A review on Mg-based metallic glasses for biomedical scaffolds: experimental and computational modeling
Abstract
Magnesium (Mg)-based metallic glasses have emerged as a promising class of biomaterials for various biomedical applications due to their unique properties, such as high strength-to-weight ratio, good biocompatibility and biodegradability. The development of Mg-based metallic glass scaffolds is of particular interest for tissue engineering and regenerative medicine applications. However, the rate of biodegradability of the materials is not well controlled and requires extensive research for efficient tissue/bone regeneration. This review provides a comprehensive overview of the recent advancements in the development of Mg-based metallic glass scaffolds and their tuneable biodegradability with different compositions and thin film coatings. It discusses the structural and biological properties, mechanical and biodegradation behavior, and various fabrication techniques employed to produce Mg-based bulk metallic glass scaffolds. Furthermore, the review explores surface modification of permanent implants with Mg-based thin film biodegradable metallic glasses to simulate tissue regeneration on the implants. Optimization of scaffold design to increase tissue growth and healing by understanding the complex interactions between the scaffold and biological tissues and predicting the long-term implant behavior using computational models are reviewed. The challenges and future research directions in this field are also discussed, providing insights into the potential of Mg-based metallic glass scaffolds for various biomedical applications, including bone tissue engineering, wound healing, and cardiovascular implants.
Keywords
INTRODUCTION
Scaffolds are biomaterials and bioimplants that stimulate tissue growth and facilitate the repair or reconstruction of diseased body parts[1]. In bone tissue engineering (BTE), scaffolds made of conventional materials such as metals, polymers, ceramics, or natural materials are introduced into the site of a bone defect to promote repair and regrowth[2,3]. While polymer or ceramic scaffolds are common in orthopedics, their limitations are significant[4-6]. These implants can trigger a foreign body reaction and deter integration with bone tissue, hindering successful repair[7-9].
Magnesium (Mg)-mediated calcitonin gene-related peptide (CGRP) mechanisms are promising to enhance bone regeneration by integrating the osteoinductive properties of Mg with the regulatory effects of CGRP on bone remodeling[10]. As a biodegradable material, Mg releases magnesium ions (Mg2+) during its degradation, which stimulate osteoblast differentiation, enhance extracellular matrix production, and promote angiogenesis, thereby improving oxygen and nutrient delivery to regenerating bone tissue[11]. It also activates key signaling pathways that contribute to bone mineralization and formation, establishing its role as a potent material for BTE[12]. Furthermore, Mg implants can induce localized CGRP release, enhancing vascularization and immune regulation at the injury site to optimize bone healing[13]. CGRP, a neuropeptide released by sensory nerves, complements the effects of Mg by promoting osteoblast proliferation, enhancing bone formation, and inhibiting osteoclast activity to reduce bone resorption[14]. It also modulates the inflammatory response, creating a balanced microenvironment conducive to tissue repair[15]. Integrated Mg and CGRP synergistically enhance bone regeneration by amplifying vascularization, stimulating osteoblast activity, and preventing excessive bone resorption[14]. This dual-action mechanism ensures efficient and balanced bone remodeling, offering a robust strategy for treating fractures, bone defects, and other skeletal injuries[16]. Mg and CGRP provide a powerful foundation for advancing BTE and regenerative medicine[17-20].
Some biodegradable metals such as Fe and Zn possess much higher Young’s modulus (higher stiffness) compared to bone which could lead to or contribute to stress shielding if the scaffold is not appropriately designed[21-23]. As a result, the implant bears most of the load, weakening the surrounding bone and suffering from poor plasticity, making it unsuitable for most medical (orthopedic) applications[24-26]. Mg is a vital mineral in the human body, essential for various physiological processes and a promising biodegradable implant scaffold material due to its biocompatibility, osteoconductivity, and mechanical properties that are more similar to natural bone that contributes less to stress shielding than many other metals[27-29]. Since its first use as wire ligature in 1878, magnesium has been proposed for biomedical applications[30].
As a biomaterial, it offers clinical advantages by dissolving in a controlled manner within the body
To address these limitations, magnesium alloys have been developed by adding elements such as aluminum (Al), zinc (Zn), calcium (Ca), or rare earth metals to the pure metal[33-35]. These alloys typically exhibit improved mechanical strength, corrosion resistance, and more controlled degradation rates, making them more suitable for load-bearing applications[33]. However, the biocompatibility of these alloys can vary depending on the added elements, and there is a risk that some alloying elements could introduce toxicity or unwanted side effects[33]. Furthermore, while alloying can improve the properties of Mg, it may still not fully address issues such as stress shielding or the precise control of the degradation rate, which are critical for matching the performance of scaffolds to the tissue healing process[33].
Bulk metallic glasses (BMGs) represent an advanced class of materials explored as load-bearing implants in biomedical applications due to their exceptional strength, flexibility, and corrosion resistance[33]. They exhibit an amorphous structure, lacking the regular atomic arrangement found in crystalline metallic alloys[36]. Mg-based BMGs (Mg-BMGs) are particularly attractive for load-bearing biodegradable implant scaffolds, offering the mechanical characteristics of bone[37-39]. The lower modulus of the Mg-BMGs provides a better match to the modulus of cortical bone and helps reduce stress shielding, a beneficial feature for hard-tissue prostheses[40]. In addition, Mg-based metallic glass has an improved corrosion resistance compared to crystalline Mg and its alloys[41,42]. These properties make Mg-BMGs particularly attractive for scaffolds with tailored properties including degradation rates[33]. Tissue growth and cell infiltration that are crucial for osseointegration and the fusion of implant and bone can be enhanced through the coating of porous materials that require further studies[39]. This includes surface engineering of implants with an interlayer of Mg-based thin film metallic glass (TFMGs) coatings to simulate tissue regeneration on desired permanent implant substrates[43-45] and thin film.
BMGs are advanced materials with an amorphous atomic structure, formed by rapidly cooling metal alloys to prevent crystallization. They offer exceptional properties such as high strength, elasticity, corrosion and wear resistance, and ease of processing in their supercooled liquid state. Common BMG systems include
Figure 1. BMGs, including Ti-, Zr-, Fe-, Mg-, Zn-, Ca- and Sr-based alloying systems. Reproduced with permission from ref.[46] © 2016 Acta Materialia Inc. Published by Elsevier Ltd. All rights reserved. BMGs: Bulk metallic glasses.
The discovery of BMGs sparked significant scientific and technological interest due to their potential application[47]. Since the mid-20th century, scientists and engineers have been researching suitable properties of metallic glasses through diverse manufacturing techniques to overcome their limitations[48-50]. BMGs are formed from rapidly cooled molten alloys that inhibit the formation of crystalline nuclei, resulting in amorphous materials[46]. The cooling process for the formation of amorphous material includes water quenching[46,51], melt spinning[52], gas atomization[53], etc. In 1960, Klement et al. reported the first BMG[54] by rapidly cooling specific combinations of multi-elements (primarily metals) below the glass transition temperature (Tg) to prevent the formation of crystalline materials[44]. Figure 2 describes this phenomenon, illustrating the supercooled liquid region between the melting temperatures (Tm) and Tg over periods not more than the time required for crystal nucleation. These multicomponent (> 4 elements) metallic glasses are commonly based on Zn, Au, Mg, Fe, Zr, Ti, etc.[46]. However, ternary component metallic glass from
Figure 2. Fabrication concept of metallic glass: Schematic of continuous cooling transformation (CCT) curve diagram for the onset of crystallization of a glass-forming liquid. Between Tm and Tg, (arrow 1; normal cooling Nc) depicts the crystallization phase which can be avoided with the cooling rate (Rc; arrow 2) ≥ critical cooling rate (Rc; arrow 3) to conserve the macroscopically disordered structure of the liquid at the Tg. This image is licensed under Creative Commons Attribution and reproduced from ref.[61] © 2024 Onyeagba C. Raphael. All rights reserved.
BMGs appear as atomically frozen (solidified) liquid due to no long-range atomic order[47,61]. They can also be fabricated using various casting techniques designed for rapid cooling of molten alloys to prevent crystallization, maintaining an amorphous structure[61]. Techniques include suction casting, which rapidly draws molten alloy into a pre-cooled mold under vacuum[47]; injection casting, where molten metal is injected under high pressure to form complex shapes[53]; and copper mold casting, which uses a copper mold to quickly extract heat and solidify the alloy[52,62-65]. These methods are constrained by the cooling rates (Rc) shown in Figure 2. To form a fully amorphous structure, BMGs require extremely high Rc, typically between 103 and 106 K/s, beyond the Tg [Figure 2] to prevent crystallization[66]. Conventional casting methods achieve Rc of only up to 102 K/s, often insufficient to avoid crystalline phase formation[66]. While techniques, such as melt spinning and splat quenching, can reach Rc of 104 to 106 K/s, they are limited to producing thin ribbons or flakes[66]. Alternative fabrication methods, such as 3D printing and laser processing, can achieve the necessary high Rc and avail the benefit of complex geometries and tailored properties[67]. In 3D printing, techniques such as selective laser melting (SLM) or laser powder bed fusion (LPBF) are employed, where a laser selectively melts and fuses layers of metallic powder, allowing the creation of intricate BMG structures layer by layer[68,69]. This method provides precise control over the design and material distribution, making it ideal for custom applications[51,70]. However, 3D printing of crack-free BMG components remains a challenge due to the generation of structural heterogeneity and crystallization during the rapid solidification process[71-73]. Ultimately, the choice of fabrication techniques depends on the desired size, shape, and properties of the component.
Research on the reliability, rigidity, and dynamic mechanical characteristics of BMGs dates to the early 1970s, with most early examples being low-dimensional, such as amorphous ribbons and wires[44]. The toughness of some BMGs approaches the values of crystalline metals, significantly exceeding that of ceramics. Composite BMGs, which consist of crystals embedded in an amorphous matrix, can rival the toughness of their crystalline counterparts while retaining the benefits of the glassy structure[44,45]. Additionally, their unique thermal properties, such as a distinct Tg, allow for precision shaping and molding without crystallization [Figure 2].
Figure 3 shows the fracture toughness and yield strength of a range of BMGs from the literature in comparison to polymers, ceramics, steel, and titanium alloys[74]. BMGs occupy a distinctive region in the Ashby maps, characterized by their exceptionally high yield strength and moderate fracture toughness that were not achievable from the other materials.
Figure 3. (A) Ashby map of fracture toughness versus density of materials including the location of select bulk metallic glasses and Zr-Ti-Nb-Cu-Be bulk metallic glass matrix composites; Composite alloys have among the highest fracture toughness (B) Ashby map of fracture toughness versus yield strength of materials including selected metallic glasses and composites. Contour lines indicate plastic zone size, and the shaded region signifies the diameter (d) < 1 mm region that typically confines monolithic metallic glasses. The plastic zone size of the toughened composites is shown in the inset. This image is reproduced from ref.[74] Copyright © 2013 Douglas C. Hofmann. All rights reserved.
The development of BMG matrix composites (BMGMCs) has been a key focus, which involves incorporating ductile phases into the brittle BMG matrix to improve their mechanical properties[75]. BMGs are expected to see widespread applications in consumer electronics, jewelry, fuel cells, coatings, and nano/microtechnology due to an enhanced understanding of their properties and processing potential[74]. Ashby maps for fracture toughness versus density [Figure 3A] and yield strength [Figure 3B] highlight that these amorphous alloys and composites compare favorably with other engineering materials, although the plastic zone size (1-10 μm) limits BMG applications as the deformation is not spread evenly in thicker (> 1mm) BMGs and leads to brittle failure under stress[76,77].
BMGs typically have moderate fracture toughness compared to more ductile materials (e.g., metals) due to their lack of plastic deformation mechanisms that can lead to brittle fracture under certain conditions[78,79]. Despite this, some BMGs demonstrate a good balance between strength and toughness, making them suitable for applications requiring high strength and moderate toughness[79]. Their position on the Ashby map [Figure 3B] highlights their role as materials that provide high strength with adequate toughness, bridging the gap between brittle ceramics and ductile crystalline metals[79]. Mg-BMGs can be categorized under new BMGs and exhibit a unique combination of properties on the Ashby map, particularly in terms of their high yield strength and moderate fracture toughness, making them competitive with other lightweight structural materials[80].
This review focuses on the latest research on bulk Mg-based metallic glasses (Mg-MGs) as tailored scaffolds or thin films as tissue regenerative interlayers on permanent implants, focusing on their Experimental aspects (synthesis, structural/mechanical properties, biocompatibility, biodegradability) and Computational Modeling of Mg-MGs in both thin films and bulk properties for biomedical scaffolds with an emphasis on controlling corrosion rate for tissue regeneration. The challenges and future directions in this field are also discussed.
MG-BASED BULK METALLIC GLASSES
Processing and Properties of Mg-based BMG
Research has demonstrated that magnesium is hypoallergenic and promotes the generation of new bone as evidenced by both in vitro and in vivo experiments[81]. It is one of the few load-bearing light metals having a density of 1.74 g/cm3 and modulus of elasticity (45 GPa) closest to bone (23 GPa). However, magnesium has limited solubility for other elements, which limits processing methods. Its crystal structure [hexagonal close-packed (HCP)] restricts deformation and affects the toughness required in biomedical implants. Extensive research has been conducted to create Mg-based scaffolds for temporary implants such as screws, pins, and stents by incorporating well-known biocompatible alloying elements (Ti, Ca, Zn, etc.)[46].
Figure 4 shows steps for manufacturing porous Mg-based alloy for scaffolds in animal bone implants. Fabricating materials as a porous scaffold facilitates cell/tissue regrowth which makes them more suitable for biomedical implants[82].
Figure 4. Step 1: the 3D entangled titanium wire material (A and E) was prepared with Ti wires. Step 2: the Ti-Mg composite (B and F) was prepared with high-purity Mg melts. Step 3: Ti wires were removed by HF solution and an open-porous magnesium scaffold (C and G) was successfully manufactured. Step4:open-porous magnesium scaffolds were implanted into the lateral epicondyle of rabbits (D). Image reproduced from ref.[82] licensed under a Creative Commons Attribution 3.0 unported license, Copyright © 2016, Meng-qi Cheng.
However, traditional alloying methods are not very effective in improving properties such as elasticity and chemical behavior in magnesium[83]. This is because magnesium has limited solubility for other elements and a crystal structure that restricts its ability to deform (ductility). Adding fibers as reinforcement was found to overcome these limitations [Figure 5A][83]. As shown in Figure 3, BMGs have limited ductility[48,84], and require improved fabrication and processing techniques to enhance their properties. The structure of the Mg-based binary or ternary alloys can be modified by introducing and substituting elements in matrix systems, as shown in Figure 5B[85,86].
Figure 5. (A) Directions of metallic glass matrix/alloy development; (B) Type of alloys, microstructure modifications, surface treatments, and biological effects of Mg-based scaffolds. The figure is licensed under CC-BY 4.0 and reproduced from ref.[88] with permission © 2022 by the authors. Licensee MDPI, Basel, Switzerland.
Mg-MGs can be processed using commonly known manufacturing methods[87,88]. Table 1 displays the production of various Mg-BMGs throughout time, utilizing diverse processes. Combining Mg-based metallic glass with mesoporous silica nanocomposites boosts biocompatibility and BTE capabilities[89]. Alloying Mg with elements such as Zn, Ni, and Ca enhances glass-forming ability and mechanical properties, making the material more suitable for biomedical applications[90-94]. For instance, the addition of a small amount of Ni could improve the glass-forming ability of an Mg-Cu-Y matrix[86]. Manufacturing
Various fabrication methods and critical size of different Mg-based BMGs
Mg-based BMGs composition | Fabrication method | Critical diameter | Post-production condition | Ref. |
Mg-Zn-Ca | Induction furnace under an inert atmosphere, cast in copper mold | 5 mm rod | Fully amorphous phase | [92] |
Mg-Zn-Ca-Sr | Copper mold casting | 4~6 mm rod | Fully amorphous phase after 5hr of milling | [93] |
Mg-Ca-Zn | Induction furnace under an inert atmosphere | 3-4 mm rod | Mixture of crystalline and amorphous phase | [94] |
Mg-Cu-Ag-Er | Copper mold casting | 8-10 mm rod | Fully amorphous phase | [95] |
Mg-Y-Cu-Ag-Pd | Water quenching | 12 mm rod | Fully amorphous phase | [96] |
Mg-Ni-Nd | Chill-block melt-spinning | 1 mm ribbons | Fully amorphous phase | [97] |
Mg-Cu-Ni-Ag-Zn-Y-Gd | Copper mold casting method | 14 mm ribbons | Fully amorphous phase | [98] |
Mg-Cu-Y-Zn | Induction furnace under an inert atmosphere | 3 mm rod | Fully amorphous phase | [85] |
Mg-Cu-Ni-Gd | Copper mold casting | 25-50 mm rod | Fully amorphous phase | [102] |
As previously emphasized, Mg-BMGs are highly significant due to their exceptional properties. This amorphous nature of BMG allows for a broad range of glassy compositions without specific stoichiometric requirements, facilitating microscopic property adjustments within a defined range through optimization of the glass transition composition[95-99]. The atomic structures of five types of Mg-BMGs using neutron diffraction and classical molecular simulation methods were explored by Gulenko et al.[100] and the findings indicated that bond lengths remained relatively stable despite changes in composition. A similar study investigated the structure of multicomponent Mg-MGs using techniques such as high-resolution transmission electron microscopy, and reverse Monte Carlo modeling[101]. They focused on the
Figure 6. Schematic illustration of the Mg-based BMGs (Mg65Cu20Y10Ni5) developed using the reverse Monte Carlo modeling shows (A) simulation box; (B) elemental distribution; and (C and D) short-range-order (SRO) region formation because of clustering. The figure is licensed under CC-BY 4.0 and reproduced from ref.[101] © 2017 Babilas et al.; licensee Beilstein-Institute.
Mg-BMGs are promising candidates for temporary implants such as orthopedic implants and cardiovascular stents owing to the following properties and advantages compared to their crystalline counterparts and other bioabsorbable implants. Mg-BMGs combine their lightweight nature and biodegradability with tailored elastic properties compared to pure magnesium, enhancing their toughness[32,102]. While Mg-BMGs generally have superior mechanical properties, such as compressive strength, compared to conventional cast magnesium alloys, their performance can be influenced by factors such as sample size, casting defects, and alloying elements which can improve glass-forming ability and plasticity[103,104]. Tuning the properties makes such materials ideal for temporary implant devices, such as bone fixation screws or stents, to gradually dissolve in the body, eliminating the need for secondary surgeries to remove them. Engineering of the Mg-BMGs to degrade at controlled rates and matching the healing process of tissues remains challenging. Thus, fine-tuning the characteristics of these materials as scaffolds and ensuring long-term clinical use requires further research and optimization. This section systematically analyses the requisite attributes of Mg-MGs, such as biocompatibility, biodegradability, mechanical properties, and other attributes, that make them excellent for stimulating tissue regeneration and scaffolding.
Biocompatibility: Mg is an essential element for human health. Mg-BMGs exhibit excellent biocompatibility, minimizing the risk of adverse tissue reactions. Due to the intrinsic biocompatibility, metallic glass of Mg attracts significant interest in the biomedical field[105,106]. It has garnered considerable attention in the biomedical field, primarily because of magnesium’s indispensable function in the human body, specifically with bone health[105,107,108]. In-vivo and in-vitro research on Mg-Zn-Ca BMGs resulted in good biocompatibility no toxicity to cells and minimal bio-destruction[109].
Biodegradability: Unlike permanent implants, Mg-based scaffolds degrade over time, allowing the diseased body part to regrow in its place[110,111]. Magnesium implants degrade slowly in dry air but relatively quickly in biological fluids, with pure magnesium corroding at rates of 0.5 mm to 1 mm per month[112-119]. Magnesium alloys, such as AZ91D, WE43, and AM60, exhibit slower degradation rates, approximately 0.1 mm to
Mechanical Properties: Mg-BMGs possess mechanical properties comparable to bone that reduce the risk of stress shielding, a complication observed with stiffer implant materials[127]. Most research on the creation of biodegradable magnesium alloys for use as biomaterials has focused on crystalline alloys[25]. The amorphous biodegradable magnesium structure provides superior mechanical properties: low density, high strength and elasticity, which are crucial for biomedical applications with respect to the regulation of the degradation rate and stress shielding[128-130].
The composition of this biocompatible Mg-BMG can be adjusted to achieve desired degradation rates and mechanical properties[131]. The degradation rate of Mg-BMGs is controllable through compositional changes and surface treatments, allowing for gradual scaffold dissolution that aligns with tissue regeneration timelines[132,133]. Porosity and pore structure can be finely tuned to enhance cell infiltration and vascularization, crucial for effective tissue integration[133-135]. Surface properties, including chemistry and wettability, can be modified to promote better cell adhesion and bioactivity[136,137]. Additionally, scaffolds can be functionalized with bioactive agents such as growth factors or antibiotics to support specific healing processes and reduce infection risks[120,121,138,139]. Overall, these tailorable properties enable Mg-BMG scaffolds to be engineered for optimal performance across a range of medical applications, from bone regeneration to wound healing and cardiovascular implants.
Figure 7 shows an anticipated 10-year progression and demand for magnesium based on their history[83,140]. Researchers have explored various Mg-based alloy scaffolds created using laser-based additive manufacturing techniques. Li et al.[141] examined WE43 Mg alloy scaffolds produced via LPBF, finding that increasing the strut diameter to 800 μm enhanced the elastic modulus from 0.2 GPa to 0.8 GPa. They also found that plasma electrolytic oxidation treatments reduced the corrosion rate to approximately
Figure 7. Increasing the capacity and demand for magnesium. This image is reproduced from ref.[140] © Copyright 2023, All rights reserved. Vision Research Reports.
In another set of studies, researchers investigated Mg-based composites created through laser additive manufacturing. Yang et al.[143] developed bioglass-reinforced Mg-based composites that showed a refined and homogenized structure, an enhanced corrosion rate, and good biocompatibility. Yao et al.[144] used SLM to produce binary and ternary Mg alloys, improving microhardness and corrosion resistance. Xu et al.[145] enhanced the biodegradation resistance of ZK30 + Cu alloys through grain refinement, which also imparted antibacterial properties due to the copper content. Yin et al.[146] studied ZK30/bioactive glass composites, concluding that the addition of bioactive glass improved corrosion resistance, microhardness, and biocompatibility. Shuai et al.[147] created an antibacterial ZK60-Cu(x) alloy, finding that increasing the Cu content boosted antibacterial properties while maintaining good cytocompatibility. In recent years, advancements have been made in producing large quantities of metallic glasses. A key strategy for creating BMGs involves selecting elements with significant differences in atomic size. This complex atomic structure hinders crystallization during cooling, allowing for the formation of thicker, amorphous materials. BMGs come in a diverse range of chemical compositions, offering the potential to tailor their mechanical, magnetic, chemical, and biological properties for specific applications[52].
Applications of Mg-based BMG
Table 2 describes several critical design criteria that metallic implants must meet to be suitable for biomedical applications, especially cardiovascular, orthopedic, and dental applications.
Design criteria for biodegradable metallic implant devices in cardiovascular and orthopedic applications achievable by new BMGs according to Ashby map
Criteria | Orthopedic internal fixation device | Cardiovascular stent | Ref. |
Mechanical properties | Tensile strength > 300 MPa Yield strength > 230 MPa Elongation to failure > 15%-18% Elastic modulus approx. to that of cortical bone (10-20 GPa) | Tensile strength > 300 MPa Yield strength > 200 MPa Elongation to failure > 15%-18% | [127,130,152,153] |
Biocompatibility | Non-inflammatory, non-toxic, hypoallergenic. No particle retention or harmful release. Promotes osteoclast and osteoblast attachment. Prevent fibrous encapsulation | Non-toxic hypoallergenic. No retention of particles or harmful release. Avoid smooth muscle cell attachment and promote endothelial cell attachment | [154] |
Corrosion behavior | Hydrogen evolution < 10 μL/cm2/day Screws and plates (0.2-0.5 mm year-1) | Hydrogen evolution < 10 μL/cm2/day Penetration rate < 20 μm year-1 | [131,150] |
Mechanical integrity and resorption | Osteotomy staples < 3 months Full absorption 1-2 years Screws and Plates < 6 months | Mechanical integrity (UTS) 3-6 months Full absorption 1-2 years | [151,155] |
Magnesium alloys are considered for load-bearing implant devices such as plates, screws, and pins for bone fracture repair, as their elastic modulus is closer to natural bone compared to other metals used for implants[148,149]. Firstly, the corrosion rate and associated hydrogen evolution must be carefully controlled, with a degradation target value typically less than 10 μL/cm2/day to avoid complications[150]. The penetration rate of the corrosion process should also be limited to less than 20 μm/year to maintain the mechanical integrity of the implant over the required service life[151]. Additionally, the biodegradation products released during the corrosion process must be biocompatible and not elicit adverse biological responses.
The mechanical properties of the biodegradable metal, such as strength, ductility, and fatigue resistance, need to be tailored to match the specific requirements of the target application, whether for load-bearing orthopedic devices or flexible cardiovascular stents[152,153]. Surface modifications further enhance the corrosion resistance, biocompatibility, and osseointegration of the implant[154]. Meeting these multifaceted design criteria is crucial to developing safe and effective biodegradable metallic implants for use in the human body.
Mg-alloys possess light weight, strength, biodegradability, and biocompatibility, making them excellent interfaces for orthopedic implants as they minimize stress shielding and associated effects[155,156]. However, the rapid degradation and hydrogen gas evolution of Mg-based implants in the physiological environment is a major obstacle, leading to rapid loss of mechanical integrity and potential adverse effects such as local gas cavities, alkalization, and Mg2+ enrichment[157-159]. By-products such as Mg2+, hydrogen gas (H2), and hydroxide ions (OH-), can disrupt the surrounding microenvironment[80]. Excessive Mg2+ release may cause local ion overload, pH shifts, and cytotoxicity, while hydrogen gas accumulation can form cavities that hinder tissue integration[160]. These effects can impair cellular activity, bone regeneration, and scaffold biocompatibility. Strategies such as surface coatings, pH-buffering materials, and alloying with elements such as Ca and Zn are being explored to control the corrosion rate and reduce toxicity[11,161,162]. The low corrosion resistance of Mg alloys is also related to heavy metal contamination and the formation of galvanic couples[163]. The position of Mg-BMGs on the Ashby map [Figure 3B] highlights their balance of strength, toughness, and low density, making them suitable for biomedical and lightweight structural applications[164]. However, their fracture toughness is generally lower than some crystalline metals, which can limit their use in more demanding structural applications[80,165,166]. Corrosion-induced defects, such as pitting and stress corrosion cracking, compromise the load-bearing capability of the scaffold, particularly under physiological stress[167,168]. Optimized scaffold designs with hierarchical porosity, mechanical reinforcement, and corrosion-resistant alloys are essential to improve structural stability and prolong functional performance during the critical healing period[151].
There are also technical barriers and challenges in adopting Mg-based scaffolds, such as improved manufacturing methods, high cost, and a better understanding of their performance[169]. To address these issues, the design of new metallic glass composites and surface modification of magnesium and its alloys with biocompatible nanostructured thin film protective coatings and treatments help slow degradation and improve their hemocompatibility and cytocompatibility[170-172]. Fabricating the material as a porous scaffold facilitates cell growth, and using Mg-based metallic glass as a thin film coating on permanent implants can improve tissue regeneration and growth. Alternatively, a protective thin film coating from biodegradable materials on an Mg-based scaffold can improve its corrosion resistance.
MG-BASED THIN FILM METALLIC GLASSES
TFMGs are a separate category of metallic glasses with a much smaller scale (micrometers or nanometres) and present controllable properties compared to bulk materials, making them a promising candidate for tuneable biodegradable implants[43]. Metallurgical modification including microstructure and composition optimization through alloying and thin film processes can be used to control Mg degradation with respect to its specific application[156,173]. Mg-based TFMGs avail improved surface bioactivity, greater tissue integration, and regulated degradation rates[174-176], thereby controlling the hydrogen gas evolution[177,178]. The surface of conventional implants can be modified with Mg-based TFMG coating to enhance tissue regrowth[156] through the biodegradability of the thin film. TFMG can be fabricated with techniques such as sol-gel, dip coating, chemical vapor deposition (CVD), Physical Vapor Deposition (PVD), and pulsed laser deposition (PLD)[179].
The PVD-sputter system [Figure 7] is a robust and flexible technique for TFMG fabrication. It involves a vacuum deposition where the material is transferred from a solid state to a vapor or atomic state before depositing it onto a substrate. Sputtering is an effective and cost-efficient PVD method for depositing thin films[180]. It is a flexible and high-throughput deposition process that can produce high-quality thin films[181]. Sputtering is scalable and can be used for large-area depositions, making it cost-effective for industrial production[182,183]. The deposition rate in sputtering can be easily controlled and optimized for high efficiency with a wide range of material options, including metals, alloys, and compounds, allowing for the fabrication of diverse thin film structures[184,185,186]. Thin films deposited by sputtering can help reduce costs and miniaturize devices, providing effective protection and functionalization of materials, and making them useful for various applications[187,188].
PVD co-sputtering excels in thin film fabrication due to its versatility and precision. It allows simultaneous sputtering of multiple targets, enabling the deposition of complex multicomponent films [Figure 8] including metallic and polymorphic metallic glasses from a wide range of materials[44,189,190]. This technique provides fine control over film composition, thickness, and uniformity, ensuring high-quality, defect-free films with excellent adhesion[44]. Co-sputtering is efficient and scalable, suitable for large-scale production, and adaptable to various temperatures and integration with other processes. The method operates in a vacuum, minimizing contamination, and generating minimal waste, offering both environmental and economic benefits[191].
Figure 8. Schematic illustration of the magnetron sputtering process revealing the bombardment of the substrate surface with sputtered atoms from the target to create a thin film. The figure is licensed under CC-BY 4.0 and reproduced from ref.[44] © 2023 C.R. Onyeagba,M. Valashani,H. Wang,C. Brown,P. Yarlagadda,T. Tesfamichael. Published by Elsevier B.V.
Table 3 shows a comparison between Mg-based implant scaffolds and those scaffolds coated with TFMGs. From Table 3, we can see the role of the thin film in improving the attributes and performance of the scaffolds. TFMG coatings improve the degradability and microstructure of Mg-based implant surfaces by hindering the subcutaneous gas pockets during the healing period[192,193]. The amorphous nature of the metallic glasses offers better resistance to localized corrosion and stress corrosion cracking, which are common issues in biomedical environments.
Comparison of Magnesium-based BMG scaffolds and TFMG coated scaffolds[43]
Aspect | Magnesium-based scaffolds | TFMG-coated Magnesium-based scaffolds |
Material | Pure magnesium is biomaterial and biodegradable | Material selection of the TFMG composition is challenging (e.g., MgZnCa, MgZnZr, Mg-Zr-Ca-Sr-Sn, etc.) |
Corrosion resistance | Scaffold corrodes relatively quickly in physiological environments | Improved corrosion resistance of scaffold due to the protective TFMG layer |
Mechanical properties | Good for load-bearing scaffolds but properties affected by rapid degradation | Thin film coating maintained the base metal’s mechanical properties for the scaffold’s life |
Cell growth | May require additional surface treatments before the implantation of scaffolds for optimal cell growth | Generally engineered surface morphology during coating suitable for cell adhesion and growth |
Degradation control | Design of the degradation rate to match tissue healing is challenging | The TFMG coating maintains its protective qualities throughout the degradation process |
Toxicity | The rapid degradation of Mg can lead to localized high pH environments affecting surrounding tissues | The slow degradation rate of the TFMG coating reduces tissue reaction and release of toxic elements |
Manufacturing complexity | It is a relatively straightforward manufacturing process but may require post-processing | Increased complexity due to the need for precise control over the coating surface morphology and process to ensure film uniformity and adherence with the base metal |
Table 4 shows Mg-based thin films including metallic glasses with improved corrosion resistance and other properties compared to pure metal[194-196]. One of the promising scaffolds with great biocompatibility and less toxicity to tissue cells is Mg-Zn-Ca metallic glass. The addition of Ca forms fine Mg2Ca precipitates, enhancing the mechanical properties through precipitation hardening[197,198]. Moreover, as shown in Table 4 the corrosion resistance of Mg (Ecorr -1.886, Icorr 86.06 × 10-6)[199] improved significantly after alloying with other elements (Nd, Zn, Zr, Ca, Sr, Sn, Yb, Ag, Cu, Gd, Ag)[200-205], for example, Mg-Zn-Ca with higher Ecorr -0.07 and lower Icorr 0.26 × 10-6)[201].
Potential and current density of Mg-based thin films deduced from polarization curves
Sample | Ecorr (V) | Icorr (A/cm2) | Electrolyte | Ref. |
Pure Mg | -1.886 | 86.06 × 10-6 | SBF | [199] |
Mg-Nd-Zn-Zr | -1.659 | 1.718 × 10-6 | SBF | [200] |
Mg-Zn-Ca | -0.07 | 0.26 × 10-6 | NaCl | [201] |
Mg-Zr-O | 1.43 | 0.71× 10-6 | K2ZrF6 | [202] |
Mg-Zr-Ca | -1.89 | 0.51× 10-6 | SBF | [203] |
Mg-Zr-Ca-Sr-Sn | -1.78 | 0.04 × 10-6 | SBF | [203] |
Mg-Zn-Yb-Ag | -1186 | 5.43 × 10-6 | SBF | [204] |
Mg-Cu-Gd-Ag | -1.06 | 0.54 × 10-6 | NaCl | [205] |
The inclusion of Zn in Mg improves tensile and creep strength, reduces grain size, and enhances the alloy’s castability[198]. Zn is solubilized up to 2 wt% in Mg at room temperature in the equilibrium state. When the Ca and Zn concentrations are optimized in the Mg-Ca-Zn system, they exhibit a favorable combination of degradation mitigation/control, mechanical properties, and biocompatibility. Figure 9 shows the corrosion characteristics of Mg TFMGs (Mg-Zn-Ca) with different Zn compositions studied by Li et al.[206]. Mg-Zn-Ca TFMGs exhibited better corrosion resistance than pure crystalline Mg, and when the Zn content exceeded 50%, the films showed passivation behavior for Mg49.3Ca8.5Zn42.2 [Figure 9].
Figure 9. Tafel plot of Mg-Ca-Zn TFMG and pure Mg. reproduced with permission from reference[206] © Copyright Royal Society of Chemistry 2024. TFMGs: Thin film metallic glass.
Polarization studies were conducted in simulated body fluid (SBF), NaCl, K2ZrF6 solution electrolyte at room temperature and show that a thin film coating can improve the corrosion/degradability of Mg-BMG composite[179,201,202].
Figure 10 shows the scanning electron microscopy (SEM) before and after the corrosion tests of the
Figure 10. Scanning electron microscopic images for the uncoated and 4 and 6 mm Mg-Zn-Ca TFMG-coated Mg samples fabricated via magnetron sputtering process before and after polarization: (A) uncoated with (i) magnification by 600 × and (ii) magnification by
The interplay between coating thickness, process parameters, and degradation rate is complex, and a systematic approach is required to understand and optimize the performance of Mg-based thin films for biomedical applications. Zhao et al.[207] provide a comprehensive review of the mechanisms, classification, modeling, and experimental testing of Mg corrosion in bio-applications. The coating thickness plays a crucial role in the degradation rate of Mg-based materials. Thinner coatings may be more effective in controlling the degradation rate, as Tong et al.[208] demonstrated that the corrosion rate of bulk Mg alloy samples accelerated with increasing layer thickness. The coating process parameters can significantly influence the microstructure, mechanical properties, and corrosion resistance of Mg-based thin films. Moreover, the layer thickness was found to influence the formation quality, microstructure, and mechanical properties of the Mg alloy samples[208]. The various coating techniques have been explored to control the degradation rate of Mg-based materials, including sol-gel TiO2 coatings[209], biomimetic silk coatings[210], chemical conversion coatings such as MgF2 and MgF2-MgPO4[211], and PVD coatings such as TiO2/MgO[207]. Optimizing the coating composition, microstructure, and thickness can tailor the degradation rate. To illustrate, Kania et al.[209] showed that nano-porous titanate coatings can control the degradation rate of Mg implants.
COMPUTATIONAL MODELS FOR SIMULATION OF TISSUE GROWTH IN Mg-BASED BMG SCAFFOLDS
The integration of Mg-BMGs into biomedical scaffolds presents a promising avenue for enhancing tissue regeneration and implant performance.
Mechano-driven models have successfully predicted variations in bone ingrowth distribution based on scaffold implantation sites, emphasizing the importance of the mechanobiological environment for
Computational models are pivotal in understanding and predicting the complex interactions between
Figure 11. Computer-based methods, such as Finite Element Analysis (FEM)-based Bilinear Isotropic Stress-Strain (BISO) elastoplastic model prediction of scaffolds for tibial bone defect repair. Reproduced with permission from ref.[212], CC BY-NC-ND 4.0 © 2018 Elsevier Ltd.
FEA
FEA is a widely used computational tool in biomedical engineering for assessing the mechanical behavior of implant scaffolds and predicting tissue responses[222,223]. In the context of Mg-BMG scaffolds, FEA models can simulate the distribution of mechanical stresses within the scaffold and surrounding tissues under various loading conditions[224,225]. This is crucial for ensuring the scaffold provides adequate support without causing excessive stress that could impede tissue growth or lead to implant failure. FEA can incorporate the gradual degradation of Mg-BMGs due to corrosion. By modeling the reduction in scaffold mass and structural integrity over time, FEA helps predict how the scaffold’s mechanical properties evolve, thereby influencing tissue regeneration dynamics[226].
Figure 12 compares the stress distribution in bone when using solid versus porous scaffolds [Figure 12A-B], highlighting the benefits of porous scaffolds in reducing stress shielding. The analysis reveals that stress transmission through bone is significantly higher (325 MPa) [Figure 12C-E] with a porous scaffold, while a solid scaffold reduces this to 76.204 MPa [Figure 12D-F], indicating a 76% reduction due to a modulus mismatch with the bone[227]. Porous scaffolds, however, offer a better modulus match, leading to a more even distribution of stress and promoting bone regeneration and stronger implant interfaces, thus reducing stress shielding and implant loosening. The study also notes the importance of considering manufacturing errors and surface roughness in FEA to predict scaffold performance, particularly in patient-specific applications accurately. FEA was used to select the unit cell and indicate the implant’s performance, which was then validated through compression testing with an anatomical stiffness-matched design that may be suitable for segmental bone defect repair, with mitigated stress shielding[212].
Figure 12. FEA of solid and porous scaffolds based on segmental femur defect for BTE and its related stress distributions (units in MPa): (A and B) Implantation of porous and solid scaffolds; (C and D) stress distribution contours on the bone; (E and F) von Mises stress contours of porous and solid scaffolds; (G and H) stress distribution contours for porous and solid scaffolds at 50 MPa. This figure is licensed under CC-BY 4.0 and reproduced from ref.[227] © 1996-2024 MDPI (Basel, Switzerland). FEA: Finite element analysis.
The stress distribution in solid and porous magnesium scaffolds can have different impacts on BTE for segmental femur defects[227]. Solid magnesium scaffolds tend to exhibit higher stress concentrations at the scaffold-bone interface, which can lead to:
(1) Increased risk of scaffold failure, as high-stress concentrations can cause the scaffold to fracture or degrade prematurely, compromising the BTE process[228].
(2) Reduced bone regeneration, since high-stress concentrations can inhibit bone cell growth and differentiation, resulting in impaired bone regeneration and reduced integration with the scaffold[228,229].
On the other hand, porous magnesium scaffolds tend to exhibit lower stress concentrations and more uniform stress distributions, which can lead to improved scaffold stability with lower stress concentrations that can reduce the risk of scaffold failure and improve its stability, allowing for more effective BTE[230,231]. They also enhance bone regeneration where the porous structure can provide a more favorable environment for bone cell growth and differentiation, leading to improved bone regeneration and integration with the scaffold[230,232]. However, the optimal porosity and pore size of the scaffold can vary depending on the specific BTE application and the desired mechanical and biological properties
Advanced FEA models integrate multiple physical phenomena, such as mechanical loading, chemical corrosion, and biological tissue growth[234-236]. These coupled simulations provide a more comprehensive understanding of the interplay between scaffold degradation and tissue development-a study by Liu et al.[237] utilized FEA to model the mechanical stability of Mg-based scaffolds during degradation. The model incorporated corrosion rates and assessed how the loss of material affected stress distribution, providing insights into scaffold design parameters that optimize mechanical support while facilitating tissue ingrowth.
Figure 13 illustrates a biomechanical analysis of a femur via FEA to study bone properties and structural behavior. The left panel depicts the Bone Mineral Density (BMD) distribution of a bone, with a color gradient indicating varying density levels crucial for simulating mechanical strength and stress distribution. The right panel shows a 3D femur model with specific regions marked, likely for evaluating stress concentration, fracture risk, or load response under physiological conditions. Such analyses are vital in orthopedic research, implant design, and understanding bone adaptation to mechanical loads[238].
Figure 13. Representation of the bone mineral density distribution within the bone (left) and location of the string gauges at the femur specimen (right). This figure is licensed under CC-BY 4.0 Reproduced from ref.[238] © 2015 Liebl Hans.
Biomechanical performance design method of joint prosthesis for medical rehabilitation via Generative Structure Optimization (GSO). The method involves 3D reconstruction of hard bone and cartilage structures from heterogeneous medical images such as computed tomography (CT) and magnetic resonance imaging (MRI), followed by FEA to verify the reconstructed structure and multi-objective optimization to design the 3D printing parameters, including adaptive layer thickness, infill patterns, and infill trajectories[239]. The GSO approach covers various stages from personalized CT imaging to 3D reconstruction, finite element analysis, and structural parameter optimization. The physical experiment of additive manufacturing shows that the proposed method can improve the relative density, surface topography, and wear-resistance performance of the joint prosthesis, thereby enhancing its biomechanical performance in terms of kinematics and dynamics[239]. This method aims to promote high-end customization and improve the well-being of patients requiring prosthetic rehabilitation.
ABM
ABM simulates the interactions between cells, such as osteoblasts and osteoclasts, and the scaffold environment in BTE. It models the behavior of osteoblasts, osteoclasts, and other relevant cell types in response to the scaffold environment, including factors such as Mg2+ concentration, pH changes, and mechanical cues[240-242]. ABM allows for the simulation of spatial distributions and temporal evolution of cell populations around the implant, which is essential for understanding how cells migrate, adhere, and form new tissue structures in response to scaffold degradation[240,241]. A study employed ABM to investigate how varying Mg2+ concentrations from degrading Mg-BMG scaffolds affect osteoblast proliferation and bone matrix deposition, providing predictions on optimal degradation rates that balance scaffold support with favorable cellular responses[240]. ABM has also been used to study the complex interactions between different cell types and chemical components in the context of bone regeneration and mechanobiology[242-246]. Computational modeling and simulation of porous scaffolds, including Mg-based trabecular bone implants, is an important tool in BTE applications to restore and treat bone defects[247]. ABM is a powerful computational approach for simulating the complex interactions between cells and the scaffold environment in BTE, allowing researchers to predict optimal scaffold properties and cellular responses.
CFD
CFD is used to model the transport of fluids and solutes around and within the scaffold. CFD models can simulate the release of Mg2+ from the scaffold and their subsequent diffusion through surrounding tissues[248,249]. Understanding the concentration gradients of Mg2+ is crucial, as excessive concentrations can lead to cytotoxicity, while optimal levels can enhance bone regeneration. CFD helps in assessing how scaffold degradation influences the transport of nutrients and waste products, which are vital for cell survival and tissue health[250-252]. A recent study by Manescu et al.[253] utilized CFD to model the diffusion of Mg2+ from a degrading Mg-BMG scaffold within a bone defect. The results highlighted regions of potential Mg2+ accumulation and informed scaffold porosity designs that promote even ion distribution, thereby mitigating cytotoxic risks[253].
Multiscale modeling integrates processes occurring at different spatial and temporal scales, from molecular interactions to macroscopic tissue behavior. This approach is particularly beneficial for Mg-BMG scaffolds due to the complex interplay between material degradation and biological responses[254]. Models at the molecular scale can simulate the corrosion mechanisms of Mg-BMGs, including electrochemical reactions and ion release rates[46,255,256]. Cellular scale models focus on how individual cells respond to changes in their microenvironment, such as alterations in pH and ion concentration due to scaffold degradation[257,258]. Tissue and organ scale models assess the overall tissue regeneration, mechanical integration with the host bone, and long-term implant stability[254]. Researchers have developed a multiscale model that linked molecular corrosion processes of Mg-BMGs with cellular responses and tissue-level bone regeneration[259-262]. The model successfully predicted scaffold performance over 12 months, providing valuable insights for
MD simulations
MD simulations are invaluable for understanding the behavior of Mg-BMG scaffolds used in tissue growth applications. These simulations provide atomic-scale insights into key processes such as biodegradation, protein adsorption, and ion release[89,132,263]. MD models scaffold degradation in physiological environments, revealing the mechanisms of corrosion, ion diffusion, and by-product formation [e.g., Mg(OH)2], which influence local pH and tissue compatibility[89,177]. They also simulate protein-surface interactions, helping optimize scaffold surfaces for better cell adhesion, while tracking ion release to predict its role in promoting osteogenesis and bone mineralization[89].
Additionally, MD predicts the mechanical integrity of scaffolds under physiological loads, ensuring their structural stability during tissue regeneration[264,265]. Immersion and electrochemical tests validate corrosion models, while surface characterization techniques such as AFM confirm protein adsorption prediction[135,177]. Compression and tensile tests ensure the accuracy of mechanical property simulations, and in vitro cell culture studies validate biological responses such as ion concentration effects on osteoblast activity[88,135]. Although MD is limited by scale and biological complexity, its integration with other methods such as finite element analysis and machine learning (ML) offers a path toward comprehensive scaffold design and optimization for biomedical applications[266].
DFT
DFT has been extensively used to study the electronic structure and atomic-scale properties of Mg-based metallic glass (BMG) scaffolds for tissue engineering applications. It provides critical insights into the biodegradation mechanisms, protein adsorption, ion release, and mechanical properties of these scaffolds, which are crucial for their performance in tissue engineering. Specifically, DFT models the surface interactions of Mg-BMG scaffolds with water and ions, allowing the prediction of corrosion rates and the effects of alloying elements such as Zn, Ca, and Y on corrosion resistance[267,268]. DFT also examines how proteins or amino acids bind to the scaffold surfaces, aiding in the optimization of biocompatibility[269,270]. Additionally, it simulates the dissolution of Mg2+, which is critical for promoting osteogenesis and calculates the elastic constants of the scaffolds to predict their stiffness and load-bearing capacity[268,271]. The validation of DFT predictions is achieved through comparisons with experimental data, and the integration of DFT with larger-scale models, such as MD or finite element analysis, can provide multiscale insights[269,270]. Moving forward, the combination of DFT with ML and functionalized surface simulations will further enhance its potential in optimizing Mg-BMG scaffolds for tissue engineering applications[270].
Phase field modeling
Phase field modeling (PFM) is a powerful computational technique that can simulate the evolution of microstructures in Mg-MGs during various processes such as solidification, crystallization, and corrosion[272]. This approach is particularly valuable for investigating the glass-to-crystal transition, which affects the stability and degradation of metallic glasses in biological environments[132,263]. By simulating these microstructural changes, phase field modeling provides a deeper understanding of how Mg-MGs behave under various conditions relevant to their biomedical applications[132,263]. For instance, the model can determine the conditions that minimize crystallization and maintain the amorphous structure, which is critical for ensuring mechanical stability and corrosion resistance in biological environments[90,273]. The predictions from phase field modelling are validated through experimental techniques such as SEM and
Corrosion simulations
The corrosion behavior of Mg-MGs is a key aspect when considering their use in biomedical applications, especially for bone scaffolds that are designed to degrade over time. Computational methods such as MD, DFT, and electrochemical simulations are used to predict how Mg-MGs will behave in physiological environments[38,263,274]. To illustrate, electrochemical simulations can predict the corrosion rate of Mg-MGs in SBFs, considering factors such as pH, ion concentration, and temperature[267,274,275]. These computational predictions can then be validated by comparing them with experimental results obtained from immersion tests or electrochemical measurements (e.g., potentiodynamic polarization tests or electrochemical impedance spectroscopy) to assess the accuracy of the computational models[258,274,276].
Mg-MGs generally exhibit better corrosion resistance compared to their crystalline counterparts, due to their amorphous structure[263,276]. This improved corrosion resistance is a key advantage for their use in biomedical applications, as it can help ensure the mechanical stability and degradation behavior of
ML and artificial intelligence
The integration of ML and artificial intelligence (AI) in computational modeling offers enhanced predictive capabilities and optimization of scaffold designs[277,278]. ML algorithms can analyze large datasets from experimental and simulation studies to identify patterns and predict outcomes related to tissue growth and scaffold performance. AI-driven optimization techniques can determine the optimal combination of scaffold properties (e.g., porosity and alloy composition) that maximize tissue regeneration while minimizing adverse effects[279,280]. Recent studies applied machine-learning models to predict bone regeneration outcomes based on various scaffold design parameters and degradation rates[281,282]. The model facilitated the identification of design strategies that enhance tissue integration and mechanical stability.
Figure 14 summarizes a framework of a Machine Learning Regression model that can predict the performance of nanofibrous scaffolds for skin tissue engineering.
Figure 14. Development of Machine Learning Regression Models to understand the problem and the data to select the most appropriate analytic technique for predicting performance. The figure is licensed under CC-BY 4.0. Reproduced from ref.[283] © Copyright (2024) The Institution of Engineering and Technology.
The framework comprises two key stages: Model Preparation and Model Deployment. In the preparation stage, transformer loading estimation involves data preprocessing, ML model fitting, and evaluation to identify the most relevant smart meters (SMs) and the optimal regression algorithm[283]. Regression techniques, including random forest, decision tree, AdaBoost, and gradient boosting, can be applied to datasets featuring four numerical parameters-water contact angle, fiber diameter, Young's modulus, and pore diameter-to predict cell number[278]. In the deployment stage, data from the selected SMs is input into the trained and tuned model to estimate transformer loading and identify congestion, with performance results[283]. Among the techniques, AdaBoost has been reported to demonstrate the highest accuracy, with a mean absolute percentage error of 1.41% and a coefficient of determination (R2) of 0.999, indicating a strong correlation between predicted and actual values[278]. These results offer a valuable tool for estimating cell generation based on scaffold physicochemical properties, aiding physicians in the design and optimization of scaffolds for skin tissue engineering.
ML can help identify new alloy compositions with optimized mechanical properties and biodegradation rates for use in biomedical scaffolds. ML can be used to analyze the relationship between alloy composition, processing conditions, and the mechanical and degradation properties of Mg-MGs[283]. Computational models have been used to design new Mg-based MG compositions, such as Mg-Zn-Ca and Mg-Zn-Ag, predicting their amorphous forming ability, mechanical properties, and corrosion behavior[284-286]. These models, combined with experimental validation through techniques such as magnetron sputtering and additive manufacturing, accelerate the development of Mg-based MGs for biomedical scaffolds by optimizing their properties for biocompatibility, biodegradability, and mechanical performance[284,285].
Limitations of existing computational models
Key limitations of existing computational models for simulating tissue growth in Mg-BMG scaffolds are:
• Limitations in accurately simulating the complex interactions between different tissue types (e.g., cartilage and bone) and the Mg-based biomaterial scaffold[240].
• Challenges in accurately modeling the parameters involved in tissue healing and cell seeding procedures in perfusion bioreactors to achieve optimal bone tissue growth[287].
• Difficulties in accurately predicting the in vivo service life and degradation rate of Mg-based biomedical devices, which should match the rate of bone/tissue growth[236].
• Limitations in accurately modeling the antibacterial and cell response properties of Mg-based scaffolds coated with materials such as alginate and magnesium oxide[288].
• Challenges in computationally modeling the 3D printing and fabrication processes of Mg-based metallic glass scaffolds, including issues related to fluid dynamics and structural integrity[68,289].
• Limitations in fully recreating the tissue heterogeneity and accurately reflecting human pharmacokinetics in computational models[290].
• Difficulties in computationally modeling the disordered structure and reduced mechanical properties of metallic glasses, which can lead to potential tissue damage[291].
• Exploring computational approaches to model the disordered structure and mechanical properties of metallic glasses to minimize the risk of tissue damage[291].
FUTURE DIRECTIONS AND CHALLENGES TO PROMOTE Mg-BASED METALLIC GLASS SCAFFOLDS
To advance Mg-based metallic glass scaffolds in biomedical applications, future focus should entail optimization of porosity, pore structure, and interconnectivity for better cell infiltration and tissue growth. Incorporating bioactive agents such as growth factors enhances tissue regenerative properties and evaluates long-term performance and integration with host tissues. For wound healing, developing scaffolds or coatings with antimicrobial properties and exploring the combined effects of Mg2+ released are practical future challenges. In cardiovascular applications, it is critical to design scaffolds with improved mechanical properties and biocompatibility for long-term performance. The overall challenges in biomedical scaffolds include balancing mechanical properties, biodegradability, biocompatibility, and manufacturability addressing potential cytotoxicity and materials stability. Continued research is essential to address these challenges and fully exploit the potential of Mg-based metallic glass scaffolds.
Scaling up the production of TFMG-coated implants from laboratory to commercial scale remains challenging. Substrate effects and contamination can be a problem in the production process of TFMG-coated implants[292,293]. Optimized and improved preparation processes, matrix systems, and structural modelings should be developed to address the challenges in commercialized TFMG production. More long-term studies are needed to fully understand the performance and potential side effects of Mg-based TFMG coatings in clinical settings[294]. Accessing and testing the biocompatibility of TFMG coatings in vivo remains a challenge. Advancements in computational methodologies and interdisciplinary collaboration are poised to overcome existing challenges and enhance the utility of models in Mg-BMG scaffold development. Incorporating genomic, proteomic, and metabolomic data can provide deeper insights into cellular responses and improve model accuracy. Developing models that can update in real time with experimental data will enable dynamic simulations that better reflect ongoing biological processes. Refining multiscale models to seamlessly integrate molecular, cellular, and tissue-level processes will provide more holistic predictions of scaffold performance. Leveraging big data and advanced AI techniques can enhance model training, reduce uncertainty, and enable the discovery of novel scaffold design principles. Tailoring scaffold designs based on patient-specific data through computational models can lead to personalized implants that optimize tissue regeneration and implant integration.
Successful translation of Mg-based scaffold implants to clinical practice will require continued research to address and control corrosion, biocompatibility and biodegradation. Surface modification of Mg-MGs as discussed here is promising and needs more input such as bioactive surface modification of the material for efficient biomedical applications. The economic viability of surface modification of Mg-based scaffolds is critical as the high cost of TFMG materials and deposition techniques will deter the clinical translation of these scaffolds; thus, optimized composition and process are paramount to make them commercially viable products for widespread use.
While computational models offer significant advantages in understanding and optimizing Mg-BMG scaffolds, several challenges persist. Accurately capturing the multifaceted interactions between scaffold degradation, ion release, and biological responses remains a formidable task[295]. Simplifications and assumptions in models can limit their predictive accuracy, and many model parameters, such as corrosion rates and cellular response thresholds, are derived from experimental data that may exhibit variability, which can affect the reliability of model predictions[295]. This uncertainty can affect the reliability of model predictions. High-fidelity models, especially multiscale and coupled simulations, demand substantial computational power and time, which can be a barrier to their widespread application. Combining data from various sources (e.g., mechanical testing, biological assays, imaging) into cohesive models is challenging but necessary for comprehensive simulations[296,297].
Moreover, potential solutions to address the limitations of existing computational models include:
• Developing more sophisticated multiscale models that can capture the complex interactions between different tissue types and the Mg-based scaffold.
• Improving computational models to better simulate the parameters involved in tissue healing and cell seeding in bioreactors.
• Enhancing computational models to accurately predict the in vivo degradation and absorption of
• Incorporating advanced computational techniques, such as finite element analysis and computational fluid dynamics, to model the 3D printing and fabrication processes of Mg-based metallic glass scaffolds.
• Advancing computational models to better reflect tissue heterogeneity and human pharmacokinetics.
CONCLUSION
The development of Mg-based metallic glass scaffolds represents a promising frontier in biomedical applications, offering significant advancements in tissue engineering, wound healing, and cardiovascular implants. By optimizing scaffold characteristics such as pore structure, porosity, and interconnectivity, and incorporating bioactive agents, researchers can enhance cell infiltration, proliferation, and tissue regeneration. The Mg2+ release with other therapeutic agents can further improve wound healing outcomes. For cardiovascular applications, the focus on improving mechanical properties, corrosion resistance, and biocompatibility, alongside evaluating long-term in vivo performance, is crucial. However, challenges remain, including balancing mechanical strength with degradation rates, developing scalable manufacturing techniques, ensuring long-term stability, and addressing potential cytotoxicity. Addressing these challenges through surface modification with TFMGs and continued research and innovation will be essential to realizing the full potential of Mg-based metallic glass scaffolds and advancing their application in various medical fields. Moreover, computational models provide a powerful tool for simulating tissue growth around Mg-based implant scaffolds. By combining different approaches such as finite element analysis for mechanical interactions, agent-based models for cellular dynamics, and diffusion-reaction models for nutrient supply researchers can gain valuable insights into the behavior of these biodegradable materials in vivo. These models enhance our understanding of how Mg-based scaffolds perform and offer the potential to optimize scaffold design and predict long-term outcomes, ultimately improving their application in regenerative medicine and permanent implants.
Future research on Mg-MGs (Mg-BMGs) for biomedical scaffolds should focus on enhancing glass-forming ability, optimizing biodegradability and corrosion control, and tailoring mechanical properties to match natural bone. Advanced computational modeling (e.g., MD, DFT, and FEA) can accelerate alloy design and predict degradation behavior, while bioactive surface modifications and 3D printing can improve biocompatibility and scaffold customization. Long-term in-vivo studies are needed to evaluate clinical performance, and sustainable production methods can facilitate cost-effective manufacturing. These advancements aim to establish Mg-BMGs as a leading material for biodegradable, bioactive scaffolds in regenerative medicine.
Moreover, their ability to degrade naturally aligns with tissue healing, reducing the need for surgical removal. Realizing these applications requires interdisciplinary collaboration among materials scientists, engineers, computational modelers, and clinicians to optimize alloy properties, predict performance, and design effective bioactive surfaces. Such collaboration is key to advancing Mg-BMGs as a revolutionary material in regenerative medicine.
Fabrication techniques must overcome the low glass-forming ability of Mg alloys to produce large, defect-free amorphous structures suitable for medical applications. Cost control remains a hurdle, as current manufacturing processes and alloying elements can be expensive, limiting scalability. Additionally, ensuring long-term biocompatibility is crucial, requiring detailed studies to address potential cytotoxicity, inflammatory responses, and the controlled release of degradation products. Tackling these challenges demands innovation in processing methods, alloy design, and interdisciplinary collaboration to unlock the full potential of Mg-BMGs in biomedical applications.
DECLARATIONS
Authors’ contributions
Manuscript writing, data analysis, methodology, investigation, formal analysis, visualization, data curation, and conceptualization: Onyeagba, C. R.
Validation, methodology, funding acquisition, conceptualization. Manuscript review and editing: Tesfamichael, T.
Availability of data and materials
Not applicable.
Financial support and sponsorship
This work pertains to the Faculty Write-Up (FWU) scholarship program of the Queensland University of Technology. Dr. Onyeagba, C. R. acknowledges the scholarship received from the Faculty of Engineering, School of Mechanical, Medical and Process Engineering (MMPE), Centre for Biomedical Technology and Centre for Materials Science in Brisbane, Australia.
Conflicts of interest
All authors declared that there are no conflicts of interest.
Ethical approval and consent to participate
Not applicable.
Consent for publication
Not applicable.
Copyright
© The Author(s) 2025.
REFERENCES
1. Baino, F.; Novajra, G.; Vitale-Brovarone, C. Bioceramics and scaffolds: a winning combination for tissue engineering. Front. Bioeng. Biotechnol. 2015, 3, 202.
2. Arifvianto, B.; Zhou, J. Fabrication of metallic biomedical scaffolds with the space holder method: a review. Materials 2014, 7, 3588-622.
3. Boccaccini, A. R.; Ma, P. X.; Liverani, L. Tissue engineering using ceramics and polymers. 3th ed. Woodhead Publishing; 2021. p. 888. Available from: https://books.google.com/books?hl=zh-CN&lr=&id=T48mEAAAQBAJ&oi=fnd&pg=PP1&dq=.+Boccaccini+AR,+Ma+PX,+Liverani+L.+Tissue+engineering+using+ceramics+and+polymers.+3th+ed.+Woodhead+Publishing%3B+2021.+p.+888.&ots=dFsHECuJGQ&sig=SJ5feosbK0lDHAPtYiXHwzW35cE#v=onepage&q&f=false. [Last accessed on 6 Mar 2025].
4. Suamte, L.; Tirkey, A.; Barman, J.; Jayasekhar, B. P. Various manufacturing methods and ideal properties of scaffolds for tissue engineering applications. Smart. Mater. Manuf. 2023, 1, 100011.
5. Ahmadipour, M.; Mohammadi, H.; Pang, A. L.; et al. A review: silicate ceramic-polymer composite scaffold for bone tissue engineering. Int. J. Polym. Mater. Polym. Biomater. 2022, 71, 180-95.
6. Thangavel, M.; Elsen, S. R. Review of physical, mechanical, and biological characteristics of 3D-printed bioceramic scaffolds for bone tissue engineering applications. ACS. Biomater. Sci. Eng. 2022, 8, 5060-93.
7. Nuss, K. M.; von, R. B. Biocompatibility issues with modern implants in bone-a review for clinical orthopedics. Open. Orthop. J. 2008, 2, 66-78.
8. Söhling, N.; Ondreka, M.; Kontradowitz, K.; Reichel, T.; Marzi, I.; Henrich, D. Early immune response in foreign body reaction is implant/material specific. Materials 2022, 15, 2195.
9. Kämmerling, L.; Fisher, L. E.; Antmen, E.; et al. Mitigating the foreign body response through ‘immune-instructive’ biomaterials. J. Immunol. Regen. Med. 2021, 12, 100040.
10. Zhang, Y.; Xu, J.; Ruan, Y. C.; et al. Implant-derived magnesium induces local neuronal production of CGRP to improve bone-fracture healing in rats. Nat. Med. 2016, 22, 1160-9.
11. Shanmugavadivu, A.; Lekhavadhani, S.; Babu, S.; Suresh, N.; Selvamurugan, N. magnesium-incorporated biocomposite scaffolds: a novel frontier in bone tissue engineering. J. Magnes. Alloys. 2024, 12, 2231-48.
12. Hung, C. C.; Chaya, A.; Liu, K.; Verdelis, K.; Sfeir, C. The role of magnesium ions in bone regeneration involves the canonical Wnt signaling pathway. Acta. Biomater. 2019, 98, 246-55.
13. Ye, L.; Xu, J.; Mi, J.; et al. Biodegradable magnesium combined with distraction osteogenesis synergistically stimulates bone tissue regeneration via CGRP-FAK-VEGF signaling axis. Biomaterials 2021, 275, 120984.
14. Wang, Q.; Qin, H.; Deng, J.; et al. Research progress in calcitonin gene-related peptide and bone repair. Biomolecules 2023, 13, 838.
15. Zaidi, M.; Moonga, B. S.; Abe, E. Calcitonin and bone formation: a knockout full of surprises. J. Clin. Invest. 2002, 110, 1769-71.
16. Müller, E.; Schoberwalter, T.; Mader, K.; et al. The biological effects of magnesium-based implants on the skeleton and their clinical implications in orthopedic trauma surgery. Biomater. Res. 2024, 28, 0122.
17. Han, F.; Wang, J.; Ding, L.; et al. Tissue engineering and regenerative medicine: achievements, future, and sustainability in asia. Front. Bioeng. Biotechnol. 2020, 8, 83.
18. Miki, K.; Takeshita, N.; Yamashita, M.; Kitamura, M.; Murakami, S. Calcitonin gene-related peptide regulates periodontal tissue regeneration. Sci. Rep. 2024, 14, 1344.
19. Wimalawansa, S. J. Calcitonin gene-related peptide and its receptors: molecular genetics, physiology, pathophysiology, and therapeutic potentials. Endocr. Rev. 1996, 17, 533-85.
20. Wu, H.; Lin, X. Q.; Long, Y.; Wang, J. Calcitonin gene-related peptide is potential therapeutic target of osteoporosis. Heliyon 2022, 8, e12288.
21. Yeung, K. W. K.; Wong, K. H. M. Biodegradable metallic materials for orthopaedic implantations: a review. Technol. Health. Care. 2012, 20, 345-62.
22. Qin, Y.; Wen, P.; Guo, H.; et al. Additive manufacturing of biodegradable metals: current research status and future perspectives. Acta. Biomater. 2019, 98, 3-22.
23. Yazdimamaghani, M.; Razavi, M.; Vashaee, D.; Moharamzadeh, K.; Boccaccini, A. R.; Tayebi, L. Porous magnesium-based scaffolds for tissue engineering. Mater. Sci. Eng. C. 2017, 71, 1253-66.
24. Levy, G. K.; Goldman, J.; Aghion, E. The prospects of zinc as a structural material for biodegradable implants-a review paper. Metals 2017, 7, 402.
25. Amukarimi, S.; Mozafari, M. Biodegradable magnesium-based biomaterials: an overview of challenges and opportunities. MedComm 2021, 2, 123-44.
26. Shahzamanian, M.; Banerjee, R.; Dahotre, N. B.; Srinivasa, A. R.; Reddy, J. Analysis of stress shielding reduction in bone fracture fixation implant using functionally graded materials. Compos. Struct. 2023, 321, 117262.
27. Li, K.; Liang, L.; Du, P.; et al. Mechanical properties and corrosion resistance of powder metallurgical Mg-Zn-Ca/Fe bulk metal glass composites for biomedical application. J. Mater. Scie. Technol. 2022, 103, 73-83.
28. Velikokhatnyi, O. I.; Kumta, P. N. First principles study of the elastic properties of magnesium and iron based bio-resorbable alloys. Mater. Sci. Eng. B. 2018, 230, 20-3.
29. Cheng, J.; Liu, B.; Wu, Y.; Zheng, Y. Comparative in vitro study on pure metals (Fe, Mn, Mg, Zn and W) as biodegradable metals. J. Mater. Sci. Technol. 2013, 29, 619-27.
30. Witte, F. The history of biodegradable magnesium implants: a review. Acta. Biomater. 2010, 6, 1680-92.
31. Waizy, H.; Seitz, J.; Reifenrath, J.; et al. Biodegradable magnesium implants for orthopedic applications. J. Mater. Sci. 2013, 48, 39-50.
32. Meagher, P.; O’Cearbhaill, E. D.; Byrne, J. H.; Browne, D. J. Bulk metallic glasses for implantable medical devices and surgical tools. Adv. Mater. 2016, 28, 5755-62.
33. Sezer, N.; Evis, Z.; Koç, M. Additive manufacturing of biodegradable magnesium implants and scaffolds: review of the recent advances and research trends. J. Magnes. Alloys. 2021, 9, 392-415.
34. Staiger, M. P.; Pietak, A. M.; Huadmai, J.; Dias, G. Magnesium and its alloys as orthopedic biomaterials: a review. Biomaterials 2006, 27, 1728-34.
36. Cheng, Y.; Ma, E. Atomic-level structure and structure-property relationship in metallic glasses. Prog. Mater. Sci. 2011, 56, 379-473.
37. Xie, G.; Wang, X. Metallic glasses for biomedical applications. In: Setsuhara Y, Kamiya T, Yamaura S, editors. Novel structured metallic and inorganic materials. Singapore: Springer; 2019. pp. 421-33.
38. Biały, M.; Hasiak, M.; Łaszcz, A. Review on biocompatibility and prospect biomedical applications of novel functional metallic glasses. J. Funct. Biomater. 2022, 13, 245.
39. Du, P.; Wu, Z.; Li, K.; Xiang, T.; Xie, G. Porous Ti-based bulk metallic glass orthopedic biomaterial with high strength and low Young’s modulus produced by one step SPS. J. Mater. Res. Technol. 2021, 13, 251-9.
40. Demetriou, M. D.; Wiest, A.; Hofmann, D. C.; et al. Amorphous metals for hard-tissue prosthesis. JOM. 2010, 62, 83-91.
41. Inoue, A. Stabilization of metallic supercooled liquid and bulk amorphous alloys. Acta. Materialia. 2000, 48, 279-306.
43. Sharma, A.; Zadorozhnyy, V. Review of the recent development in metallic glass and its composites. Metals 2021, 11, 1933.
44. Onyeagba, C.; Valashani, M.; Wang, H.; Brown, C.; Yarlagadda, P.; Tesfamichael, T. Nanomechanical surface properties of co-sputtered thin film polymorphic metallic glasses based on Ti-Fe-Cu, Zr-Fe-Al, and Zr-W-Cu. Surf. Interfaces. 2023, 40, 103090.
45. Onyeagba, C.; Will, G.; Barclay, M.; Brown, C.; Wang, H.; Tesfamichael, T. Polymorphous nanostructured metallic glass coatings for corrosion protection of medical grade Ti substrate. Intermetallics 2024, 165, 108167.
46. Li, H. F.; Zheng, Y. F. Recent advances in bulk metallic glasses for biomedical applications. Acta. Biomater. 2016, 36, 1-20.
47. Li, Z.; Huang, Z.; Sun, F.; Li, X.; Ma, J. Forming of metallic glasses: mechanisms and processes. Mater. Today. Adv. 2020, 7, 100077.
48. Dambatta, M.; Izman, S.; Yahaya, B.; Lim, J.; Kurniawan, D. Mg-based bulk metallic glasses for biodegradable implant materials: a review on glass forming ability, mechanical properties, and biocompatibility. J. Non-Cryst. Solids. 2015, 426, 110-5.
49. Bejarano, J.; Boccaccini, A. R.; Covarrubias, C.; Palza, H. Effect of Cu- and Zn-doped bioactive glasses on the in vitro bioactivity, mechanical and degradation behavior of biodegradable PDLLA scaffolds. Materials 2020, 13, 2908.
50. Purnama, A.; Hermawan, H.; Couet, J.; Mantovani, D. Assessing the biocompatibility of degradable metallic materials: state-of-the-art and focus on the potential of genetic regulation. Acta. Biomater. 2010, 6, 1800-7.
51. Williams, E.; Lavery, N. Laser processing of bulk metallic glass: a review. J. Mater. Process. Technol. 2017, 247, 73-91.
52. Wang, W.; Dong, C.; Shek, C. Bulk metallic glasses. Mater. Sci. Eng. R:. Reports. 2004, 44, 45-89.
53. Sohrabi, N.; Jhabvala, J.; Logé, R. E. Additive manufacturing of bulk metallic glasses-process, challenges and properties: a review. Metals 2021, 11, 1279.
54. Klement, W.; Willens, R. H.; Duwez, P. Non-crystalline structure in solidified gold-silicon alloys. Nature 1960, 187, 869-70.
56. Pan, C.; Wu, T.; Chen, M.; Chang, Y.; Lee, C.; Huang, J. Hot embossing of micro-lens array on bulk metallic glass. Sens. Actuators. A. Phys. 2008, 141, 422-31.
57. Kawamura, Y.; Shibata, T.; Inoue, A.; Masumoto, T. Workability of the supercooled liquid in the Zr65Al10Ni10Cu15 bulk metallic glass. Acta. Mater. 1998, 46, 253-63.
58. Martinez, R.; Kumar, G.; Schroers, J. Hot rolling of bulk metallic glass in its supercooled liquid region. Scripta. Mater. 2008, 59, 187-90.
59. Wiest, A.; Harmon, J.; Demetriou, M.; Daleconner, R.; Johnson, W. Injection molding metallic glass. Scripta. Mater. 2009, 60, 160-3.
60. Schroers, J.; Hodges, T. M.; Kumar, G.; et al. Thermoplastic blow molding of metals. Materials. Today. 2011, 14, 14-9.
62. Li, X.; Li, G.; Ma, J.; Cao, Y.; Xu, Y.; Ming, W. Progress in the preparation, forming and machining of metallic glasses. J. Manuf. Processes. 2024, 117, 244-77.
63. Khan, M. M.; Nemati, A.; Rahman, Z. U.; Shah, U. H.; Asgar, H.; Haider, W. Recent advancements in bulk metallic glasses and their applications: a review. Crit. Rev. Solid. State. Mater. Sci. 2018, 43, 233-68.
65. Li, H. X.; Lu, Z. C.; Wang, S. L.; Wu, Y.; Lu, Z. P. Fe-based bulk metallic glasses: glass formation, fabrication, properties and applications. Progress. Mater. Sci. 2019, 103, 235-18.
67. Jung, H. Y.; Choi, S. J.; Prashanth, K. G.; et al. Fabrication of Fe-based bulk metallic glass by selective laser melting: a parameter study. Mater. Des. 2015, 86, 703-8.
68. Zhang, C.; Ouyang, D.; Pauly, S.; Liu, L. 3D printing of bulk metallic glasses. Mater. Sci. Eng. R:. Reports. 2021, 145, 100625.
69. Kumar, G.; Desai, A.; Schroers, J. Bulk metallic glass: the smaller the better. Adv. Mater. 2011, 23, 461-76.
70. Zhang, C.; Li, X.; Liu, S.; Liu, H.; Yu, L.; Liu, L. 3D printing of Zr-based bulk metallic glasses and components for potential biomedical applications. J. Alloys. Compds. 2019, 790, 963-73.
71. Ouyang, D.; Zhang, P.; Zhang, C.; Liu, L. Understanding of crystallization behaviors in laser 3D printing of bulk metallic glasses. Appl. Mater. Today. 2021, 23, 100988.
72. Xie, F.; Chen, Q.; Gao, J.; Li, Y. Laser 3D printing of Fe-based bulk metallic glass: microstructure evolution and crack propagation. J. Mater. Eng. Perform. 2019, 28, 3478-86.
73. Ouyang, D.; Li, N.; Xing, W.; Zhang, J.; Liu, L. 3D printing of crack-free high strength Zr-based bulk metallic glass composite by selective laser melting. Intermetallics 2017, 90, 128-34.
74. Hofmann, D. C. Bulk metallic glasses and their composites: a brief history of diverging fields. J. Mater. 2012, 1, 517904.
76. Pan, D.; Inoue, A.; Sakurai, T.; Chen, M. W. Experimental characterization of shear transformation zones for plastic flow of bulk metallic glasses. Proc. Natl. Acad. Sci. U. S. A. 2008, 105, 14769-72.
77. Hofmann, D. C.; Suh, J. Y.; Wiest, A.; et al. Designing metallic glass matrix composites with high toughness and tensile ductility. Nature 2008, 451, 1085-9.
78. Xu, J.; Ramamurty, U.; Ma, E. The fracture toughness of bulk metallic glasses. JOM. 2010, 62, 10-8.
79. He, Q.; Cheng, Y.; Ma, E.; Xu, J. Locating bulk metallic glasses with high fracture toughness: chemical effects and composition optimization. Acta. Mater. 2011, 59, 202-15.
80. Nekouie, V.; Abeygunawardane-arachchige, G.; Roy, A.; Silberschmidt, V. V. Bulk metallic glasses: mechanical properties and performance. In: Silberschmidt VV, Matveenko VP, editors. Mechanics of advanced materials. Cham: Springer International Publishing; 2015. pp. 101-34.
81. Hu, J.; Shao, J.; Huang, G.; Zhang, J.; Pan, S. In vitro and in vivo applications of magnesium-enriched biomaterials for vascularized osteogenesis in bone tissue engineering: a review of literature. J. Funct. Biomater. 2023, 14, 326.
82. Cheng, M. Q.; Wahafu, T.; Jiang, G. F.; et al. A novel open-porous magnesium scaffold with controllable microstructures and properties for bone regeneration. Sci. Rep. 2016, 6, 24134.
83. Mordike, B.; Ebert, T. Magnesium: properties - applications - potential. Mater. Sci. Eng. A. 2001, 302, 37-45.
84. Tóth, L.; Yarema, S. Y. Formation of the science of fatigue of metals. Part 1. 1825-1870. Mater. Sci. 2006, 42, 673-80.
85. Zhang, X.; Chen, G.; Bauer, T. Mg-based bulk metallic glass composite with high bio-corrosion resistance and excellent mechanical properties. Intermetallics 2012, 29, 56-60.
87. Ductile magnesium, rolling, alloy design, rare earth, yttrium, Erbium, Y, casting, rolling, bending, sheet forming. 2024. Available from: https://www.dierk-raabe.com/magnesium-alloys/. [Last accessed on 6 Mar 2025].
88. Antoniac, I.; Manescu, P. V.; Paltanea, G.; et al. Additive manufactured magnesium-based scaffolds for tissue engineering. Materials 2022, 15, 8693.
89. Chu, Y. S.; Wong, P. C.; Jang, J. S.; Chen, C. H.; Wu, S. H. Combining Mg-Zn-Ca bulk metallic glass with a mesoporous silica nanocomposite for bone tissue engineering. Pharmaceutics 2022, 14, 1078.
90. Jin, C.; Liu, Z.; Yu, W.; Qin, C.; Yu, H.; Wang, Z. Biodegradable Mg-Zn-Ca-based metallic glasses. Materials 2022, 15, 2172.
91. Chen, J.; Dong, J.; Fu, H.; et al. In vitro and in vivo studies on the biodegradable behavior and bone response of Mg69Zn27Ca4 metal glass for treatment of bone defect. J. Mater. Sci. Technol. 2019, 35, 2254-62.
92. Zhao, Y.; Zhao, X. Structural relaxation and its influence on the elastic properties and notch toughness of Mg-Zn-Ca bulk metallic glass. J. Alloys. Compd. 2012, 515, 154-60.
93. Li, H.; Pang, S.; Liu, Y.; Sun, L.; Liaw, P. K.; Zhang, T. Biodegradable Mg-Zn-Ca-Sr bulk metallic glasses with enhanced corrosion performance for biomedical applications. Mater. Des. 2015, 67, 9-19.
94. Gu, X.; Shiflet, G.; Guo, F.; Poon, S. Mg-Ca-Zn bulk metallic glasses with high strength and significant ductility. J. Mater. Res. 2005, 20, 1935-8.
95. Sun, Y.; Zhang, H.; Fu, H.; Wang, A.; Hu, Z. Mg-Cu-Ag-Er bulk metallic glasses with high glass forming ability and compressive strength. Mater. Sci. Eng. A. 2009, 502, 148-52.
96. Amiya, K.; Inoue, A. Preparation of bulk glassy Mg65Y10Cu15Ag5Pd5 alloy of 12 mm in diameter by water ouenching. Mater. Trans. 2001, 42, 543-5.
97. Li, Y.; Liu, H. Y.; Jones, H. Easy glass formation in magnesium-based Mg-Ni-Nd alloys. J. Mater. Sci. 1996, 31, 1857-63.
98. Park, E.; Kim, D. Formation of Mg-Cu-Ni-Ag-Zn-Y-Gd bulk glassy alloy by casting into cone-shaped copper mold in air atmosphere. J. Mater. Res. 2005, 20, 1465-9.
99. Yuan, G.; Qin, C.; Inoue, A. Mg-based bulk glassy alloys with high strength above 900 MPa and plastic strain. J. Mater. Res. 2005, 20, 394-400.
100. Gulenko, A.; Forto, C. L.; Gao, J.; et al. Atomic structure of Mg-based metallic glasses from molecular dynamics and neutron diffraction. Phys. Chem. Chem. Phys. 2017, 19, 8504-15.
101. Babilas, R.; Łukowiec, D.; Temleitner, L. Atomic structure of Mg-based metallic glass investigated with neutron diffraction, reverse Monte Carlo modeling and electron microscopy. Beilstein. J. Nanotechnol. 2017, 8, 1174-82.
102. Shi, L.; Xu, J. Mg based bulk metallic glasses: Glass transition temperature and elastic properties versus toughness. J. Non-Cryst. Solids. 2011, 357, 2926-33.
103. Wang, S.; Sun, M.; Song, Z.; Xu, J. Cast defects induced sample-size dependency on compressive strength and fracture toughness of Mg-Cu-Ag-Gd bulk metallic glass. Intermetallics 2012, 29, 123-32.
104. Ma, H.; Shi, L.; Xu, J.; Ma, E. Chill-cast in situ composites in the pseudo-ternary Mg-(Cu,Ni)-Y glass-forming system: microstructure and compressive properties. J. Mater. Res. 2006, 22, 314-25.
105. Rahman, M.; Dutta, N. K.; Roy, C. N. Magnesium alloys with tunable interfaces as bone implant materials. Front. Bioeng. Biotechnol. 2020, 8, 564.
106. Xu, H.; Hu, T.; Wang, M.; et al. Degradability and biocompatibility of magnesium-MAO: the consistency and contradiction between in-vitro and in-vivo outcomes. Arab. J. Chem. 2020, 13, 2795-805.
107. Rondanelli, M.; Faliva, M. A.; Tartara, A.; et al. An update on magnesium and bone health. Biometals 2021, 34, 715-36.
108. Herber, V.; Okutan, B.; Antonoglou, G.; G, S. N.; Payer, M. Bioresorbable magnesium-based alloys as novel biomaterials in oral bone regeneration: general review and clinical perspectives. J. Clin. Med. 2021, 10, 1842.
109. Ding, P.; Liu, Y.; He, X.; Liu, D.; Chen, M. In vitro and in vivo biocompatibility of Mg-Zn-Ca alloy operative clip. Bioact. Mater. 2019, 4, 236-44.
110. Uddin, M. S.; Hall, C.; Murphy, P. Surface treatments for controlling corrosion rate of biodegradable Mg and Mg-based alloy implants. Sci. Technol. Adv. Mater. 2015, 16, 053501.
111. Xue, D.; Yun, Y.; Tan, Z.; Dong, Z.; Schulz, M. J. In vivo and in vitro degradation behavior of magnesium alloys as biomaterials. JMater. Sci. Technol. 2012, 28, 261-7.
112. Guo, K. W. A review of magnesium/magnesium alloys corrosion and its protection. Recent. Pat. Corros. Sci. 2020, 2, 13-21.
113. Kannan, M. B.; Raman, R. K. In vitro degradation and mechanical integrity of calcium-containing magnesium alloys in modified-simulated body fluid. Biomaterials 2008, 29, 2306-14.
114. Ng, W.; Chiu, K.; Cheng, F. Effect of pH on the in vitro corrosion rate of magnesium degradable implant material. Mater. Scie. Eng. C. 2010, 30, 898-903.
115. Törne, K.; Örnberg, A.; Weissenrieder, J. The influence of buffer system and biological fluids on the degradation of magnesium. J. Biomed. Mater. Res. B. Appl. Biomater. 2017, 105, 1490-502.
116. Mueller, W. D.; de, M. M. F.; Nascimento, M. L.; Zeddies, M. Degradation of magnesium and its alloys: dependence on the composition of the synthetic biological media. J. Biomed. Mater. Res. A. 2009, 90, 487-95.
117. Kirkland, N. T.; Birbilis, N.; Staiger, M. P. Assessing the corrosion of biodegradable magnesium implants: a critical review of current methodologies and their limitations. Acta. Biomater. 2012, 8, 925-36.
118. Kumar, S.; Katyal, P.; Chaudhary, R.; Singh, V. Assessment of factors influencing bio-corrosion of magnesium based alloy implants: a review. Mater. Today:. Proc. 2022, 56, 2680-9.
119. Kamrani, S.; Fleck, C. Biodegradable magnesium alloys as temporary orthopaedic implants: a review. Biometals 2019, 32, 185-93.
120. Liu, C.; Xin, Y.; Tian, X.; Chu, P. K. Degradation susceptibility of surgical magnesium alloy in artificial biological fluid containing albumin. J. Mater. Res. 2007, 22, 1806-14.
121. Li, X.; Liu, X.; Wu, S.; Yeung, K. W. K.; Zheng, Y.; Chu, P. K. Design of magnesium alloys with controllable degradation for biomedical implants: from bulk to surface. Acta. Biomater. 2016, 45, 2-30.
122. Xin, Y.; Liu, C.; Zhang, X.; Tang, G.; Tian, X.; Chu, P. K. Corrosion behavior of biomedical AZ91 magnesium alloy in simulated body fluids. J. Mater. Res. 2007, 22, 2004-11.
123. Alawi AM, Majoni SW, Falhammar H. Magnesium and human health: perspectives and research directions. Int. J. Endocrinol. 2018, 2018, 9041694.
124. Gums, J. G. Magnesium in cardiovascular and other disorders. Am. J. Health. Syst. Pharm. 2004, 61, 1569-76.
125. Gröber, U.; Schmidt, J.; Kisters, K. Magnesium in prevention and therapy. Nutrients 2015, 7, 8199-226.
127. Zhu, S.; Wu, C.; Li, G.; Zheng, Y.; Nie, J. Creep properties of biodegradable Zn-0.1Li alloy at human body temperature: implications for its durability as stents. Mater. Res. Lett. 2019, 7, 347-53.
128. Li, H.; Yang, H.; Zheng, Y.; Zhou, F.; Qiu, K.; Wang, X. Design and characterizations of novel biodegradable ternary Zn-based alloys with IIA nutrient alloying elements Mg, Ca and Sr. Mater. Des. 2015, 83, 95-102.
129. Bowen, P. K.; Guillory, R. J.; Shearier, E. R.; et al. Metallic zinc exhibits optimal biocompatibility for bioabsorbable endovascular stents. Mater. Sci. Eng. C. 2015, 56, 467-72.
130. Khan, A. R.; Grewal, N. S.; Zhou, C.; Yuan, K.; Zhang, H.; Jun, Z. Recent advances in biodegradable metals for implant applications: exploring in vivo and in vitro responses. Results. Eng. 2023, 20, 101526.
131. Moravej, M.; Mantovani, D. Biodegradable metals for cardiovascular stent application: interests and new opportunities. Int. J. Mol. Sci. 2011, 12, 4250-70.
132. Bin, S. J. B.; Fong, K. S.; Chua, B. W.; Gupta, M. Mg-based bulk metallic glasses: a review of recent developments. J. Magnes. Alloys. 2022, 10, 899-914.
133. Manescu, P. V.; Antoniac, I.; Antoniac, A.; et al. Bone regeneration induced by patient-adapted Mg alloy-based scaffolds for bone defects: present and future perspectives. Biomimetics 2023, 8, 618.
134. Vahidgolpayegani, A.; Wen, C.; Hodgson, P.; Li, Y. 2-Production methods and characterization of porous Mg and Mg alloys for biomedical applications. Available from: https://www.sciencedirect.com/science/article/pii/B9780081012895000020. [Last accessed on 6 Mar 2025].
135. Uppal, G.; Thakur, A.; Chauhan, A.; Bala, S. Magnesium based implants for functional bone tissue regeneration-a review. J. Magnes. Alloys. 2022, 10, 356-86.
136. Zheng, K.; Kapp, M.; Boccaccini, A. R. Protein interactions with bioactive glass surfaces: a review. Appl. Mater. Today. 2019, 15, 350-71.
137. Oliver, J. N.; Su, Y.; Lu, X.; Kuo, P. H.; Du, J.; Zhu, D. Bioactive glass coatings on metallic implants for biomedical applications. Bioact. Mater. 2019, 4, 261-70.
138. Yang, Y.; Lu, C.; Yang, M.; et al. Copper-doped mesoporous bioactive glass endows magnesium-based scaffold with antibacterial activity and corrosion resistance. Mater. Chem. Front. 2021, 5, 7228-40.
139. Yazdimamaghani, M.; Razavi, M.; Vashaee, D.; Pothineni, V. R.; Rajadas, J.; Tayebi, L. Significant degradability enhancement in multilayer coating of polycaprolactone-bioactive glass/gelatin-bioactive glass on magnesium scaffold for tissue engineering applications. Appl. Surf. Sci. 2015, 338, 137-45.
140. Metal magnesium market (by application: die casting, aluminum alloys, titanium reduction, iron & steel making) - global industry analysis, size, share, growth, trends, revenue, regional outlook 20222030. Available from: https://www.visionresearchreports.com/metal-magnesium-market/39159. [Last accessed on 6 Mar 2025].
141. Li, M.; Benn, F.; Derra, T.; et al. Microstructure, mechanical properties, corrosion resistance and cytocompatibility of WE43 Mg alloy scaffolds fabricated by laser powder bed fusion for biomedical applications. Mater. Sci. Eng. C. 2021, 119, 111623.
142. Wu, C.; Zai, W.; Man, H. Additive manufacturing of ZK60 magnesium alloy by selective laser melting: parameter optimization, microstructure and biodegradability. Mater. Today. Commun. 2021, 26, 101922.
143. Yang, Y.; Lu, C.; Peng, S.; et al. Laser additive manufacturing of Mg-based composite with improved degradation behaviour. Virt. Phys. Prototyp. 2020, 15, 278-93.
144. Yao, X.; Tang, J.; Zhou, Y.; et al. Surface modification of biomedical Mg-Ca and Mg-Zn-Ca alloys using selective laser melting: corrosion behaviour, microhardness and biocompatibility. J. Magnes. Alloys. 2021, 9, 2155-68.
145. Xu, R.; Zhao, M.; Zhao, Y.; et al. Improved biodegradation resistance by grain refinement of novel antibacterial ZK30-Cu alloys produced via selective laser melting. Mater. Lett. 2019, 237, 253-7.
146. Yin, Y.; Huang, Q.; Liang, L.; et al. In vitro degradation behavior and cytocompatibility of ZK30/bioactive glass composites fabricated by selective laser melting for biomedical applications. J. Alloys. Compd. 2019, 785, 38-45.
147. Shuai, C.; Liu, L.; Zhao, M.; et al. Microstructure, biodegradation, antibacterial and mechanical properties of ZK60-Cu alloys prepared by selective laser melting technique. J. Mater. Sci. Technol. 2018, 34, 1944-52.
148. Sezer, N.; Evis, Z.; Kayhan, S. M.; Tahmasebifar, A.; Koç, M. Review of magnesium-based biomaterials and their applications. J. Magnes. Alloys. 2018, 6, 23-43.
149. Zivic, F.; Grujović, N.; Manivasagam, G.; Richard, C.; Landoulsi, J.; Petrovic, V. The potential of magnesium alloys as bioabsorbable/ biodegradable implants for biomedical applications. Tribol. Ind. 2014, 36, 67-73. Available from: https://www.tribology.rs/journals/2014/2014-1/8.pdf. [Last accessed on 24 Mar 2025]
150. Agarwal, S.; Curtin, J.; Duffy, B.; Jaiswal, S. Biodegradable magnesium alloys for orthopaedic applications: a review on corrosion, biocompatibility and surface modifications. Mater. Sci. Eng. C. 2016, 68, 948-63.
151. Alaneme, K. K.; Kareem, S. A.; Olajide, J. L.; Sadiku, R. E.; Bodunrin, M. O. Computational biomechanical and biodegradation integrity assessment of Mg-based biomedical devices for cardiovascular and orthopedic applications: a review. Int. J. Lightweight. Mater. Manufacture. 2022, 5, 251-66.
152. Bowen, P. K.; Shearier, E. R.; Zhao, S.; et al. Biodegradable metals for cardiovascular stents: from clinical concerns to recent Zn-alloys. Adv. Healthc. Mater. 2016, 5, 1121-40.
153. Jiang, J.; Qian, Y.; Huang, H.; Niu, J.; Yuan, G. Biodegradable Zn-Cu-Mn alloy with suitable mechanical performance and in vitro degradation behavior as a promising candidate for vascular stents. Biomater. Adv. 2022, 133, 112652.
154. Borhani, S.; Hassanajili, S.; Ahmadi, T. S. H.; Rabbani, S. Cardiovascular stents: overview, evolution, and next generation. Prog. Biomater. 2018, 7, 175-205.
155. Negrescu, A.; Necula, M.; Costache, M.; Cimpean, A. In vitro and in vivo biological performance of Mg-based bone implants. Rev. Biol. Biomed. Sci. 2020, 3, 11-41.
156. Rahim, S. A.; Joseph, M. A.; Sampath, K. T. S.; T, H. Recent progress in surface modification of Mg alloys for biodegradable orthopedic applications. Front. Mater. 2022, 9, 848980.
157. Tian, P.; Liu, X. Surface modification of biodegradable magnesium and its alloys for biomedical applications. Regen. Biomater. 2015, 2, 135-51.
158. Papenberg, N. P.; Gneiger, S.; Weißensteiner, I.; Uggowitzer, P. J.; Pogatscher, S. Mg-alloys for forging applications-a review. Materials 2020, 13, 985;.
159. Riaz, U.; Shabib, I.; Haider, W. The current trends of Mg alloys in biomedical applications-a review. J. Biomed. Mater. Res. B. Appl. Biomater. 2019, 107, 1970-96.
160. Bedair, T. M.; Heo, Y.; Ryu, J.; Bedair, H. M.; Park, W.; Han, D. K. Biocompatible and functional inorganic magnesium ceramic particles for biomedical applications. Biomater. Sci. 2021, 9, 1903-23.
161. Canales, D. A.; Reyes, F.; Saavedra, M.; et al. Electrospun fibers of poly (lactic acid) containing bioactive glass and magnesium oxide nanoparticles for bone tissue regeneration. Int. J. Biol. Macromol. 2022, 210, 324-36.
162. Babaremu, K. O.; John, M. E.; Mfoh, U.; Akinlabi, E. T.; Okokpujie, I. P. Behavioral characteristics of magnesium as a biomaterial for surface engineering application. J. Bio. Tribo. Corros. 2021, 7, 579.
163. Dieringa, H.; Hort, N.; Letzig, D.; et al. Mg alloys: challenges and achievements in controlling performance, and future application perspectives. In: Orlov D, Joshi V, Solanki KN, Neelameggham NR, editors. Magnesium technology 2018. Cham: Springer International Publishing; 2018. pp. 3-14.
164. Laws, K. J.; Shamlaye, K. F.; Granata, D.; Koloadin, L. S.; Löffler, J. F. Electron-band theory inspired design of magnesium-precious metal bulk metallic glasses with high thermal stability and extended ductility. Sci. Rep. 2017, 7, 3400.
166. Axinte, E. Metallic glasses from “alchemy” to pure science: present and future of design, processing and applications of glassy metals. Mater. Des. 2012, 35, 518-56.
167. Bonithon, R.; Lupton, C.; Roldo, M.; et al. Open-porous magnesium-based scaffolds withstand in vitro corrosion under cyclic loading: a mechanistic study. Bioact. Mater. 2023, 19, 406-17.
168. Yusop, A. H. M.; Alsakkaf, A.; Kadir, M. R. A.; Sukmana, I.; Nur, H. Corrosion of porous Mg and Fe scaffolds: a review of mechanical and biocompatibility responses. Corros. Eng. Sci. Technol. 2021, 56, 310-26.
169. Kulekci, M. K. Magnesium and its alloys applications in automotive industry. Int. J. Adv. Manuf. Technol. 2008, 39, 851-65.
170. Rahman, M.; Li, Y.; Wen, C. HA coating on Mg alloys for biomedical applications: a review. J. Magnes. Alloys. 2020, 8, 929-43.
172. Gao, J. Design of new metallic glass composites and nanostructured alloys with improved mechanical properties. University of Sheffield; 2016. Available from: https://etheses.whiterose.ac.uk/12404/. [Last accessed on 6 Mar 2025].
173. Zhang, T.; Wang, W.; Liu, J.; Wang, L.; Tang, Y.; Wang, K. A review on magnesium alloys for biomedical applications. Front. Bioeng. Biotechnol. 2022, 10, 953344.
174. Yi, J.; Xia, X. X.; Zhao, D. Q.; Pan, M. X.; Bai, H. Y.; Wang, W. H. Micro‐and nanoscale metallic glassy fibers. Adv. Eng. Mater. 2010, 12, 1117-22.
175. Zberg, B.; Arata, E. R.; Uggowitzer, P. J.; Löffler, J. F. Tensile properties of glassy MgZnCa wires and reliability analysis using Weibull statistics. Acta. Mater. 2009, 57, 3223-31.
176. Lin, C. H.; Huang, C. H.; Chuang, J. F.; Huang, J. C.; Jang, J. S.; Chen, C. H. Rapid screening of potential metallic glasses for biomedical applications. Mater. Sci. Eng. C. 2013, 33, 4520-6.
177. Balasubramanian, S. Magnetron sputtered magnesium-based thin film metallic glasses for bioimplants. Biointerphases 2021, 16, 011005.
178. Kiani, F.; Wen, C.; Li, Y. Prospects and strategies for magnesium alloys as biodegradable implants from crystalline to bulk metallic glasses and composites-a review. Acta. Biomater. 2020, 103, 1-23.
179. Rajan, S. T.; Arockiarajan, A. Thin film metallic glasses for bioimplants and surgical tools: a review. J. Alloys. Compd. 2021, 876, 159939.
180. Butt, M. A. Thin-film coating methods: a successful marriage of high-quality and cost-effectiveness-a brief exploration. Coatings 2022, 12, 1115.
181. Gibson, D. R.; Brinkley, I.; Waddell, E. M.; Walls, J. M. Closed field magnetron sputtering: new generation sputtering process for optical coatings. Adv. Opt. Thin. Films. III. 2008, 7101, 107-18.
182. Baptista, A.; Silva, F.; Porteiro, J.; Míguez, J.; Pinto, G. Sputtering physical vapour deposition (PVD) coatings: a critical review on process improvement and market trend demands. Coatings 2018, 8, 402.
183. Mcclanahan, E. D.; Laegreid, N. Production of thin films by controlled deposition of sputtered material. In: Behrisch R, Wittmaack K, editors. Sputtering by particle bombardment III. Berlin: Springer Berlin Heidelberg; 1991. pp. 339-77.
184. Safi, I. Recent aspects concerning DC reactive magnetron sputtering of thin films: a review. Surf. Coat. Technol. 2000, 127, 203-18.
185. Maissel, L. I.; Schaible, P. M. Thin films deposited by bias sputtering. J. Appl. Phys. 1965, 36, 237-42.
186. Wasa, K.; Kitabatake, M.; Adachi, H. Thin film materials technology: sputtering of control compound materials. Springer Science & Business Media; 2004. p. 518. Available from: https://books.google.com/books?hl=zh-CN&lr=&id=dTmAsG07D00C&oi=fnd&pg=PA1&dq=Wasa+K,+Kitabatake+M,+Adachi+H.+Thin+film+materials+technology:+sputtering+of+control+compound+materials.+Springer+Science+%26+Business+Media%3B+2004.+p.+518.%5B&ots=ck7T0a6jiV&sig=lE6YIkL9Fq0YKB51_09tjoQerFE#v=onepage&q&f=false. [Last accessed on 6 Mar 2025].
187. Garg, R.; Gonuguntla, S.; Sk, S.; et al. Sputtering thin films: materials, applications, challenges and future directions. Adv. Colloid. Interface. Sci. 2024, 330, 103203.
188. Aissani, L.; Alhussein, A.; Zia, A.; Mamba, G.; Rtimi, S. Magnetron sputtering of transition metal nitride thin films for environmental remediation. Coatings 2022, 12, 1746.
189. Wu, E. A. Materials engineering for compatible chemistries in sodium solid-state-batteries and thin-film solid oxide fuel cells. ProQuest; 2024. Available from: https://www.proquest.com/openview/7565d4c01aad601bfa8ec8b32ec14c2f/1?pq-origsite=gscholar&cbl=18750&diss=y. [Last accessed on 6 Mar 2025].
190. Li, Z.; Mi, B.; Ma, X.; et al. Review of thin-film resistor sensors: exploring materials, classification, and preparation techniques. Chem. Eng. J. 2023, 477, 147029.
191. Haque, M. M.; Mahjabin, S.; Islam, M. A.; et al. Modulation of optoelectronic properties of WO3 thin film via Cr doping through RF co-sputtering. Inorg. Chem. Commun. 2025, 114300.
192. Liu, J.; Fu, Y.; Tang, Y.; et al. Thickness dependent structural evolution in Mg-Zn-Ca thin film metallic glasses. J. Alloys. Compd. 2018, 742, 524-35.
193. Yu, H.; Wang, J.; Shi, X.; Louzguine-luzgin, D. V.; Wu, H.; Perepezko, J. H. Ductile biodegradable Mg-based metallic glasses with excellent biocompatibility. Adv. Funct. Mater. 2013, 23, 4793-800.
194. Xu, Z.; Smith, C.; Chen, S.; Sankar, J. Development and microstructural characterizations of Mg-Zn-Ca alloys for biomedical applications. Mater. Sci. Eng. B. 2011, 176, 1660-5.
195. Zhang, S.; Zhang, X.; Zhao, C.; et al. Research on an Mg-Zn alloy as a degradable biomaterial. Acta. Biomater. 2010, 6, 626-40.
196. Zhang, B.; Hou, Y.; Wang, X.; Wang, Y.; Geng, L. Mechanical properties, degradation performance and cytotoxicity of Mg-Zn-Ca biomedical alloys with different compositions. Mater. Sci. Eng. C. 2011, 31, 1667-73.
197. Ortega, Y.; Monge, M. A.; Pareja, R. The precipitation process in Mg-Ca-(Zn) alloys investigated by positron annihilation spectroscopy. J. Alloys. Compd. 2008, 463, 62-6.
198. Farahany, S.; Bakhsheshi-rad, H. R.; Idris, M. H.; Abdul, K. M. R.; Lotfabadi, A. F.; Ourdjini, A. In-situ thermal analysis and macroscopical characterization of Mg-xCa and Mg-0.5Ca-xZn alloy systems. Thermochim. Acta. 2012, 527, 180-9.
199. Xu, X.; Lu, P.; Guo, M.; Fang, M. Cross-linked gelatin/nanoparticles composite coating on micro-arc oxidation film for corrosion and drug release. Appl. Surf. Sci. 2010, 256, 2367-71.
200. Zhang, X.; Wang, Z.; Yuan, G.; Xue, Y. Improvement of mechanical properties and corrosion resistance of biodegradable Mg-Nd-Zn-Zr alloys by double extrusion. Mater. Sci. Eng. B. 2012, 177, 1113-9.
201. Olugbade, T. O.; Abioye, T. E.; Farayibi, P. K.; et al. Electrochemical properties of MgZnCa-based thin film metallic glasses fabricated by magnetron sputtering deposition coated on a stainless steel substrate. Anal. Lett. 2020, 54, 1588-602.
202. Han, Y.; Song, J. Novel Mg2Zr5O12/Mg2Zr5O12-ZrO2-MgF2 gradient layer coating on magnesium formed by microarc oxidation. J. Am. Ceram. Soc. 2009, 92, 1813-6.
203. Zhang, W.; Li, M.; Chen, Q.; Hu, W.; Zhang, W.; Xin, W. Effects of Sr and Sn on microstructure and corrosion resistance of Mg-Zr-Ca magnesium alloy for biomedical applications. Mater. Des. 2012, 39, 379-83.
204. Lu, W.; He, M.; Yu, D.; et al. Ductile behavior and excellent corrosion resistance of Mg-Zn-Yb-Ag metallic glasses. Mater. Des. 2021, 210, 110027.
205. Tsai, P.; Lee, C.; Song, S.; et al. Improved mechanical properties and corrosion resistance of Mg-based bulk metallic glass composite by coating with Zr-based metallic glass thin film. Coatings 2020, 10, 1212.
206. Li, J.; Gittleson, F. S.; Liu, Y.; et al. Exploring a wider range of Mg-Ca-Zn metallic glass as biocompatible alloys using combinatorial sputtering. Chem. Commun. 2017, 53, 8288-91.
207. Zhao, S.; Tayyebi, M.; Mahdireza,; Hu, G. A review of magnesium corrosion in bio-applications: mechanism, classification, modeling, in-vitro, and in-vivo experimental testing, and tailoring Mg corrosion rate. J. Mater. Sci. 2023, 58, 12158-81.
208. Tong, P.; Sheng, Y.; Hou, R.; Iqbal, M.; Chen, L.; Li, J. Recent progress on coatings of biomedical magnesium alloy. Smart. Mater. Med. 2022, 3, 104-16.
209. Kania, A.; Szindler, M. M.; Szindler, M. Structure and corrosion behavior of TiO2 thin films deposited by ALD on a biomedical magnesium alloy. Coatings 2021, 11, 70.
210. Rahman, M.; Dutta, N. K.; Choudhury, N. R. Microroughness induced biomimetic coating for biodegradation control of magnesium. Mater. Sci. Eng. C. 2021, 121, 111811.
211. Heimann, R. B. Magnesium alloys for biomedical application: advanced corrosion control through surface coating. Surf. Coat. Technol. 2021, 405, 126521.
212. Vance, A.; Bari, K.; Arjunan, A. Compressive performance of an arbitrary stiffness matched anatomical Ti64 implant manufactured using direct metal laser sintering. Mater. Des. 2018, 160, 1281-94.
213. Nasello, G.; Vautrin, A.; Pitocchi, J.; et al. Mechano-driven regeneration predicts response variations in large animal model based on scaffold implantation site and individual mechano-sensitivity. Bone 2021, 144, 115769.
214. Bashkuev, M.; Checa, S.; Postigo, S.; Duda, G.; Schmidt, H. Computational analyses of different intervertebral cages for lumbar spinal fusion. J. Biomech. 2015, 48, 3274-82.
215. Md, S. A. P.; Abdul, R. R. A.; Harun, M. N.; et al. The influence of flow rates on the dynamic degradation behaviour of porous magnesium under a simulated environment of human cancellous bone. Mater. Des. 2017, 122, 268-79.
216. Pobloth, A. M.; Checa, S.; Razi, H.; et al. Mechanobiologically optimized 3D titanium-mesh scaffolds enhance bone regeneration in critical segmental defects in sheep. Sci. Transl. Med. 2018, 10, eaam8828.
217. Reddy, T. H.; Pal, S.; Kumar, K. C.; Mohan, M. K.; Kokol, V. Finite element analysis for mechanical response of magnesium foams with regular structure obtained by powder metallurgy method. Procedia. Eng. 2016, 149, 425-30.
218. Cho, S. M.; Yang, B. E.; Kim, W. H.; et al. Biomechanical stability of magnesium plate and screw fixation systems in LeFort I osteotomy: a three-dimensional finite element analysis. Maxillofac Plast Reconstr Surg 2024;46:40.
219. Alshammari, A.; Alabdah, F.; Wang, W.; Cooper, G. Virtual design of 3D-printed bone tissue engineered scaffold shape using mechanobiological modeling: relationship of scaffold pore architecture to bone tissue formation. Polymers 2023, 15, 3918.
220. Jain, S.; Yassin, M. A.; Fuoco, T.; et al. Engineering 3D degradable, pliable scaffolds toward adipose tissue regeneration; optimized printability, simulations and surface modification. J. Tissue. Eng. 2020, 11, 2041731420954316.
221. Loerakker, S.; Ristori, T. Computational modeling for cardiovascular tissue engineering: the importance of including cell behavior in growth and remodeling algorithms. Curr. Opin. Biomed. Eng. 2020, 15, 1-9.
222. Post, J. N.; Loerakker, S.; Merks, R. M. H.; Carlier, A. Implementing computational modeling in tissue engineering: where disciplines meet. Tissue. Eng. Part. A. 2022, 28, 542-54.
223. Donnaloja, F.; Jacchetti, E.; Soncini, M.; Raimondi, M. T. Natural and synthetic polymers for bone scaffolds optimization. Polymers 2020, 12, 905.
224. Wang, L.; Wang, C.; Wu, S.; Fan, Y.; Li, X. Influence of the mechanical properties of biomaterials on degradability, cell behaviors and signaling pathways: current progress and challenges. Biomater. Sci. 2020, 8, 2714-33.
225. Pina, S.; Ribeiro, V. P.; Marques, C. F.; et al. Scaffolding strategies for tissue engineering and regenerative medicine applications. Materials 2019, 12, 1824.
226. Ghosh, R.; Chanda, S.; Chakraborty, D. Application of finite element analysis to tissue differentiation and bone remodelling approaches and their use in design optimization of orthopaedic implants: a review. Int. J. Numer. Method. Biomed. Eng. 2022, 38, e3637.
227. Verma, R.; Kumar, J.; Singh, N. K.; Rai, S. K.; Saxena, K. K.; Xu, J. Design and analysis of biomedical scaffolds using TPMS-based porous structures inspired from additive manufacturing. Coatings 2022, 12, 839.
228. Gryko, A.; Prochor, P.; Sajewicz, E. Finite element analysis of the influence of porosity and pore geometry on mechanical properties of orthopaedic scaffolds. J. Mech. Behav. Biomed. Mater. 2022, 132, 105275.
229. Noordin MA, Saad APB, Ngadiman NHA, Mustafa NS, bin Mohd Yusof N, Ma’aram A. Finite element analysis of porosity effects on mechanical properties for tissue engineering scaffold. Biointerface. Res. Appl. Chem. 2020, 11, 8836-43.
230. Kakarla, A. B.; Kong, I.; Nukala, S. G.; Kong, W. Mechanical behaviour evaluation of porous scaffold for tissue-engineering applications using finite element analysis. J. Compos. Sci. 2022, 6, 46.
231. Putra, R. U.; Prakoso, A. T.; Nugrasyah, A.; et al. Fatigue prediction of porous magnesium bone scaffold using finite element method. 4th Forum in Research, Science, and Technology (FIRST-T1-T2-2020). Palembang Indonesia. Atlantis Press; 2021
232. Maslov, L. B. Biomechanical model and numerical analysis of tissue regeneration within a porous scaffold. Mech. Solids. 2020, 55, 1115-34.
233. Jahir-hussain, M. J.; Maaruf, N. A.; Esa, N. E. F.; Jusoh, N. The effect of pore geometry on the mechanical properties of 3D-printed bone scaffold due to compressive loading. IOP. Conf. Ser. Mater. Sci. Eng. 2021, 1051, 012016.
234. Joshi, A.; Dias, G.; Staiger, M. P. In silico modelling of the corrosion of biodegradable magnesium-based biomaterials: modelling approaches, validation and future perspectives. Biomater. Transl. 2021, 2, 257-71.
235. Boland, E. L.; Shine, C. J.; Kelly, N.; Sweeney, C. A.; McHugh, P. E. A review of material degradation modelling for the analysis and design of bioabsorbable stents. Ann. Biomed. Eng. 2016, 44, 341-56.
236. Kovacevic, S.; Ali, W.; Martínez-Pañeda, E.; LLorca, J. Phase-field modeling of pitting and mechanically-assisted corrosion of Mg alloys for biomedical applications. Acta. Biomater. 2023, 164, 641-58.
237. Liu, B.; Liu, J.; Wang, C.; et al. High temperature oxidation treated 3D printed anatomical WE43 alloy scaffolds for repairing periarticular bone defects: In vitro and in vivo studies. Bioact Mater 2024;32:177-89.
238. Liebl, H.; Garcia, E. G.; Holzner, F.; et al. In-vivo assessment of femoral bone strength using finite element analysis (FEA) based on routine MDCT imaging: a preliminary study on patients with vertebral fractures. PLoS. One. 2015, 10, e0116907.
239. Xu, J.; Wang, K.; Gao, M.; Tu, Z.; Zhang, S.; Tan, J. Biomechanical performance design of joint prosthesis for medical rehabilitation via generative structure optimization. Comput. Methods. Biomech. Biomed. Engin. 2020, 23, 1163-79.
240. Nourisa, J. The application of agent-based modeling and fuzzy-logic controllers for the study of magnesium biomaterials. Available from: https://macau.uni-kiel.de/receive/macau_mods_00004014. [Last accessed on 6 Mar 2025].
241. Nourisa, J.; Zeller-Plumhoff, B.; Helmholz, H.; Luthringer-Feyerabend, B.; Ivannikov, V.; Willumeit-Römer, R. Magnesium ions regulate mesenchymal stem cells population and osteogenic differentiation: a fuzzy agent-based modeling approach. Comput. Struct. Biotechnol. J. 2021, 19, 4110-22.
242. Perier-Metz, C.; Cipitria, A.; Hutmacher, D. W.; Duda, G. N.; Checa, S. An in silico model predicts the impact of scaffold design in large bone defect regeneration. Acta. Biomater. 2022, 145, 329-41.
243. Pant, A.; Paul, E.; Niebur, G. L.; Vahdati, A. Integration of mechanics and biology in computer simulation of bone remodeling. Prog. Biophys. Mol. Biol. 2021, 164, 33-45.
244. Lee, P. S.; Sriperumbudur, K. K.; Dawson, J.; van, R. U.; Appali, R. Mathematical models on bone cell homeostasis and kinetics in the presence of electric fields: a review. Prog. Biomed. Eng. 2024, 7, 012004.
245. Gaziano, P.; Marino, M. Computational modeling of cell motility and clusters formation in enzyme-sensitive hydrogels. Meccanica 2024, 59, 1335-49.
246. Santos BC, Noritomi PY, da Silva JVL, Maia IA, Manzini BM. Biological multiscale computational modeling: a promising tool for 3D bioprinting and tissue engineering. Bioprinting 2022, 28, e00234.
247. Gaziano, P.; Marino, M. A phase-field model of cell motility in biodegradable hydrogel scaffolds for tissue engineering applications. Comput. Mech. 2024, 74, 45-66.
248. Pires, T.; Dunlop, J. W. C.; Fernandes, P. R.; Castro, A. P. G. Challenges in computational fluid dynamics applications for bone tissue engineering. Proc. Math. Phys. Eng. Sci. 2022, 478, 20210607.
249. Omar, A. M.; Hassan, M. H.; Daskalakis, E.; et al. Geometry-based computational fluid dynamic model for predicting the biological behavior of bone tissue engineering scaffolds. J. Funct. Biomater. 2022, 13, 104.
250. Kumar, M.; Mohol, S. S.; Sharma, V. A computational approach from design to degradation of additively manufactured scaffold for bone tissue engineering application. RPJ. 2022, 28, 1956-67.
251. d’Adamo, A.; Salerno, E.; Corda, G.; et al. Experimental measurements and CFD modelling of hydroxyapatite scaffolds in perfusion bioreactors for bone regeneration. Regen. Biomater. 2023, 10, rbad002.
252. Channasanon, S.; Kaewkong, P.; Chantaweroad, S.; et al. Scaffold geometry and computational fluid dynamics simulation supporting osteogenic differentiation in dynamic culture. Comput. Methods. Biomech. Biomed. Engin. 2024, 27, 587-98.
253. Manescu, P. V.; Paltanea, G.; Antoniac, A.; et al. Mechanical and computational fluid dynamic models for magnesium-based implants. Materials 2024, 17, 830.
254. Wu, C.; Entezari, A.; Zheng, K.; et al. A machine learning-based multiscale model to predict bone formation in scaffolds. Nat. Comput. Sci. 2021, 1, 532-41.
255. Li, H.; Liu, Y.; Pang, S.; Liaw, P. K.; Zhang, T. Corrosion fatigue behavior of a Mg-based bulk metallic glass in a simulated physiological environment. Intermetallics 2016, 73, 31-9.
256. Jiang, L.; Bao, M.; Dong, Y.; Yuan, Y.; Zhou, X.; Meng, X. Processing, production and anticorrosion behavior of metallic glasses: a critical review. J. Non-Cryst. Solids. 2023, 612, 122355.
257. Cao, J. D.; Kirkland, N. T.; Laws, K. J.; Birbilis, N.; Ferry, M. Ca-Mg-Zn bulk metallic glasses as bioresorbable metals. Acta. Biomater. 2012, 8, 2375-83.
258. rajendran R, Aggarwal D, Bonvalet Rolland M, Gruescu C, Shabadi R. Design and development of large-diameter Mg-Zn-Ca bulk metallic glass for biomedical applications: a mechanical and corrosion perspective. Intermetallics 2024, 175, 108520.
259. García-Aznar, J. M.; Nasello, G.; Hervas-Raluy, S.; Pérez, M. Á.; Gómez-Benito, M. J. Multiscale modeling of bone tissue mechanobiology. Bone 2021, 151, 116032.
260. Kendall, J. J.; Ledoux, C.; Marques, F. C.; et al. An in silico micro-multiphysics agent-based approach for simulating bone regeneration in a mouse femur defect model. Front. Bioeng. Biotechnol. 2023, 11, 1289127.
261. Shen, J.; Yong, L.; Chen, B.; et al. Effect of biocomposite mediated magnesium ionic micro-homeostasis on cell fate regulation and bone tissue regeneration. Compos. Part. B. Eng. 2023, 265, 110961.
262. Zhang, X.; Hao, Z. Computational models of magnesium medical implants degradation: a review. J. Phys. Conf. Ser. 2021, 1838, 012012.
263. Cai, Z.; Du, P.; Li, K.; Chen, L.; Xie, G. A review of the development of titanium-based and magnesium-based metallic glasses in the field of biomedical materials. Materials 2024, 17, 4587.
264. Dutta, S.; Roy, M. Recent developments in engineered magnesium scaffolds for bone tissue engineering. ACS. Biomater. Sci. Eng. 2023, 9, 3010-31.
265. Yao, X.; Tang, J.; Zhou, Y.; et al. Selective laser melting of an Mg/metallic glass hybrid for significantly improving chemical and mechanical performances. Appl. Surf. Sci. 2022, 580, 152229.
266. Lebrun, N.; Dupla, F.; Bruhier, H.; et al. Metallic glasses for biological applications and opportunities opened by laser surface texturing: a review. Appl. Surf. Sci. 2024, 670, 160617.
267. Ramya, M. Advances in biodegradable orthopaedic implants: optimizing magnesium alloy corrosion resistance for enhanced bone repair. Biomed. Mater. Devices. 2025, 3, 396-414.
268. Zhou, H.; Liang, B.; Jiang, H.; Deng, Z.; Yu, K. Magnesium-based biomaterials as emerging agents for bone repair and regeneration: from mechanism to application. J. Magnes. Alloys. 2021, 9, 779-804.
269. Wang, H.; Yuan, H.; Wang, J.; et al. Influence of the second phase on protein adsorption on biodegradable Mg alloys’ surfaces: comparative experimental and molecular dynamics simulation studies. Acta. Biomater. 2021, 129, 323-32.
270. Cerqueira, A.; Romero-Gavilán, F.; García-Arnáez, I.; et al. Characterization of magnesium doped sol-gel biomaterial for bone tissue regeneration: the effect of Mg ion in protein adsorption. Mater. Sci. Eng. C. 2021, 125, 112114.
271. Wang, X.; Wang, C.; Chu, C.; Xue, F.; Li, J.; Bai, J. Structure-function integrated biodegradable Mg/polymer composites: design, manufacturing, properties, and biomedical applications. Bioact. Mater. 2024, 39, 74-105.
272. Zhao, Y. Understanding and design of metallic alloys guided by phase-field simulations. npj. Comput. Mater. 2023, 9, 1038.
273. Wang, J.; Meng, L.; Xie, W.; et al. Corrosion and in vitro cytocompatibility investigation on the designed Mg-Zn-Ag metallic glasses for biomedical application. J. Magnes. Alloys. 2024, 12, 1566-80.
274. Xu, L.; Liu, X.; Sun, K.; Fu, R.; Wang, G. Corrosion behavior in magnesium-based alloys for biomedical applications. Materials 2022, 15, 2613.
275. Rout, P. K.; Roy, S.; Ganguly, S.; Rathore, D. K. A review on properties of magnesium-based alloys for biomedical applications. Biomed. Phys. Eng. Express. 2022, 8, 042002.
276. Wang, P.; Mao, Y.; Zhou, X.; Wang, M.; He, M. Surface microstructure and corrosion resistance characterization of Mg-based amorphous alloys. J. Mater. Sci. 2024, 59, 20050-67.
277. Guo, J. L.; Januszyk, M.; Longaker, M. T. Machine learning in tissue engineering. Tissue. Eng. Part. A. 2023, 29, 2-19.
278. Salem, D. A.; Moharam, M. H.; Hashem, E. M. Development of machine learning regression models for predicting the performance of nanofibrous scaffolds for skin tissue engineering. J. Bio-X. Res. 2024, 7, 0008.
279. Gharibshahian, M.; Torkashvand, M.; Bavisi, M.; Aldaghi, N.; Alizadeh, A. Recent advances in artificial intelligent strategies for tissue engineering and regenerative medicine. Skin. Res. Technol. 2024, 30, e70016.
280. Nosrati, H.; Nosrati, M. Artificial intelligence in regenerative medicine: applications and implications. Biomimetics 2023, 8, 442.
282. Fan, J.; Xu, J.; Wen, X.; et al. The future of bone regeneration: artificial intelligence in biomaterials discovery. Mater. Today. Commun. 2024, 40, 109982.
283. Mai, T. T.; Nguyen, P. H.; Haque, N. A. N. M. M.; Pemen, G. A. J. M. Exploring regression models to enable monitoring capability of local energy communities for self-management in low-voltage distribution networks. IET. Smart. Grid. 2022, 5, 25-41.
284. Chen, J.; Dou, Y.; Li, S.; Xu, B.; Wang, T. Eutectic and bulk metallic glasses interpretation of Ca(Zr,Ti,Mg,Fe)-based binary biomedical materials via dual-cluster formulas model. Appl. Phys. A. 2024, 130, 8034.
285. Zhang, J. Y.; Zhou, Z. Q.; Zhang, Z. B.; et al. Recent development of chemically complex metallic glasses: from accelerated compositional design, additive manufacturing to novel applications. Mater. Futures. 2022, 1, 012001.
286. Wang, J.; Wang, C.; Rao, W.; Jung, I. Design and characterization of biodegradable Mg-Zn-Ag metallic glasses. Trans. Nonferrous. Met. Soc. China. 2024, 34, 2814-27.
287. Musthafa H, Walker J, Domagala M. Computational modelling and simulation of scaffolds for bone tissue engineering. Computation 2024, 12, 74.
288. Bin, S. J. B.; Fong, K. S.; Chua, B. W.; Gupta, M. Development of biocompatible bulk MgZnCa metallic glass with very high corrosion resistance in simulated body fluid. Materials 2022, 15, 8989.
289. Zhang, P.; Tan, J.; Tian, Y.; Yan, H.; Yu, Z. Research progress on selective laser melting (SLM) of bulk metallic glasses (BMGs): a review. Int. J. Adv. Manuf. Technol. 2022, 118, 2017-57.
290. Addissouky, T. A. Transforming toxicity assessment through microphysiology, bioprinting, and computational modeling. ACT. 2024, 9, 1-14.
291. Jabed, A.; Bhuiyan, M. N.; Haider, W.; Shabib, I. Distinctive features and fabrication routes of metallic-glass systems designed for different engineering applications: a review. Coatings 2023, 13, 1689.
292. Rajan S, Karthika M, Bendavid A, Subramanian B. Apatite layer growth on glassy Zr48Cu36Al8Ag8 sputtered titanium for potential biomedical applications. Appl. Surf. Sci. 2016, 369, 501-9.
293. Rajan, S. T.; Das, M.; Kumar, P. S.; Arockiarajan, A.; Subramanian, B. Biological performance of metal metalloid (TiCuZrPd:B) TFMG fabricated by pulsed laser deposition. Colloids. Surf. B. Biointerfaces. 2021, 202, 111684.
294. Yiu, P.; Diyatmika, W.; Bönninghoff, N.; Lu, Y.; Lai, B.; Chu, J. P. Thin film metallic glasses: properties, applications and future. J. Appl. Phys. 2020, 127, 030901.
295. Celarek, A.; Kraus, T.; Tschegg, E. K.; et al. PHB, crystalline and amorphous magnesium alloys: promising candidates for bioresorbable osteosynthesis implants? Mater. Sci. Eng. C. 2012, 32, 1503-10.
296. Hua, N.; Zhang, X.; Liao, Z.; et al. Dry wear behavior and mechanism of a Fe-based bulk metallic glass: description by Hertzian contact calculation and finite-element method simulation. J. Non-Cryst. Solids. 2020, 543, 120065.
297. Li, Y. Laser welding of metallic glass to crystalline metal in laser- foil-printing additive manufacturing. ProQuest. 2019. Available from: https://www.proquest.com/openview/968eddffd4c9393dc4440afe1475f31a/1?pq-origsite=gscholar&cbl=51922&diss=y. [Last accessed on 6 Mar 2025].
Cite This Article

How to Cite
Download Citation
Export Citation File:
Type of Import
Tips on Downloading Citation
Citation Manager File Format
Type of Import
Direct Import: When the Direct Import option is selected (the default state), a dialogue box will give you the option to Save or Open the downloaded citation data. Choosing Open will either launch your citation manager or give you a choice of applications with which to use the metadata. The Save option saves the file locally for later use.
Indirect Import: When the Indirect Import option is selected, the metadata is displayed and may be copied and pasted as needed.
About This Article
Special Issue
Copyright
Data & Comments
Data
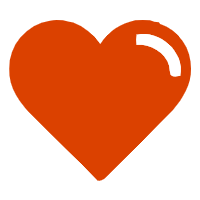
Comments
Comments must be written in English. Spam, offensive content, impersonation, and private information will not be permitted. If any comment is reported and identified as inappropriate content by OAE staff, the comment will be removed without notice. If you have any queries or need any help, please contact us at [email protected].