
27. Wang, Y.; Wang, S.; Li, R.; et al. Quantum-confined perovskite nanocrystals enabled by negative catalyst strategy for efficient light-emitting diodes. Small 2024, 20, e2402825.
28. Chu, Z.; Zhao, Y.; Ma, F.; et al. Large cation ethylammonium incorporated perovskite for efficient and spectra stable blue light-emitting diodes. Nat. Commun. 2020, 11, 4165.
29. Yuan, F.; Ran, C.; Zhang, L.; et al. A Cocktail of multiple cations in inorganic halide perovskite toward efficient and highly stable blue light-emitting diodes. ACS. Energy. Lett. 2020, 5, 1062-9.
30. Jiang, Y.; Qin, C.; Cui, M.; et al. Spectra stable blue perovskite light-emitting diodes. Nat. Commun. 2019, 10, 1868.
31. Liu, Y.; Cui, J.; Du, K.; et al. Efficient blue light-emitting diodes based on quantum-confined bromide perovskite nanostructures. Nat. Photonics. 2019, 13, 760-4.
32. Zhang, M.; Zhang, J.; Zhao, G.; et al. Efficient blue CsPbBr3 perovskite nanocrystals synthesis with the assistance of zwitterionic straight chain amino acids. Colloids. Surf. A. Physicochem. Eng. Asp. 2023, 673, 131793.
33. Grandhi, G. K.; Likhit, H. M.; Ong, S. P.; Im, W. B. Jahn-Teller distortion-driven robust blue-light-emitting perovskite nanoplatelets. Appl. Mater. Today. 2020, 20, 100668.
34. Li, X.; Lou, B.; Chen, X.; et al. Deep-blue narrow-band emissive cesium europium bromide perovskite nanocrystals with record high emission efficiency for wide-color-gamut backlight displays. Mater. Horiz. 2024, 11, 1294-304.
35. Xie, Y.; Peng, B.; Bravić, I.; et al. Highly Efficient blue-emitting CsPbBr3 perovskite nanocrystals through neodymium doping. Adv. Sci. 2020, 7, 2001698.
36. Guria, A. K.; Dutta, S. K.; Adhikari, S. D.; Pradhan, N. Doping Mn2+ in lead halide perovskite nanocrystals: successes and challenges. ACS. Energy. Lett. 2017, 2, 1014-21.
37. Feldmann, S.; Gangishetty, M. K.; Bravić, I.; et al. Charge carrier localization in doped perovskite nanocrystals enhances radiative recombination. J. Am. Chem. Soc. 2021, 143, 8647-53.
38. Liu, W.; Lin, Q.; Li, H.; et al. Mn2+-doped lead halide perovskite nanocrystals with dual-color emission controlled by halide content. J. Am. Chem. Soc. 2016, 138, 14954-61.
39. Liu, Y.; Zhao, Y.; Zhu, Y.; Zhan, Y.; Matras-postolek, K.; Yang, P. Ion exchange derived CsPbBr3: Mn nanocrystals with stable and bright luminescence towards white light-emitting diodes. Mater. Res. Bull. 2022, 153, 111915.
40. Hou, S.; Gangishetty, M. K.; Quan, Q.; Congreve, D. N. Efficient blue and white perovskite light-emitting diodes via manganese doping. Joule 2018, 2, 2421-33.
41. Proppe, A. H.; Johnston, A.; Teale, S.; et al. Multication perovskite 2D/3D interfaces form via progressive dimensional reduction. Nat. Commun. 2021, 12, 3472.
42. Kim, Y. H.; Wolf, C.; Kim, Y. T.; et al. Highly efficient light-emitting diodes of colloidal metal-halide perovskite nanocrystals beyond quantum size. ACS. Nano. 2017, 11, 6586-93.
43. Slimi, B.; Mollar, M.; Ben, A. I.; Kriaa, A.; Chtourou, R.; Marí, B. Synthesis and characterization of perovskite FAPbBr3-xIx thin films for solar cells. Monatsh. Chem. 2017, 148, 835-44.
44. Yu, D.; Cai, B.; Cao, F.; et al. Cation exchange-induced dimensionality construction: from monolayered to multilayered 2D single crystal halide perovskites. Adv. Mater. Interfaces. 2017, 4, 1700441.
45. Takeoka, Y.; Asai, K.; Rikukawa, M.; Sanui, K. Systematic studies on chain lengths, halide species, and well thicknesses for lead halide layered perovskite thin films. Bull. Chem. Soc. Jpn. 2006, 79, 1607-13.
46. Zhang, D.; Eaton, S. W.; Yu, Y.; Dou, L.; Yang, P. Solution-phase synthesis of cesium lead halide perovskite nanowires. J. Am. Chem. Soc. 2015, 137, 9230-3.
47. Pan, A.; He, B.; Fan, X.; et al. Insight into the ligand-mediated synthesis of colloidal CsPbBr3 perovskite nanocrystals: the role of organic acid, base, and cesium precursors. ACS. Nano. 2016, 10, 7943-54.
Cite This Article
How to Cite
Download Citation
Export Citation File:
Type of Import
Tips on Downloading Citation
Citation Manager File Format
Type of Import
Direct Import: When the Direct Import option is selected (the default state), a dialogue box will give you the option to Save or Open the downloaded citation data. Choosing Open will either launch your citation manager or give you a choice of applications with which to use the metadata. The Save option saves the file locally for later use.
Indirect Import: When the Indirect Import option is selected, the metadata is displayed and may be copied and pasted as needed.
About This Article
Special Issue
Copyright
Data & Comments
Data
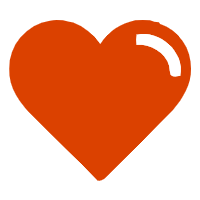
Comments
Comments must be written in English. Spam, offensive content, impersonation, and private information will not be permitted. If any comment is reported and identified as inappropriate content by OAE staff, the comment will be removed without notice. If you have any queries or need any help, please contact us at [email protected].