Single-atom catalysts for (photo)electrocatalytic biomass valorization to high-value-added chemicals
Abstract
As a crucial renewable energy resource, biomass can be converted into high-value-added chemicals via unique catalytic routes, which facilitate the reduction of excessive dependency on fossil resources. However, the complex functional groups inherent in biomass and biomass-derived compounds enable considerable difficulties for their selective functionalization. The precise cleavage of special chemical bonds in biomass highly depended on the structure design of catalysts. Single-atom catalysts (SACs) have garnered significant attention in biomass valorization through the electrocatalytic and photoelectrocatalytic processes due to their maximal atom utilization efficiency, unique electronic structure, and tunable coordination environments. The present review outlines the latest research progress in this emerging field, focusing on the (photo)electrocatalytic application of SACs in biomass valorization, including cellulose-derived and hemicellulose-derived compounds and lignin. We also emphasize the innovative design and precise modulation of atomically dispersed metal active sites at the atomic level. Through state-of-the-art catalytic systems, we elaborately discuss the structure-activity relationship and elucidate the mechanisms of the (photo)electrocatalytic processes over SACs. Finally, we provide the prospects of SACs in (photo)electrocatalytic biomass valorization.
Keywords
INTRODUCTION
Biomass, as the only form of renewable carbon resource, has great advantages for preparing fine chemicals replacing fossil resources, such as abundant supply, widespread distribution, zero CO2 emissions, and recycling utilization. It is the fourth-largest energy source, following only oil, coal, and natural gas. With the increasingly serious shortage of fossil energy and environmental pollution, the high-value utilization of biomass-based platform molecules as a renewable and clean resource has become an important worldwide research topic[1]. Among biomass existence forms, lignocellulosic biomass is the most abundant, with readily available sources and a fast regeneration rate, making it a promising alternative to petroleum resources[2]. Lignocellulosic biomass is composed of cellulose (40%-60%), hemicellulose (20%-40%) and lignin (an aromatic oxygen-containing polymer, 10%-25%)[3,4]. After hydrolysis of cellulose and hemicellulose, hexose (mannose, glucose, galactose)[5] and pentose (xylose, arabinose) can be obtained, which can be further dehydrated to obtain 5-hydroxymethylfurfural (HMF)[6,7], furfural (FUR)[8], 2-methylfuran (2-MF), glycerol, ethanol[9], methanol[10] and other biomass platform compounds. Lignin mainly comprises three phenylpropane structures (syringyl, guaiacyl, and hydroxyphenyl)[11]. By breaking the C-C bond, it is converted into highly functional monomer aromatic compounds[12-14]. These compounds can be used as fine chemicals directly, and they can also continue to be converted directly or indirectly into a variety of valuable compounds through reduction or oxidation routes.
In the early years, the study on the valorization of biomass-based platform molecules is mainly based on thermal catalytic upgrading[4,15,16]. It includes homogeneous catalytic systems (organic metal complexes, metal salts, etc.)[17-19] and heterogeneous catalytic systems (bulk metal oxides, supported noble
Due to the dual impact of energy shortage and environmental crisis, the development of catalytic conversion processes driven by renewable energy (wind, solar, tidal energy, etc.) has attracted wide attention[21-32]. Advanced catalytic technologies, such as photoelectrocatalysis and electrocatalysis, offer significant advantages in catalysis. Firstly, photoelectrocatalysis and electrocatalysis can stimulate the electronic structure of reactant molecules through external energy (light or electric energy) and reduce the activation energy of the reaction, thus enabling highly efficient catalytic transformations under conditions that are beyond the reach of traditional thermal catalytic reactions. Secondly, these two catalytic methods can precisely control catalytic reactions. By adjusting the external light intensity or current density, the reaction rate, selectivity, and product distribution can be precisely controlled. Furthermore, photoelectrocatalysis and electrocatalysis can usually be carried out under milder conditions, reducing energy consumption and environmental pollution, which aligns with the development trend of green catalysis. In contrast to traditional thermal catalysis, these two approaches have a more extensive range of applications and can catalyze reactions that are challenging to achieve through conventional thermal catalysis, such as photocatalytic water decomposition and electrochemical synthesis. (Photo)electrocatalytic processes offer prospects for synthesizing value-added chemicals using biomass-based platform molecules under mild reaction conditions, which are easy to control and environmentally friendly. These methods are expected to become highly efficient green technologies for energy sustainable development[33,34]. Intermediates or free radicals generated from biomass dehydrogenation can further transform into multicarbon compounds through carbon-carbon coupling reactions[35,36]. However, achieving effective C-H activation and controllable C-C coupling in photoelectrochemical/electrocatalytic biomass valorization remains challenging. The kinetic barrier of C-C coupling on metal sites of single- or dual-atom catalysts and the modulation of intermediate adsorption strength are critical factors influencing catalytic performance[36,37]. Yang et al. elucidated the reasons behind the continued difficulty in carbon-carbon coupling on dual-atom electrocatalysts, providing new insights for enhancing reaction selectivity and activity[37]. A primary hurdle in (photo)electrocatalytic biomass conversion lies in crafting cutting-edge catalysts that exhibit selectivity, stability, and superior performance in operando conditions.
Single-atom catalysts (SACs) are a unique type of supported metal catalysts with almost 100% of the metal atoms exposed on the surface, providing outstanding catalytic efficiency, activity, and selectivity. These catalysts offer distinctive advantages over metals and metal X-ides. They feature individual metal atoms as active sites, which enables precise catalytic activity and influences reaction selectivity in a manner that differs from that of metal or oxide particles, reducing catalyst usage. Furthermore, the atomic-level dispersion of SACs confers increased resistance to poisoning, thereby enhancing stability and prolonging the lifespan of the catalyst. The effective adsorption and deactivation of toxic substances achieve this. Moreover, the tunability of SACs at the atomic level allows for the optimization of catalytic performance by adjusting the metal type, number, and positioning, thereby enabling the customization of the catalyst for diverse reaction requirements.
The stability of metal atoms within the SAC structure is a significant challenge. The interaction between metal atoms and the support in SACs significantly influences the dispersion and stability of these metal atoms. Enhanced metal-support interactions can stabilize the position of metal atoms and consequently elevate the stability of the catalyst. This can be achieved through engineered designs at the atomic scale or by introducing specific ligands to stabilize metal atoms. The stability of SACs under varying environmental conditions is also a crucial consideration. For instance, elevated temperatures, pressures, or oxidative/reductive environments may trigger catalyst deactivation or degradation. Additionally, the stability of SACs during repeated usage or recycling processes is paramount. The catalyst should uphold stable catalytic activity and selectivity throughout these cycles. Recently, a spectrum of synthesis methods for SACs has been reported, with precise control over synthesis conditions to enhance catalyst stability.
SACs have garnered significant research attention over the past decade due to their great potential to significantly enhance catalytic activity and selectivity with high catalyst atomic economy[38-46]. They have exhibited excellent catalytic performance in numerous thermocatalytic reactions involving biomass-based platform molecules[47-56]. Several reviews on their application in biomass valorization have been reported[43,57,58]. Vasconcelos et al. provided a comprehensive overview of the utilization of SACs and single-atom alloys (SAA) for enhancing the catalytic conversion of carbohydrates, formic acid, and other molecules. They also focused on the preparation and characterization of these materials and gain an insight into the potential mechanisms involved during the reactions[43]. De et al. summarized recent studies on SAC applications in selectively transforming biomass-based platform molecules into value-added products, highlighting their unique catalytic activities and discussing fundamental insights into their superior performance[57]. Wang et al. presented a comprehensive review of defect engineering in preparing SACs and provided an in-depth analysis of upgrading biomass-based platform molecules using SACs[59]. Lu et al. reviewed examples of fuels and value-added chemicals production from biomass-based platform molecules and CO2 using SACs[60].
SACs play a central role in electrocatalytic and photoelectrocatalytic reactions, although significant differences exist in their structures, properties, applications, and mechanisms of action. In electrocatalysis, SAC-based electrocatalysts are typically composed of carbon supports or metal oxides as substrate materials, with single atoms dispersed on the surface by in situ reduction or physical adsorption techniques. These catalysts modulate the electronic structure of surface adsorption states and active sites, facilitating electron transfer and controlling reaction pathways to achieve efficient electrocatalytic conversions. Conversely, photoelectrocatalysts for light-driven catalysis typically require manipulating material band structures and surface morphologies to enable efficient light absorption and separation of photogenerated carriers, thereby enhancing photocatalytic performance. As a result, SACs for photoelectrocatalysis must possess excellent electrocatalytic capabilities and demonstrate proficient light absorption and photogenerated carrier separation capabilities to utilize light energy fully for catalytic activation.
In recent years, significant research efforts have been devoted to biomass valorization using SACs through electrocatalytic and photoelectrocatalytic methods, aiming to convert efficiently biomass-based compounds into valuable chemicals and fuels[61]. However, detailed reviews have not been reported on the (photo)electrocatalytic conversion of biomass-based compounds using SACs. This review summarizes recent state-of-the-art research on biomass valorization via the (photo)electrocatalytic approaches, highlighting the remarkable performance of SACs in biomass catalytic upgrading. Specifically, we focus on the (photo)electrocatalytic application of SACs for biomass-based compounds, providing illustrative examples. Additionally, the unique structure-activity relationship is discussed in detail. We also elucidate the mechanisms of the (photo)electrocatalytic processes facilitated by SACs. Finally, we provide the prospects of SACs in electrocatalytic biomass conversion.
SACs FOR CELLULOSE AND HEMICELLULOSE DERIVED MOLECULES VALORIZATION
Biomass valorization can be achieved both at the anode and the cathode. The anodic electrocatalytic oxidation (ECO) reaction is pivotal in this area and has been thoroughly researched. The high-value-added oxidative products (2,5-furandicarboxylic acid (FDCA), gluconic acid, formate, etc.) are key intermediates in the pharmaceutical and chemical industry.
The upgrading of aldehyde compounds
After the hydrolysis of cellulose and hemicellulose, sugar compounds are produced, which subsequently undergo dehydration, retro-aldol condensation, or other reactions to generate aldehyde compounds such as HMF and FUR. These products hold significant promise in biomass conversion and utilization, which can be utilized to prepare biomass-based chemicals, such as biofuels and other high-value-added compounds.
Electrocatalytic upgrading of HMF
The electrooxidation process can convert HMF into high-value-added products [Table 1]. Among the oxidation products of HMF, FDCA emerges as exceptionally promising. Not only can it substitute terephthalic acid, a petroleum-derived monomer used in various polyesters, in the synthesis of biodegradable polymers, but it is also widely utilized as an important intermediate for fragrances and as a chelating agent of metals, among other uses. SACs have been extensively designed for the HMF oxidation reaction (HMFOR). Metal oxide supports-confined noble metal single atoms represent one type of SAC. Due to the electron interaction between the metal single atoms and oxide support, the electron density at various atomic sites will be redistributed. This can regulate the adsorption energy of HMF and intermediates, thus enhancing the electrocatalytic performance of HMFOR.
Various SACs for HMF valorization
![]() | |||
Entry | Catalyst | Catalytic activity | Ref. |
1 | Ir-Co3O4 | 98% FE, 98% FDCA yield | [62] |
2 | Ru1-NiO | 42.5% DFF yield | [63] |
3 | Ru/CoOx | 55.2% FE, 76.5% FDCA selectivity, and 55.0% FDCA yield | [64] |
4 | Ru0.3/NiFe LDH | 99.24% FDCA selectivity and 98.68% FDCA yield | [65] |
5 | Rh-SA/NiFe NMLDH | Nearly 100% FE, long-term stability of more than 100 h | [66] |
6 | Ru1Cu SAA | 0.47 mmol cm-2 h-1 DHMF formation rate, 85.6% FE | [67] |
In 2021, Lu et al. reported an Ir-Co3O4 catalyst for HMFOR[62]. High-angle annular dark-field scanning transmission electron microscopy (HAADF-STEM) characterization showed that the SAC was composed of highly dispersed Ir. The linear sweep voltammetry (LSV) curves show a lower onset potential and higher current density for Ir-Co3O4 than Co3O4 and IrO2. This indicates that HMFOR has higher activity on
Figure 1. (A) OCP curves of Ir-Co3O4 and Co3O4. TPD spectra of Ir-Co3O4 and Co3O4 at HMF/He (B), ethylene (C), and CO (E) atmospheres. (D) The adsorption model of HMF molecules on Ir-Co3O4. (F) The electrocatalytic performance of Ir-Co3O4. Copyright 2021, Wiley[62].
Ge et al. achieved efficient selective oxidation of biomass-derived alcohols, resulting in various high-value-added aldehyde products, using a single-atom ruthenium catalyst supported on oxidized nickel
Figure 2. (A) XPS spectra of Ru 3p for Ru1-NiO. (B) XANES spectra of Ru K-edge. (C) The electrocatalytic performance of Ru1-NiO. CV curves of (D) NiO, (E) Ru1-NiO. (F) The ex situ Raman spectra of Ru1-NiO. Copyright 2022, Wiley[63].
In 2023, Gu et al. reported a single-atom Ru catalyst with a 0.5 wt% Ru loading anchored on the surface of CoOx, wherein the presence of Ru played a crucial part in enhancing HMFOR activity[64]. HAADF-STEM shows that the Ru species are atomically dispersed on the CoOx substrate [Figure 3A]. The X-ray diffraction (XRD) pattern reveals the absence of distinct diffraction peaks corresponding to Ru or RuO2, indicating a high degree of dispersion for Ru on ultrathin CoOx [Figure 3B]. XPS and CV results have shown that the single atom Ru can increase the proportion of Co3+ in CoOx and accelerate the reaction kinetics for generating active species of Co4+ [Figure 3C and D]. Notably, as shown in Figure 3E, Ru/CoOx exhibited significantly superior HMFOR performance compared to CoOx catalysts. Furthermore, a more significant reduction of OCP (0.4 V) was observed for the single-atom Ru/CoOx electrode compared to that of the contrasting catalyst electrodes, demonstrating stronger surface adsorption of HMF on single-atom Ru/CoOx [Figure 3F]. From the HMF electrolysis, a FE of 55.2%, FDCA selectivity of 76.5%, and a yield of 55.0% were obtained (Table 1, entry 3). This takes place via the HMFCA pathway, as evidenced by high-performance liquid chromatography (HPLC). The single-atom Ru/CoOx electrode exhibited excellent cyclic stability for HMFOR.
Figure 3. (A) AC HAADF-STEM image of Ru1/CoOx. (B) XRD pattern and (C) XPS spectra of Co 2p for CoOx and Ru1/CoOx. (D) CV curves of Ru1/CoOx and CoO. (E) LSV curves of Ru1/CoOxand CoOx. (F) OCP curves of Ru1/CoOxand CoOx. Copyright 2023, ACS[64].
NiFe layered double hydroxide (LDH) catalyst has widely been studied for HMFOR and oxygen evolution reaction (OER). In order to enhance the number of active sites, extensive research has been conducted on the incorporation of single atoms onto the surface of NiFe-LDH, thereby maximizing atom utilization efficiency. Regarding noble metals, the exceptional cost-effectiveness of Ru, combined with the optimal bond strength between Ru and the intermediate species, distinguishes the single-atom Ru catalyst in terms of its outstanding performance.
Xu et al. reported a Ru0.3/NiFe LDH catalyst for HMFOR, which was synthesized using the two-step electrodeposition technique [Figure 4A][65]. Raman spectroscopic characterization confirmed the presence of high valence NiOOH as the reactive species. Doping of Ru into the crystal structure of NiFe-LDH modifies its electronic structure, thereby enhancing the adsorption energy of HMF and intermediate species [Figure 4B]. Single Ru atom also facilitated the conversion of HMF to DFF. Ru0.3/NiFe LDH demonstrated remarkable performance with an HMF conversion efficiency of 99.43%, an FDCA selectivity of 99.24%, and a yield of 98.68% (Table 1, entry 4).
Figure 4. (A) The synthesis strategy for Ru0.3/NiFe LDH catalyst. (B) Ru0.3/NiFe catalytic process of HMFOR. Copyright 2023, Elsevier[65]. (C) Schematic illustration of the HMFOR mechanism over Rh-SA/NiFe NMLDH. (D) The adsorption energy comparisons of HMF and *OH. (E) The electrochemical cycling stability of Rh-SA/NiFe LDH. Copyright 2023, ACS[66].
In 2023, Zeng et al. reported the design and preparation for a catalyst with a 1.27 wt% Rh loading, anchored on the surface of nanoporous mesh-type NiFe LDH (Rh-SA/NiFe NMLDH)[66]. Based on the mechanistic studies, they proposed that the presence of Rh at the atomic level not only facilitates the promotion of chemisorption and activation of HMF, but also enhances the in-situ generation of electrophilic OHads at the Ni site [Figure 4C]. DFT calculations indicate the optimization of electronic structures for Rh-4d and Ni-3d and the reduction of barriers for electron transfer between active sites towards crucial intermediates HMF and OH* [Figure 4D]. Remarkably, the Rh-SA/NiFe NMLDH with a 100% FDCA FE exhibits an excellent long-term stability of more than 100 h (Table 1, entry 5) and great cyclic stability [Figure 4E].
In 2023, Zhou et al. described a catalyst consisting of single copper atoms supported on nitrogen-doped carbon nanosheets (Cu/NCNSs) and evaluated its catalytic performance in the electro-oxidation of twelve biomass platform compounds[68]. The single copper atoms anchored on NCNSs provide numerous active sites, which are significantly distinct from those on the Cu nanoparticles (NPs) in terms of HMF electrooxidation. The mechanism of Cu/NCNSs and Cu NPs in HMFOR is illustrated in Figure 5.
HMF, as an important C6 biomass derivative, has received wide attention for its catalytic hydrogenation to produce high-value-added products. Wu et al. reported a palladium monoatomic electrocatalyst supported on TiO2 for the selective electroreduction of HMF to dimethylfuran (DMF) in a neutral electrolyte
Figure 6. (A) Schematic illustration of electrochemical hydrogenation of HMF. Schematic illustration of the HMFRR process on (B) TiO2, (C) Pd NPs/TiO2, and (D) Pd SA/TiO2. Copyright 2023, Elsevier[69]. The electrochemical reduction processes of HMF on (E) Ru1Cu and (F) Cu.
A ruthenium-copper SAA (Ru1Cu SAA) catalyst was reported by Ji et al., which achieved efficient electrocatalytic hydrogenation (ECH) of HMF to produce DHMF[67]. Employing characterization techniques such as HAADF-STEM, X-ray absorption fine structure spectroscopy (XAFS), and CO-adsorption diffuse reflectance infrared Fourier transform spectroscopy (CO-DRIFTS), it was demonstrated that Ru is atomically dispersed on Cu nanowires. At -0.3 V vs. RHE, Ru1Cu SAA showed a higher DHMF formation rate (0.47 mmol cm-2 h-1) and FE (85.6%) (Table 1, entry 6). Moreover, in high HMF concentrations (50 and 100 mM), the FE of Ru1Cu SAA is significantly higher than Cu, showing its application potential in high concentrations. The authors carried out kinetic studies using techniques such as CV, in situ electrochemical impedance spectroscopy, and kinetic isotope effects (KIE) to investigate the kinetics of HMF electrochemical reduction on Ru1Cu and Cu catalysts. They observed different mechanisms for the electrochemical reduction of HMF on Ru1Cu and Cu. In the case of Ru1Cu SAA catalyst, with or without the presence of HMF, there was no change in the rate-determining step (RDS) of the hydrogen evolution reaction (HER) and HMF reduction reaction (HMFRR). This suggests that the hydrogenation of HMF on Ru1Cu follows an electrochemical hydrogenation (ECH) mechanism. On the other hand, when using Cu as a catalyst, the RDS changes before and after the introduction of HMF. Combining this observation with relevant literature, the researchers proposed that on Cu, the RDS involves a proton-electron coupled transfer (CPET) process, and the reaction mechanism for HMF on Cu is an electroreduction mechanism. Based on the aforementioned analysis, the electrochemical reduction processes of HMF on Ru1Cu and Cu are proposed, as shown in Figure 6E and F, respectively.
Electrocatalytic upgrading of furfural
FUR, an essential component of hemicellulose, is considered one of the most promising biomass-derived molecules for the production of various fuels and high-value-added chemicals (including furfuryl alcohol,
Zhou et al. reported highly selective ECH of FUR under neutral conditions by P-doped carbon-supported Cu SACs[70]. The controlled experiments demonstrated that the single-atom Cu site served as the active center for the reaction, facilitating the highly selective hydrogenation of FUR while inhibiting the HER. Additionally, the doping of oxyphilic P enhanced the adsorption of furfuryl alcohol, which promotes the conversion of FUR to 2-MF through furfuryl alcohol intermediates. Through experimental investigations and DFT calculations, Mukadam et al. revealed that the adsorption strength of FUR on catalytic active sites plays a crucial role in determining its product selectivity[71]. They achieved the highly selective synthesis of hydrofuroin by utilizing CuPc and CoPc with weak adsorption capabilities.
The upgrading of polyol
The polyol, such as glucose, glycerol, and ethylene glycol (EG), is widespread in the composition of biomass derivatives. Therefore, enhancing the upgrade of polyols holds significant importance in realizing the utilization of biomass resources and enhancing economic benefits [Table 2].
Various SACs for the conversion of polyol
Entry | Polyol | Catalyst | Catalytic activity | Ref. |
1 | Glycerol | Bi-Co3O4 | 97.05 ± 2.55% FE, 97.01 ± 1.73% formate selectivity | [72] |
2 | Glycerol | PtSA-NiCo LDH/NF | 85% glycerol conversion, 88.7% FE of formate | [73] |
3 | Glycerol | Pt-SA/WOx | 60.2% DHA selectivity | [74] |
4 | Glucose | Pt/def-TiO2 NRAs | 84.3% gluconic acid yield | [75] |
5 | Ethylene glycol | PtSAC/TiO2 | 99.7% FE | [76] |
6 | Ethylene glycol | Pd-N4/Cu-N4 | Glycolate selectivity (> 88%) | [77] |
Electrocatalytic and photoelectrocatalytic upgrading of glycerol and glucose
Doped spinel oxides, such as transition metal single-atom-doped Co3O4 catalysts, demonstrate enhanced glycerol oxidation reaction (GOR) activity. The strategy of doping single-atom bismuth (Bi) at the CoOh3+ sites to enhance the selectivity and activity of Co3O4 in electrocatalytic GOR was provided by Wang et al. in 2022[72]. The Co(OH)2 precursor was initially synthesized via electrodeposition, followed by ion exchange in an EG solution containing bismuth nitrate. Subsequently, the Bi-doped Co3O4 nanosheet array (Bi-Co3O4) was fabricated through a calcination process [Figure 7A]. The AC HAADF-STEM maps of various regions indicate that Bi exists as individual atoms within Bi-Co3O4 and is doped into the Oh site of Co3O4. Experimental and theoretical studies demonstrate that the substitution of Bi single atoms at CoOh3+ sites facilitates OH* generation at the adjacent CoTd2+ sites, leading to a decrease in OH* formation energy on
Figure 7. (A) The synthesis for Bi-Co3O4. (B) Formation energies of OH* and charge-transfer energies of Co3O4 and Bi-Co3O4. (C) The influence of Bi doping on the co-adsorption of OH* and glycerol. (D) LSV curves of Co3O4 and Bi-Co3O4. (E) The FE at wide potential range. (F) Stability test of Bi-Co3O4. Copyright 2022, ACS[72].
A general synthesis scheme for a single atom electrocatalyst by a simple condensing-carbonization process was reported by Chen et al. in 2020. The prepared SACs exhibited excellent performance of electrocatalytic GOR in an alkaline solution[78].
LDHs with high specific surface area and abundant defect sites are good carrier materials for the confinement of single metal atoms. In LDHs, oxygen atoms can form M-O bonds with noble metal single atoms, thereby regulating the d-electronic state of precious metal and enhancing chemisorption and activation of reactive substances. Yu et al. reported a Pt (5.64 wt%) SAC supported on a NiCo LDH for the conversion of glycerol[73]. The catalysts were prepared using electrochemical deposition, and Pt single atoms were uniformly distributed on PtSA-NiCo LDH/nickel foam (NF) [Figure 8A and B]. The electron paramagnetic resonance (EPR) spectra have confirmed that Pt increases the concentration of oxygen vacancies [Figure 8C]. The Pt single atoms were suggested to reduce the energy barrier and promote catalytic performance. According to DFT calculations, incorporating single-atom Pt doping can result in a downward shift of the d-band center [Figure 8D]. Under optimized conditions (1.375 V vs. RHE), the SAC led to an 85% glycerol conversion with an 88.7% FE of formate [Figure 8E and F] (Table 2, entry 2). A minor presence of carbonate was identified via 13C nuclear magnetic resonance (NMR), suggesting ongoing oxidation of formate to carbon dioxide [Figure 8G]. Based on this, the oxidation mechanism of glycerol was proposed [Figure 8H]. The main reaction pathway for the glycerol ECO to formic acid is as follows: glycerol is oxidized to glycerol aldehyde at the anode, which is further oxidized to glyceric acid. Subsequently, carbon-carbon bonds cleave to yield acetic acid and formic acid. Simultaneously, acetic acid can be further oxidized to produce acetaldehyde, which then undergoes carbon-carbon bond cleavage to generate two molecules of formic acid.
Figure 8. (A) Schematic illustration for the fabrication, (B)AC HAADF-STEM and EDX element mapping images of PtSA-NiCo LDH/NF. (C) EPR spectra, (D) PDOS patterns of PtSA-NiCo LDH/NF and NiCo LDH/NF. (E) The schematic illustration of HER/GOR. (F) Electrolytic performance across a wide potential range. (G) The 13C NMR spectra of CO32-, HCOOH, and the electrolyte before and after the reaction, (H) Schematic diagram of glycerol oxidation mechanism. Copyright 2023, Elsevier[73].
Photoelectric chemical (PEC) biomass conversion is considered as a promising technology that can transform renewable energy into high-value-added products. Among many conversion reactions, the oxidation of glycerol by PEC to dihydroxyacetone (DHA) is considered to be a promising method. A WO3 amorphous/crystalline homojunction photoanode doped with Pt single atoms (Pt-SA/WOx) has been successfully constructed by Feng et al. in 2024, showing excellent GOR performance in acidic solution, with 60.2% selectivity of DHA at 1.2 V vs. RHE (Table 2, entry 3)[74]. In addition, experimental and DFT calculations show that the synergistic effect of vacancies and single-atomic doping can accelerate the charge transfer rate, prolong the carrier lifetime, and reduce the GOR kinetic barrier, thereby improving the activity of GOR and selectivity of DHA. Ensuring the sustainability of photoanodes is essential for practical applications of photoelectrocatalysis. While the photocurrent density of WO3 decreases rapidly to 39.9% of its initial value after five hours of PEC processing, indicating inadequate stability, the photocurrent densities of WOx and Pt-SA/WOx remain at 59.0% and 68.6% of their initial values, respectively. This demonstrates that incorporating amorphous/crystalline homojunction and Pt single-atom sites benefits photoanode stability.
In 2023, Tian et al. reported a photoelectrochemical method that employs a defective TiO2 nanoarray for the immobilization of a single-atom platinum (Pt/def-TiO2 NRAs) as a photoanode, enabling selective oxidation of glucose and its conversion into high-value-added gluconic acid[75]. The defect structure caused by oxygen vacancy can regulate both carrier dynamics and band structure. After the optimization of oxygen vacancy, the charge separation rate of Pt/def-TiO2 NRAs significantly improved the selectivity and yield of C6 products, and realized the selective oxidation of glucose to gluconic acid. In this study, the optical flux density of glucose oxidation by Pt/def-TiO2 NRAs at 0.6 V was 1.91 mA cm-2, and the yield of gluconic acid under simulated sunlight irradiation was 84.3% (Table 2, entry 4). In the electrooxidation of glucose, the stability of Pt/def-TiO2 photoanodes was investigated through five cycles of J-t experiments. The electrolyte was replaced every 5.5 h, and after 27.5 h of testing, no significant decay of photocurrent densities for glucose was observed, indicating excellent stability of the Pt/def-TiO2 photoanode.
Electrocatalytic upgrading of ethylene glycol
EG is a diol produced by the hydrogenation of cellulose. Due to its non-toxic, higher energy density, high boiling point, and good reactivity, it is considered a promising renewable material for the production of value-added chemicals such as formates and glycolates.
Ayele et al. pioneered the synthesis of Pt single atoms supported on titanium oxide (PtSAC/TiO2) for EG oxidation (EGO)[76]. PtSAC/TiO2 and PtNP/TiO2 catalysts were synthesized using a simple, rapid, economical hydrothermal-assisted co-precipitation method. The extended XAFS (EXAFS) analysis provides evidence of Pt single atoms [Figure 9A]. In CV tests, PtSAC/TiO2 exhibits a high ratio of Jf/Jb, suggesting either reduced adsorption of reaction intermediates of EGO or the synergistic effect of the TiO2 support in aiding the removal of reaction intermediates from the active sites [Figure 9B]. The PtSAC/TiO2 catalyst exhibits an outstanding total FE of 99.7% at 0.7 V vs. RHE (Table 2, entry 5), which is higher than that of PtNP/TiO2. The PtSAC/TiO2 enhances the electronic interaction between Pt and TiO2, thereby contributing to improved stability, as evidenced by a minimal decrease in current drop within a 12-h timeframe in an alkaline solution. Subsequently, the same group prepared a Pt SAC supported on Ni-doped TiO2, which enables the partial oxidation of EG by Ni doping[79]. The catalyst containing Ni establishes an environment that facilitates enhanced OH- adsorption, enabling increased accessibility of Pt for subsequent oxidations. Additionally, the catalyst exhibits exceptional FE (> 98%) and stability in the alkaline environment during the EGO. The catalyst exhibits enhanced electrocatalytic performance in the oxidation of EG, attributed to the synergistic effects of Ni and Pt, as depicted in Figure 9C and D. The strong interactions between the substrate and the catalyst prevent the aggregation of single atoms, thereby improving stability. Incorporating secondary metal atoms has the potential to enhance the activity of SACs. It is logical to anticipate that the interplay of the electronic structures of two metal elements will lead to a more optimized configuration, facilitating the attainment of an appropriate state for the adsorption and desorption of intermediate reactions. Consequently, dual-site SACs (DSACs) empower diatomic sites to exhibit superior catalytic activity compared to their isolated single.
In 2022, Moges et al. utilized copper phthalocyanine as the precursor and successfully synthesized DSACs Pd-N4/Cu-N4 through a simple, economical, high metal loading, and efficient electrochemical reduction
Figure 10. (A) The synthesis of Pd-N4/Cu-N4 by electrochemical reduction. (B) The EDX mapping images of C, N, Pd, and Cu. Aberration-Corrected HAADF-STEM images of Pd-N4/Cu-N4 showing the distribution of (C) Pd and (D) Cu. (E) Pd 3d for Pd-N4/Cu-N4 and Pd(OAc)2; (F) Cu 2p for CuPc and Pd-N4/Cu-N4. Copyright 2020, Wiley[77].
Because of the higher atomic number of Pd compared to Cu, Pd in the HAADF-STEM image displays a greater number of bright spots than Cu [Figure 10C and D]. Fourier-transformed EXAFS (FT-EXAFS) analysis showed that no Pd-Pd bonds were observed in the Pd-N4/Cu-N4 catalyst. These results serve to validate the existence of Pd and Cu in a singular atomic form, evenly dispersed across the exposed surface of the electrode. Furthermore, according to the X-ray absorption spectroscopy (XAS) and XPS analyses, the oxidation state of Pd in Pd-N4/Cu-N4 was determined to be +2. The findings demonstrate that Cu within Pd-N4/Cu-N4 exists in both +1 and +2 oxidation states [Figure 10E and F]. In alkaline media, the mechanism of EGO on DSAC Pd-N4/Cu-N4 electrocatalysts can be delineated into two consecutive steps: initially, EGO yields glycolate, followed by its further oxidation to formate. Remarkably, glycolate was identified as the primary product of EGO on the Pd-N4/Cu-N4 electrocatalyst. This is attributed to the synergistic effect of Pd and Cu single atoms, facilitating EGO in alkaline environments. Surface Cu species are enveloped by OH- ions, facilitating electron transfer to Pd atoms. The electron-rich Pd atoms, in turn, enhance the catalytic ability of Pd species to suppress C-C bond cleavage. Outperforming various palladium-based electrocatalysts reported in the literature, the Pd-N4/Cu-N4 electrocatalyst demonstrates significantly higher glycolate selectivity (> 88%) (Table 2, entry 6).
The upgrading of ethanol
Bioethanol is a form of renewable energy generated through the fermentation of biomass or agricultural waste. In recent years, there has been widespread attention directed towards the ethanol oxidation reaction (EOR), propelled by the significance of bioethanol and its integral role in addressing energy demands and environmental cleanliness. Its applications span various sectors, including but not limited to fuel, fuel additives, and disinfectants. Direct ethanol fuel cells (DEFCs) have the prospect of large-scale application due to their advantages (e.g., relatively convenient transportation and storage, security) of ethanol. EOR can proceed via two parallel pathways: complete oxidation and partial oxidation. The complete electrooxidation of ethanol (C1 pathway) with the formation of CO2 by breaking the C-C bond and the product of incomplete oxidation (C2 pathway) is acetate. CH3CHO is a typical intermediate of ethanol oxidation. However, the low selectivity of the C1 pathway restricts the efficiency of ethanol's complete oxidation. Developing efficient and durable electrocatalysts is crucial for the commercial viability of DEFCs. While noble metals, such as Pt and Pd, demonstrate exceptional performance in ethanol electrooxidation, their high cost, limited availability, and susceptibility to CO poisoning hinder widespread deployment. SACs, as highly efficient catalysts with nearly 100% atomic utilization, have been widely applied in bioethanol electrooxidation. Furthermore, SAA catalysts have been engineered to enhance stability.
In 2016, Liu et al. reported on how to precisely manipulate and control the atomic geometry of Pd shell atoms on Au nanowires, thereby achieving the formation of isolated and continuous Pd atoms on the surface of these nanowires[80]. However, various results lead to the same conclusion that Pd ensembles, rather than single Pd atoms, serve as the active sites for EOR. In 2020, Tiwari et al. reported that Pd-Co2P-PdSAs@GO (palladium-cobalt phosphide NPs with Pd single atoms anchored on graphene oxide) catalysts showed high activity in EOR, with a mass activity (MA) of 10,520 mA mgPd-1, which is remarkably more significant than Pt/C[81]. Pd-Co2P-PdSAs@GO exhibits high catalytic activities, C1 pathway selectivity and stability in alkaline electrolytes. The promotion of catalyst activities is attributed to charge transfer between Pd single atoms and Co2P to shift the Pd d-band center, and the distinctive electronic configuration of
Surface modification strategies of noble metal nanocatalysts can adjust the local electronic structure to enhance EOR activity, but usually at the expense of the electrochemically active surface area (ECSA). Hence, the spotlight increasingly falls on SACs. The rapid oxidative removal of CO species at noble metal sites is crucial for enhancing ethanol oxidation activity. Reportedly, Ni SACs provide a substantial quantity of adsorbed OH species, which can facilitate the oxidative elimination of CO at noble metal sites. Therefore, dispersing Ni single atoms onto noble metal NPs may improve the performance of EOR. Single Ni atom-modified Pt nanowires (SANi-PtNWs) developed by Li et al. exhibited superior EOR and methanol oxidation reaction (MOR) activity[82]. The author used inductively coupled plasma atomic emission spectroscopy (ICP-AES) to prove that there should be 2.4 Ni atoms and 15 Pt atoms per square nanometer pair; that is, the ratio of Ni and Pt atoms on the surface is about 1:6. Then, by means of EXAFS and XANES tests in synchrotron radiation, it is shown that Ni atoms are dispersed on the surface of Pt, and the average oxidation state of Ni close to two, consistent with the XPS studies. The MA of SANi-PtNWs
Strain engineering can regulate catalytic performance by adjusting the catalyst's electronic structure and intermediates’ adsorption energy on the surface. The synergistic effect between tensile strain and the influence of ligands can enhance the activity of EOR. It has been reported that applying tensile strain to Pt increases its surface reactivity towards EOR. Zhang et al. designed and synthesized core-shell PtBi@Pt catalyst with Pt subjected to tensile rather than compressive strain, thereby enhancing EOR activity[84]. Importantly, in situ fourier transform infrared spectroscopy (FTIR) analysis reveals that PtBi/SA Pt and
Figure 11. (A) Schematic illustration of the preparation procedure of PtBi-3.6% Rh1 and PtBi@PtRh1 nanoplates. Copyright 2021, Wiley[85]. (B) FECH3COOH/FEC1 and FEC1/FEC2. (C) CO-stripping curves of the three catalysts. Copyright 2022, Wiley[86]. Reaction energy barriers for (E) C-C bond cleavage of CH3CO* and (F) CO* oxidation. Copyright 2024, Elsevier[87].
In 2022, Zhang et al. reported a strategy of constructing single-atom Ir-tensile-strained Pt (100) catalyst at heterophase hcp-PtPb/fcc-Pt core–shell hexagonal nanoplates (PtPb@PtIr1 HNP/C) to enhance the activity and selectivity of EOR[86]. The noble metal Ir facilitates C-C bond cleavage in EOR; thus, single-atom Ir sites can be used to enhance the selectivity of the C1 pathway to promote EOR activity. In Figure 11B, it is evident that the PtPb@PtIr1 hexagonal nanoplates (HNPs) exhibit a higher selectivity and FE (57.93%) in the complete oxidation of ethanol, showcasing the ability of Ir1 sites to enhance the selectivity of the C1 pathway and suppress the formation of acetic acid in EOR. Additionally, the lower onset oxidation potential and higher current density of PtPb@PtIr1 HNP/C indicate that the EOR activity can be enhanced by incorporating Ir1 sites. CO-stripping curves suggest that the key sites for the oxidation of COads intermediates are the Pt(110) surface rather than Ir1 [Figure 11C]. Meanwhile, the tolerance of CO intermediates of PtPd@PtIr1 HNPs is superior to that of Pt/C.
Zhang et al. found that hydrogen implantation can induce tensile strain and cause the D-band center of Pd to downshift[87]. Experiments and DFT calculations indicate that H-implantation can enhance the p-d orbital hybrid interaction between Ga and Pd, thereby significantly promoting the C-C bond cleavage and CO* oxidation in the C1 pathway, showing excellent EOR activity and C1 pathway selectivity
The Rh-based catalyst has been verified to effectively catalyze the conversion of ethanol into CO2 via the C1 pathway[88,89]. Although PtBi@PtRh1 catalyst synthesized by Luo et al. can promote C-C bond breaking, the C1 pathway is not highly selective[85]. In 2022, Chang et al. utilized a two-step solution method to synthesize partially oxidized single Rh dispersed on the (100) surface of Pt nanocubes (RhatO-Pt NCs), which shows an unprecedentedly high CO2 selectivity with low CO2 generation potential compared to other Pt-based electrocatalysts in the EOR literature[90]. Experiment and DFT calculations demonstrated that the Rh decoration remarkably improved the CO2 selectivity in EOR, but the role of Rh and Pt in improving the performance of EOR is different, with Rh promoting the cleavage of C-C bond to form *CH3 + *CO intermediates and the removal of *CO intermediates, while Pt as the active center of the overall EOR activity [Figure 12A and B]. The RhatO-Pt NCs/C catalyst has a strong ability to break the ethanol C-C bond in the range of 0.35 to 0.75 V vs. RHE, and the selectivity of CO2 reaches 99.9% [Figure 12C].
Figure 12. (A) Anodic polarization curves for electrocatalysts. (B) DFT-calculated free energy profile of C-C bond cleavage of
Pei et al. prepared a multimetallic catalyst, where Pd nanoclusters and Ru single atoms were anchored onto defect sites of Ni(OH)2[91]. The interaction between neighboring Ru and Pd, along with the adsorption of OHad by Ni(OH)2 at low potential, results in the reduction of the reaction barrier for eliminating reactant intermediates and improves the selectivity of the C1 pathway in the EOR process. Wang et al. developed an efficient PdBi SAA catalyst (ionic liquid (IL)/Pd50Bi1) by manipulating noble metal aerogel at the atomic scale, utilizing a one-step NaBH4 reduction method, and applied it in EOR[92]. The introduction of Bi single atoms increased the catalyst's surface area, providing more abundant active sites, accelerating electron and mass transfer rates, and enhancing ethanol oxidation activity. Due to the introduction of single atom Bi and the interface engineering between the surface and IL, the IL/Pd50Bi1 aerogel demonstrated excellent ethanol oxidation performance. The MA of IL/Pd50Bi1 aerogel was 4.1 times higher than that of Pd/C catalyst. Furthermore, the IL/Pd50Bi1 aerogel has superior performance compared to currently reported Pd-based nanomaterials, exhibited excellent stability, higher CO tolerance, and faster charge transfer efficiency. NMR analysis of the electrolyte before and after ethanol oxidation electrolysis revealed that Bi significantly improved the selectivity toward the C2 pathway [Figure 12D]. DFT calculations also confirmed the reduction of the energy barrier of the RDS with the introduction of Bi single atoms, thereby accelerating the reaction kinetics of EOR [Figure 12E].
The upgrading of methanol
Methanol, the simplest primary alcohol, can be derived from biomass and has increasingly emerged as a favored chemical feedstock due to its wide availability and low cost. Although Pt-based catalysts demonstrate remarkable catalytic activity in the MOR, their high cost, limited availability, and susceptibility to CO poisoning hinder their widespread application in MOR. Therefore, the utilization of single-atom Pt catalysts to reduce Pt usage, along with the development of efficient, CO-tolerant Pt-based catalysts, holds significant importance for enhancing their durability and MOR activity. However, researchers have observed that the CO pathway for methanol oxidation cannot be facilitated on single Pt atoms due to the strong binding of CO intermediates to Pt sites. It has been reported that Pt SACs supported on carbon black and carbon nanotubes (CNTs) exhibit negligible activity towards MOR.
Zhang et al. employed a facile adsorption-impregnation method to prepare two types of Pt SACs, Pt1/RuO2 and Pt1/VXC-72, using RuO2 and carbon black as substrates, respectively[93]. Pt1/RuO2 SACs exhibited excellent MA, remarkable catalytic stability, and high tolerance to CO poisoning. However, the single-atom Pt on carbon black showed inappreciable MOR activity, consistent with literature reports. Experimental and DFT calculations showed that the coordination environment of Pt single atoms changed with RuO2 substrate, enhancing both the stability and MOR activity of Pt1/RuO2 through facilitated electrochemical CO removal. In 2022, Poerwoprajitno et al. synthesized single-Pt-atom-on-Ru catalysts, with individual Pt atoms supported on low-index facets of Ru NPs, for MOR[94]. The strong Pt-Ru bond is a key factor in maintaining structural stability during catalysis. Additionally, DFT calculations demonstrated that the catalyst exhibits strong methanol adsorption and weak CO binding capability, thus favorably augmenting MOR activity.
Chen et al. developed a high-performance Pt-based SAA catalyst (Ru1Ptn-SAA) for MOR through the dual modulation of H2 and NO3-[95]. Control experiments indicated that Ru1Ptn-SAA could not be successfully synthesized in the absence of either NO3- or H2. XAFS and XPS results showed that Pt exists in a metallic state, with electrons transferring from Ru to Pt, and the absence of Ru-Ru bonds confirmed the singular atomic form of Ru within Ru1Ptn-SAA. DFT calculations demonstrated that the introduction of Ru into Pt NPs decreases the reaction free energy of the RDS (CO* + OH*) in MOR. This facilitates easier activation of water molecules on the surface of Ru1Ptn-SAA and reduces the binding energy of Pt with CO, making CO desorption easier and enhancing MOR activity. Kong et al. prepared Ru-ca-PtNi catalysts for OER and unveiled a single-atom-cavity coupling mechanism, which enhances the activity and stability of SACs[96]. The MA of Ru-ca-PtNi was 5.8 times higher than Pt/C. Electrochemical in situ IR spectroscopy reveals that Ru single atoms anchored to vacancies can accelerate the desorption of CO. Experimental and DFT calculations indicate that single atom-vacancy coupling can regulate the electronic structure of Pt and optimize the position of the d-band center to promote the MOR. Zhuang et al. synthesized a highly efficient catalyst for methanol oxidation, denoted as PdNPs/Pd-Nx@C, by integrating Pd single atoms and NPs on nitrogen-doped carbon (N-C) substrates, which demonstrated superior MOR activity and stability[97]. Analysis of the ratio between forward and backward peak current densities, along with the CO stripping voltammogram, suggested that CO was more effectively oxidized and removed on the PdNPs/Pd-Nx@C catalyst. Experimental investigations and DFT calculations revealed that charge transfer is identified as pivotal in influencing the binding strength between active sites and CO intermediates. Specifically, the preferential electron transfer from Pd single atoms to the support hindered the charge transfer between Pd NPs and the support, thus enhancing the MOR activity and stability of the catalyst. Ruan et al. introduced Pt NPs onto N-C-supported Co single atoms via a two-step method, where the synergistic effect between
In addition, the single-atom modification of Pt and Pd becomes a highly efficient strategy to improve catalytic performance, triggering the largest number of active sites. Through etching and annealing treatments, Fan et al. harnessed the inherently isolated Bi atoms within Pt-Bi intermetallic compounds to fabricate surface-enriched single Bi atom-modulated Pt nanorods for MOR[100]. The MA of SE-Bi1/Pt NRs achieved 23.77 A mgPt-1, surpassing that of commercial Pt/C by 12.8 times. Experimental and DFT calculations corroborate that surface-enriched Bi single atoms facilitated the CO-free pathway and expedited the kinetics of *HCOOH formation. Wei et al. found that doping Ir single atoms onto Pd NPs can enhance CO tolerance and increase MOR activity and stability[101]. Electrochemical tests showed that the catalyst exhibits a MOR MA of approximately 1,717.3 mA mgPd-1. DFT calculations demonstrate that Ir single atoms alter the electronic structure of Pd NPs, facilitating electron transfer from Pd to Ir, thus contributing to the superior performance observed.
SACs FOR THE UPGRADING OF LIGNIN
Lignin, the most abundant aromatic polymer in nature, is a rich source of renewable energy due to its high calorific value and aromatic content. It is a complex cross-linked macromolecule composed of numerous aromatic rings interconnected by C-O-C and C-C bonds, exhibiting a complex structure and low reactivity. Lignin can be processed to produce various high-value fuels, chemical feedstocks, and pharmaceutical intermediates. In recent years, lignin conversion has emerged as a forefront research area worldwide. Among various lignin conversion technologies, traditional thermochemical treatments have been the most mature and extensively studied. However, their harsh reaction conditions limit their large-scale application and hinder green and sustainable production. With the advancement of green chemistry, electrocatalytic lignin conversion has gained considerable attention due to its mild reaction conditions, offering new green catalytic strategies for lignin conversion research.
The key to realizing the lignin valorization to harvest high-value-added aromatic compounds is selective cleavage of C-C bonds. Miao et al. prepared Ir-NiFeO@NF catalyst via hydrothermal and impregnation methods, which realized the upgrading of lignocellulose and energy-saving hydrogen production[102]. When 1-phenylethanol was used as a substrate, benzoic acid was obtained with a yield of 91.4%. DFT calculations suggest that individual Ir atoms promote the adsorption of reactants and the generation of OH* to facilitate the cleavage of C(O-)C bonds. Cui et al. successfully prepared a nitrogen-doped layered porous CNT carrier using the copolymerization reaction of aniline and pyrrole, and carbonization, and further impregnated in chloroplatinic acid to obtain Pt1/N-CNTs catalyst[103]. Characterization through EDX-mapping, aberration-corrected STEM (AC-STEM), and EXAFS revealed that Pt exists in a single-atom dispersed state. EXAFS spectrum fitting indicated that the central atom adopts a Pt-N4 coordination structure. At room temperature, the yield of benzaldehyde reached up to 81% in the ECO cleavage reaction of 2-Phenoxy-1-phenylethanol, significantly higher than commercial Pt/C. A series of control experiments, including radical trapping and isotope labeling experiments, demonstrated that the reaction mechanism of the Pt1/N-CNTs catalyst differs from that of traditional catalysts. The reaction proceeds through a radical pathway, with the RDS being Cβ-H abstraction. The exceptional activity of Pt1/N-CNTs stems from the Pt-N3C1 sites, which facilitate the formation of crucial Cβ radical intermediates. These enable the selective cleavage of the Cα-Cβ bond to obtain benzaldehyde. Through systematic experimental studies and DFT calculations, two possible reaction mechanisms were proposed by the authors [Figure 13A]. Initially, tert-butyl hydroperoxide (TBHP) is oxidized at the anode, resulting in the generation of tert-butyl radical (A1·, Pathway 1) and tert-butyl peroxide radical (A2·, Pathway 2). Subsequently, radical intermediate B· is generated from 2-Phenoxy-1-phenylethanol. Intermediates C1 and C2 are then formed through radical/radical cross-coupling. Finally, the selective cleavage of the Cα-Cβ bond yields benzaldehyde, CO2, and other products. Recently, ECH has garnered considerable attention due to its environmentally benign and mild approach. The key factor to improve the FE is the modulation of substrate and hydrogen adsorption energy on the cathodic catalyst surface to prevent the occurrence of HER. Tong et al. reported an electrocatalyst comprising Pt single atoms supported on a CoO/Co heterostructure (Pt1-CoO/Co), investigating the ECH performance of lignin derivatives using phenol as a model substrate[104]. DFT calculations and experimental studies demonstrated that the synergistic effect of Pt1-CoO/Co improves the ECH activity of phenol. The Pt atoms anchored around metallic Co facilitate proton reduction and adsorption, while CoO sites adsorb and activate phenol. Subsequently, hydrogen adsorbed on the single Pt atoms is transferred to the activated phenol, enabling the efficient ECH of phenol to yield high-value KA oil while simultaneously inhibiting the HER [Figure 13B].
CONCLUSIONS AND PERSPECTIVE
Biomass, a crucial component of renewable energy, plays a significant role in addressing energy and environmental challenges, thus attracting considerable attention for its conversion. Electrocatalysis and photoelectrocatalysis, as novel catalytic approaches, have been widely employed to achieve the conversion of various biomass-based compounds. With high catalytic activity, product selectivity, and excellent stability, SACs present broad prospects in the catalytic conversion of biomass. At present, SACs have been used in converting lignin, biomass-derived aldehydes, and alcohols, which drives the biomass valorization to high-value-added chemicals and plays an important role in promoting industrial synthesis. In biomass valorization, single-atom photoelectrocatalysts and electrocatalytic technologies demonstrate significant potential. With high-efficiency photoelectrocatalytic activity and selectivity, single-atom photoelectrocatalysts effectively promote biomass photocatalytic conversion processes such as degradation and synthesis. Simultaneously, electrocatalytic techniques leverage exceptional electrochemical activity and controllability to achieve biomass electrochemical transformations, including HMFOR and EOR, thereby reducing energy consumption in HER and facilitating the production of high-value chemicals at the anode. Single-atom catalysis within these advanced technologies offers a novel pathway for achieving sustainable biomass utilization, promising to drive future developments in sustainable energy and chemical production. However, challenges persist despite significant advancements in SACs for biomass electrocatalytic conversion. Future research directions can be categorized as follows: In SAC design, strategies such as doping, surface functionalization, nanostructuring, heteroatom substitution, and Atomic Layer Deposition (ALD) play a crucial role in modifying local environments of single-atomic sites to enhance catalytic efficiency and specificity. These methods collectively contribute to refining the local environments of single atomic sites for improved catalytic outcomes. Metal-organic frameworks (MOFs) present favorable attributes such as high surface area and adjustable pore structures, positioning them as promising choices for facilitating single atomic sites in catalysis. Carbon Nanotubes (CNTs) and Graphene, as carbon-based materials, exhibit distinctive electronic properties and expansive surface areas, suggesting their capacity to effectively stabilize and modify the local environment of single atomic sites. LDHs showcase diverse compositions and surface functionalities, creating opportunities to tailor the surroundings of single atomic sites, potentially enhancing catalytic activity. Meanwhile, Transition Metal Carbides/Nitrides (MXenes) demonstrate high conductivity and modifiable surface chemistry, indicating their potential as support materials for single atomic sites across various catalytic applications. Particularly for larger molecules such as lignin derivatives, coupling structures of single atoms with NPs can be designed to achieve selective conversion.
Although SACs have been applied in the electrocatalytic and photo-electrocatalytic conversion of biomass-based compounds, their practicality remains limited. Future research will focus on developing multifunctional SACs capable of simultaneously catalyzing multiple biomass conversion reactions, such as hydrogenation, deoxygenation, and carbonylation, to achieve efficient, comprehensive biomass conversion. Additionally, achieving further breakthroughs based on the conversion of model compounds and directly realizing highly selective conversion of structurally complex cellulose, hemicellulose, and lignin is an important direction for future research.
DECLARATIONS
Authors’ contributions
Conceptual design and project supervision: Cui T, Xu M, Hou X
Manuscript draft and revision: Ma J, Yan H, Xu M, Cui T
Availability of data and materials
Not applicable.
Financial support and sponsorship
This work was supported by the National Natural Science Foundation of China (No. 22379023, No. 22102007) and the China Postdoctoral Science Foundation (No. 2023M740555).
Conflicts of interest
All authors declared that there are no conflicts of interest.
Ethical approval and consent to participate
Not applicable.
Consent for publication
Not applicable.
Copyright
© The Author(s) 2025.
REFERENCES
1. Nanda, S.; Mohanty, P.; Pant, K. K.; Naik, S.; Kozinski, J. A.; Dalai, A. K. Characterization of North American lignocellulosic biomass and biochars in terms of their candidacy for alternate renewable fuels. Bioenerg. Res. 2013, 6, 663-77.
2. Taarning, E.; Osmundsen, C. M.; Yang, X.; Voss, B.; Andersen, S. I.; Christensen, C. H. Zeolite-catalyzed biomass conversion to fuels and chemicals. Energy. Environ. Sci. 2011, 4, 793-804.
3. Kavitha, S.; Yukesh, K. R.; Kasthuri, S.; et al. Profitable biomethane production from delignified rice straw biomass: the effect of lignin, energy and economic analysis. Green. Chem. 2020, 22, 8024-35.
4. Deng, W.; Feng, Y.; Fu, J.; et al. Catalytic conversion of lignocellulosic biomass into chemicals and fuels. Green. Energy. Environ. 2023, 8, 10-114.
5. Madadi, M.; Elsayed, M.; Sun, F.; et al. Sustainable lignocellulose fractionation by integrating p-toluenesulfonic acid/pentanol pretreatment with mannitol for efficient production of glucose, native-like lignin, and furfural. Bioresour. Technol. 2023, 371, 128591.
6. Li, X.; Xu, R.; Yang, J.; et al. Production of 5-hydroxymethylfurfural and levulinic acid from lignocellulosic biomass and catalytic upgradation. Ind. Crop. Prod. 2019, 130, 184-97.
7. Wang, T.; Nolte, M. W.; Shanks, B. H. Catalytic dehydration of C6 carbohydrates for the production of hydroxymethylfurfural (HMF) as a versatile platform chemical. Green. Chem. 2014, 16, 548-72.
8. Gürbüz, E. I.; Gallo, J. M.; Alonso, D. M.; Wettstein, S. G.; Lim, W. Y.; Dumesic, J. A. Conversion of hemicellulose into furfural using solid acid catalysts in γ-valerolactone. Angew. Chem. Int. Ed. 2013, 52, 1270-4.
9. Devi, A.; Bajar, S.; Kour, H.; Kothari, R.; Pant, D.; Singh, A. Lignocellulosic biomass valorization for bioethanol production: a circular bioeconomy approach. Bioenergy. Res. 2022, 15, 1820-41.
10. Wang, M.; Liu, M.; Lu, J.; Wang, F. Photo splitting of bio-polyols and sugars to methanol and syngas. Nat. Commun. 2020, 11, 1083.
11. Yoo, C. G.; Meng, X.; Pu, Y.; Ragauskas, A. J. The critical role of lignin in lignocellulosic biomass conversion and recent pretreatment strategies: a comprehensive review. Bioresour. Technol. 2020, 301, 122784.
12. Garedew, M.; Young-farhat, D.; Jackson, J. E.; Saffron, C. M. Electrocatalytic upgrading of phenolic compounds observed after lignin pyrolysis. ACS. Sustain. Chem. Eng. 2019, 7, 8375-86.
13. Meng, Q.; Hou, M.; Liu, H.; Song, J.; Han, B. Synthesis of ketones from biomass-derived feedstock. Nat. Commun. 2017, 8, 14190.
14. Zhou, H.; Li, Z.; Xu, S. M.; et al. Selectively upgrading lignin derivatives to carboxylates through electrochemical oxidative C(OH)-C bond cleavage by a Mn-doped cobalt oxyhydroxide catalyst. Angew. Chem. Int. Ed. 2021, 60, 8976-82.
15. Kumar, R.; Strezov, V. Thermochemical production of bio-oil: a review of downstream processing technologies for bio-oil upgrading, production of hydrogen and high value-added products. Renew. Sustain. Energy. Rev. 2021, 135, 110152.
16. Pang, S. Advances in thermochemical conversion of woody biomass to energy, fuels and chemicals. Biotechnol. Adv. 2019, 37, 589-97.
17. Liu, W.; Cui, Y.; Du, X.; Zhang, Z.; Chao, Z.; Deng, Y. High efficiency hydrogen evolution from native biomass electrolysis. Energy. Environ. Sci. 2016, 9, 467-72.
18. Du, X.; Liu, W.; Zhang, Z.; et al. Low-energy catalytic electrolysis for simultaneous hydrogen evolution and lignin depolymerization. ChemSusChem 2017, 10, 847-54.
19. Tang, W.; Zhang, L.; Qiu, T.; et al. Efficient conversion of biomass to formic acid coupled with low energy consumption hydrogen production from water electrolysis. Angew. Chem. Int. Ed. 2023, 62, e202305843.
20. Zhang, X.; Wilson, K.; Lee, A. F. Heterogeneously catalyzed hydrothermal processing of C5-C6 sugars. Chem. Rev. 2016, 116, 12328-68.
21. Zhang, B.; Yang, Z.; Yan, C.; Xue, Z.; Mu, T. Operando forming of lattice vacancy defect in ultrathin crumpled nivw-layered metal hydroxides nanosheets for valorization of biomass. Small 2023, 19, e2207236.
22. Li, S.; Wang, S.; Wang, Y.; et al. Doped Mn enhanced NiS electrooxidation performance of HMF into FDCA at industrial-level current density. Adv. Funct. Mater. 2023, 33, 2214488.
23. Cao, Y.; Zhang, H.; Ji, S.; et al. Adsorption site regulation to guide atomic design of ni-ga catalysts for acetylene semi-hydrogenation. Angew. Chem. Int. Ed. 2020, 59, 11647-52.
24. Rao, P.; Deng, Y.; Fan, W.; et al. Movable type printing method to synthesize high-entropy single-atom catalysts. Nat. Commun. 2022, 13, 5071.
25. Zhao, H.; Zhu, L.; Yin, J.; et al. Stabilizing lattice oxygen through Mn doping in NiCo2O4-δ spinel electrocatalysts for efficient and durable acid oxygen evolution. Angew. Chem. Int. Ed. 2024, 63, e202402171.
26. Ye, Y.; Xu, J.; Li, X.; et al. Orbital occupancy modulation to optimize intermediate absorption for efficient electrocatalysts in water electrolysis and zinc-ethanol-air battery. Adv. Mater. 2024, 36, e2312618.
27. Wang, D.; Li, Q.; Han, C.; Lu, Q.; Xing, Z.; Yang, X. Atomic and electronic modulation of self-supported nickel-vanadium layered double hydroxide to accelerate water splitting kinetics. Nat. Commun. 2019, 10, 3899.
28. Liu, S.; Gao, M.; Wu, S.; et al. A coupled electrocatalytic system with reduced energy input for CO2 reduction and biomass valorization. Energy. Environ. Sci. 2023, 16, 5305-14.
29. Wu, X.; Zhao, Z.; Shi, X.; et al. Multi-site catalysis of high-entropy hydroxides for sustainable electrooxidation of glucose to glucaric acid. Energy. Environ. Sci. 2024, 17, 3042-51.
30. Wu, Y.; Ma, L.; Wu, J.; Song, M.; Wang, C.; Lu, J. High-surface area mesoporous Sc2O3 with abundant oxygen vacancies as new and advanced electrocatalyst for electrochemical biomass valorization. Adv. Mater. 2024, 36, e2311698.
31. Zheng, X.; Yang, J.; Li, P.; et al. Ir-Sn pair-site triggers key oxygen radical intermediate for efficient acidic water oxidation. Sci. Adv. 2023, 9, eadi8025.
32. Yang, J.; Yang, Z.; Li, J.; et al. Engineering a hollow bowl-like porous carbon-confined Ru-MgO hetero-structured nanopair as a high-performance catalyst for ammonia borane hydrolysis. Mater. Horiz. 2024, 11, 2032-40.
33. Liu, W. J.; Xu, Z.; Zhao, D.; et al. Efficient electrochemical production of glucaric acid and H2 via glucose electrolysis. Nat. Commun. 2020, 11, 265.
34. Liu, C.; Shi, X. R.; Yue, K.; et al. S-species-evoked high-valence Ni2+δ of the evolved β-Ni(OH)2 electrode for selective oxidation of 5-hydroxymethylfurfural. Adv. Mater. 2023, 35, e2211177.
35. Zhou, P.; Zhang, Q.; Chao, Y.; et al. Partially reduced Pd single atoms on CdS nanorods enable photocatalytic reforming of ethanol into high value-added multicarbon compound. Chem 2021, 7, 1033-49.
36. Zhou, P.; Chao, Y.; Lv, F.; et al. Metal single atom strategy greatly boosts photocatalytic methyl activation and C-C coupling for the coproduction of high-value-added multicarbon compounds and hydrogen. ACS. Catal. 2020, 10, 9109-14.
37. Yang, W.; Jia, Z.; Zhou, B.; et al. Why is C-C coupling in CO 2 reduction still difficult on dual-atom electrocatalysts? ACS. Catal. 2023, 13, 9695-705.
38. Zhang, Z.; Wang, J.; Ge, X.; et al. Mixed plastics wastes upcycling with high-stability single-atom Ru catalyst. J. Am. Chem. Soc. 2023, 145, 22836-44.
39. Liu, J.; Lucci, F. R.; Yang, M.; et al. Tackling CO poisoning with single-atom alloy catalysts. J. Am. Chem. Soc. 2016, 138, 6396-9.
40. Xia, J.; Wang, B.; Di, J.; et al. Construction of single-atom catalysts for electro-, photo- and photoelectro-catalytic applications: State-of-the-art, opportunities, and challenges. Mater. Today. 2022, 53, 217-37.
41. Nakaya, Y.; Hirayama, J.; Yamazoe, S.; Shimizu, K. I.; Furukawa, S. Single-atom Pt in intermetallics as an ultrastable and selective catalyst for propane dehydrogenation. Nat. Commun. 2020, 11, 2838.
42. Cao, L.; Luo, Q.; Liu, W.; et al. Identification of single-atom active sites in carbon-based cobalt catalysts during electrocatalytic hydrogen evolution. Nat. Catal. 2019, 2, 134-41.
43. Vasconcelos, S. C.; Marchini, L.; Lima, C. G. S.; et al. Single-atom catalysts for the upgrading of biomass-derived molecules: an overview of their preparation, properties and applications. Green. Chem. 2022, 24, 2722-51.
44. Gan, T.; Wang, D. Atomically dispersed materials: Ideal catalysts in atomic era. Nano. Res. 2024, 17, 18-38.
45. Cui, T.; Li, L.; Ye, C.; et al. Heterogeneous single atom environmental catalysis: fundamentals, applications, and opportunities. Adv. Funct. Mater. 2022, 32, 2108381.
46. Wang, L.; Wang, L.; Zhang, L.; Liu, H.; Yang, J. Perspective of p-block single-atom catalysts for electrocatalysis. Trends. Chem. 2022, 4, 1135-48.
47. Qi, H.; Yang, J.; Liu, F.; et al. Highly selective and robust single-atom catalyst Ru1/NC for reductive amination of aldehydes/ketones. Nat. Commun. 2021, 12, 3295.
48. Liu, Z.; Li, H.; Gao, X.; et al. Rational highly dispersed ruthenium for reductive catalytic fractionation of lignocellulose. Nat. Commun. 2022, 13, 4716.
49. Li, S.; Dong, M.; Yang, J.; et al. Selective hydrogenation of 5-(hydroxymethyl)furfural to 5-methylfurfural over single atomic metals anchored on Nb2O5. Nat. Commun. 2021, 12, 584.
50. Liu, Y.; Gan, T.; He, Q.; Zhang, H.; He, X.; Ji, H. Catalytic oxidation of 5-hydroxymethylfurfural to 2,5-diformylfuran over atomically dispersed ruthenium catalysts. Ind. Eng. Chem. Res. 2020, 59, 4333-7.
51. Liu, S.; Bai, L.; van, M. A. P.; et al. Oxidative cleavage of β-O-4 bonds in lignin model compounds with a single-atom Co catalyst. Green. Chem. 2019, 21, 1974-81.
52. Meng, G.; Ji, K.; Zhang, W.; et al. Tandem catalyzing the hydrodeoxygenation of 5-hydroxymethylfurfural over a Ni3Fe intermetallic supported Pt single-atom site catalyst. Chem. Sci. 2021, 12, 4139-46.
53. Lou, Y.; Zhao, Y.; Liu, H.; et al. Edge-confined Pt1/MoS2 single-atom catalyst promoting the selective activation of carbon-oxygen bond. ChemCatChem 2021, 13, 2783-93.
54. Zhu, M.; Du, X.; Zhao, Y.; et al. Ring-opening transformation of 5-hydroxymethylfurfural using a golden single-atomic-site palladium catalyst. ACS. Catal. 2019, 9, 6212-22.
55. Li, Z.; Dong, X.; Zhang, M.; et al. Selective hydrogenation on a highly active single-atom catalyst of palladium dispersed on ceria nanorods by defect engineering. ACS. Appl. Mater. Interfaces. 2020, 12, 57569-77.
56. Meng, W.; Sun, S.; Xie, D.; et al. Engineering defective Co3O4 containing both metal doping and vacancy in octahedral cobalt site as high performance catalyst for methane oxidation. Mol. Catal. 2024, 553, 113768.
57. De, S.; Burange, A. S.; Luque, R. Conversion of biomass-derived feedstocks into value-added chemicals over single-atom catalysts. Green. Chem. 2022, 24, 2267-86.
58. Chen, J.; Xiao, Y.; Guo, F.; Li, K.; Huang, Y.; Lu, Q. Single-atom metal catalysts for catalytic chemical conversion of biomass to chemicals and fuels. ACS. Catal. 2024, 14, 5198-226.
59. Wang, D.; Shan, H.; Yin, W.; Li, H. Defect engineering of single-atom catalysts in biomass conversion. Fuel 2024, 355, 129439.
60. Lu, Y.; Zhang, Z.; Wang, H.; Wang, Y. Toward efficient single-atom catalysts for renewable fuels and chemicals production from biomass and CO2. Appl. Catal. B. Environ. 2021, 292, 120162.
61. Zhuang, J.; Wang, D. Recent advances of single-atom alloy catalyst: properties, synthetic methods and electrocatalytic applications. Mater. Today. Catal. 2023, 2, 100009.
62. Lu, Y.; Liu, T.; Dong, C. L.; et al. Tuning the selective adsorption site of biomass on Co3O4 by Ir single atoms for electrosynthesis. Adv. Mater. 2021, 33, e2007056.
63. Ge, R.; Wang, Y.; Li, Z.; et al. Selective electrooxidation of biomass-derived alcohols to aldehydes in a neutral medium: promoted water dissociation over a nickel-oxide-supported ruthenium single-atom catalyst. Angew. Chem. Int. Ed. 2022, 61, e202200211.
64. Gu, W.; Pei, A.; Zhang, S.; et al. Atomic-interface effect of single-atom Ru/CoOx for selective electrooxidation of 5-hydroxymethylfurfural. ACS. Appl. Mater. Interfaces. 2023, 15, 28036-43.
65. Xu, H.; Xin, G.; Hu, W.; et al. Single-atoms Ru/NiFe layered double hydroxide electrocatalyst: efficient for oxidation of selective oxidation of 5-hydroxymethylfurfural and oxygen evolution reaction. Appl. Catal. B. Environ. 2023, 339, 123157.
66. Zeng, L.; Chen, Y.; Sun, M.; et al. Cooperative Rh-O5/Ni(Fe) site for efficient biomass upgrading coupled with H2 production. J. Am. Chem. Soc. 2023, 145, 17577-87.
67. Ji, K.; Xu, M.; Xu, S. M.; et al. Electrocatalytic hydrogenation of 5-hydroxymethylfurfural promoted by a Ru1 Cu single-atom alloy catalyst. Angew. Chem. Int. Ed. 2022, 61, e202209849.
68. Zhou, Y.; Slater, T. J.; Luo, X.; Shen, Y. A versatile single-copper-atom electrocatalyst for biomass valorization. Appl. Catal. B. Environ. 2023, 324, 122218.
69. Wu, Y.; Jiang, Y.; Chen, W.; et al. Selective electroreduction of 5-hydroxymethylfurfural to dimethylfuran in neutral electrolytes via hydrogen spillover and adsorption configuration adjustment. Adv. Mater. 2024, 36, e2307799.
70. Zhou, P.; Chen, Y.; Luan, P.; et al. Selective electrochemical hydrogenation of furfural to 2-methylfuran over a single atom Cu catalyst under mild pH conditions. Green. Chem. 2021, 23, 3028-38.
71. Mukadam, Z.; Liu, S.; Pedersen, A.; et al. Furfural electrovalorisation using single-atom molecular catalysts. Energy. Environ. Sci. 2023, 16, 2934-44.
72. Wang, Y.; Zhu, Y.; Xie, Z.; et al. Efficient electrocatalytic oxidation of glycerol via promoted OH* generation over single-atom-bismuth-doped spinel Co3O4. ACS. Catal. 2022, 12, 12432-43.
73. Yu, H.; Wang, W.; Mao, Q.; et al. Pt single atom captured by oxygen vacancy-rich NiCo layered double hydroxides for coupling hydrogen evolution with selective oxidation of glycerol to formate. Appl. Catal. B. Environ. 2023, 330, 122617.
74. Feng, X.; Sun, T.; Feng, X.; et al. Single-atomic-site platinum steers middle hydroxyl selective oxidation on amorphous/crystalline homojunction for photoelectrochemical glycerol oxidation coupled with hydrogen generation. Adv. Funct. Mater. 2024, 34, 2316238.
75. Tian, Z.; Da, Y.; Wang, M.; et al. Selective photoelectrochemical oxidation of glucose to glucaric acid by single atom Pt decorated defective TiO2. Nat. Commun. 2023, 14, 142.
76. Ayele, A. A.; Tsai, M.; Adam, D. B.; et al. Electrochemical oxidation of ethylene glycol on TiO2-supported platinum single-atom catalyst into valuable chemicals in alkaline media. Appl. Catal. A. Gen. 2022, 646, 118861.
77. Moges, E. A.; Chang, C.; Huang, W.; et al. Sustainable synthesis of dual single-atom catalyst of Pd-N4/Cu-N4 for partial oxidation of ethylene glycol. Adv. Funct. Mater. 2022, 32, 2206887.
78. Chen, W.; Luo, X.; Slater, T. J. A.; et al. General synthesis of single atom electrocatalysts via a facile condensation-carbonization process. J. Mater. Chem. A. 2020, 8, 25959-69.
79. Ayele, A. A.; Tsai, M.; Awoke, Y. A.; et al. Ni-doped TiO2 supported Pt single-atom catalyst for partial oxidation of ethylene glycol to high-value chemicals in alkaline media. Mater. Today. Chem. 2023, 34, 101797.
80. Liu, R.; Zhang, L. Q.; Yu, C.; Sun, M. T.; Liu, J. F.; Jiang, G. B. Atomic-level-designed catalytically active palladium atoms on ultrathin gold nanowires. Adv. Mater. 2017, 29, 1604571.
81. Tiwari, J. N.; Dang, N. K.; Park, H. J.; et al. Remarkably enhanced catalytic activity by the synergistic effect of palladium single atoms and palladium-cobalt phosphide nanoparticles. Nano. Energy. 2020, 78, 105166.
82. Li, M.; Duanmu, K.; Wan, C.; et al. Single-atom tailoring of platinum nanocatalysts for high-performance multifunctional electrocatalysis. Nat. Catal. 2019, 2, 495-503.
83. Li, S.; Guan, A.; Wang, H.; et al. Hybrid palladium nanoparticles and nickel single atom catalysts for efficient electrocatalytic ethanol oxidation. J. Mater. Chem. A. 2022, 10, 6129-33.
84. Zhang, B.; Lai, W.; Sheng, T.; et al. Ordered platinum-bismuth intermetallic clusters with Pt-skin for a highly efficient electrochemical ethanol oxidation reaction. J. Mater. Chem. A. 2019, 7, 5214-20.
85. Luo, S.; Zhang, L.; Liao, Y.; et al. A tensile-strained Pt-Rh single-atom alloy remarkably boosts ethanol oxidation. Adv. Mater. 2021, 33, e2008508.
86. Zhang, G.; Cao, D.; Guo, S.; et al. Tuning the selective ethanol oxidation on tensile-trained Pt(110) surface by Ir single atoms. Small 2022, 18, e2202587.
87. Zhang, G.; Hui, C.; Yang, Z.; et al. Hydrogen-induced p-d orbital hybridization and tensile strain of PdGa single-atom alloy metallene boosts complete electrooxidation of ethanol. Appl. Catal. B. Environ. 2024, 342, 123377.
88. Lan, B.; Huang, M.; Wei, R. L.; Wang, C. N.; Wang, Q. L.; Yang, Y. Y. Ethanol electrooxidation on rhodium-lead catalysts in alkaline media: high mass activity, long-term durability, and considerable CO2 selectivity. Small 2020, 16, e2004380.
89. Zhu, C.; Lan, B.; Wei, R.; Wang, C.; Yang, Y. Potential-dependent selectivity of ethanol complete oxidation on rh electrode in alkaline media: a synergistic study of electrochemical ATR-SEIRAS and IRAS. ACS. Catal. 2019, 9, 4046-53.
90. Chang, Q.; Hong, Y.; Lee, H. J.; et al. Achieving complete electrooxidation of ethanol by single atomic Rh decoration of Pt nanocubes. Proc. Natl. Acad. Sci. USA. 2022, 119, e2112109119.
91. Pei, A.; Li, G.; Zhu, L.; et al. Nickel hydroxide-supported Ru single atoms and Pd nanoclusters for enhanced electrocatalytic hydrogen evolution and ethanol oxidation. Adv. Funct. Mater. 2022, 32, 2208587.
92. Wang, H.; Jiao, L.; Zheng, L.; et al. PdBi single-atom alloy aerogels for efficient ethanol oxidation. Adv. Funct. Mater. 2021, 31, 2103465.
93. Zhang, Z.; Liu, J.; Wang, J.; et al. Single-atom catalyst for high-performance methanol oxidation. Nat. Commun. 2021, 12, 5235.
94. Poerwoprajitno, A. R.; Gloag, L.; Watt, J.; et al. A single-Pt-atom-on-Ru-nanoparticle electrocatalyst for CO-resilient methanol oxidation. Nat. Catal. 2022, 5, 231-7.
95. Chen, L.; Liang, X.; Wang, D.; et al. Platinum-ruthenium single atom alloy as a bifunctional electrocatalyst toward methanol and hydrogen oxidation reactions. ACS. Appl. Mater. Interfaces. 2022, 14, 27814-22.
96. Kong, F.; Liu, X.; Song, Y.; et al. Selectively coupling Ru single atoms to PtNi concavities for high-performance methanol oxidation via d-band center regulation. Angew. Chem. Int. Ed. 2022, 61, e202207524.
97. Zhuang, L.; Jia, Z.; Wang, Y.; et al. Nitrogen-doped carbon black supported synergistic palladium single atoms and nanoparticles for electrocatalytic oxidation of methanol. Chem. Eng. J. 2022, 438, 135585.
98. Ruan, J.; Chen, Y.; Zhao, G.; et al. Cobalt single atoms enabling efficient methanol oxidation reaction on platinum anchored on nitrogen-doped carbon. Small 2022, 18, e2107067.
99. Lv, J.; Feng, W.; Yang, S.; Liu, H.; Huang, X. Methanol dissociation and oxidation on single Fe atom supported on graphitic carbon nitride. Appl. Organom. Chem. 2019, 33, e4930.
100. Fan, X.; Chen, W.; Xie, L.; et al. Surface-enriched single-Bi-atoms tailoring of Pt nanorings for direct methanol fuel cells with ultralow-Pt-loading. Adv. Mater. 2024, 36, e2313179.
101. Wei, X.; Zhang, J.; Liu, C.; Han, X.; Deng, Y.; Hu, W. Ir single atoms doped cuboctahedral Pd for boosted methanol oxidation reaction. Part. Part. Syst. Charact. 2022, 39, 2200013.
102. Miao, J.; Ma, Y.; Wang, X.; et al. Efficiently selective C(O-)-C bond cleavage for full lignocellulose upgrading coupled with energy-saving hydrogen production by Ir single-atom electrocatalyst. Appl. Catal. B. Environ. 2023, 336, 122937.
103. Cui, T.; Ma, L.; Wang, S.; et al. Atomically Dispersed Pt-N3C1 sites enabling efficient and selective electrocatalytic C-C bond cleavage in lignin models under ambient conditions. J. Am. Chem. Soc. 2021, 143, 9429-39.
Cite This Article

How to Cite
Ma, J.; Yan, H.; Hou, X.; Xu, M.; Cui, T. Single-atom catalysts for (photo)electrocatalytic biomass valorization to high-value-added chemicals. Microstructures 2025, 5, 2025002. http://dx.doi.org/10.20517/microstructures.2024.38
Download Citation
Export Citation File:
Type of Import
Tips on Downloading Citation
Citation Manager File Format
Type of Import
Direct Import: When the Direct Import option is selected (the default state), a dialogue box will give you the option to Save or Open the downloaded citation data. Choosing Open will either launch your citation manager or give you a choice of applications with which to use the metadata. The Save option saves the file locally for later use.
Indirect Import: When the Indirect Import option is selected, the metadata is displayed and may be copied and pasted as needed.
About This Article
Copyright
Data & Comments
Data
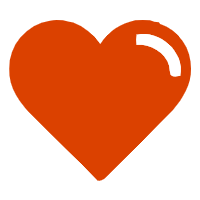
Comments
Comments must be written in English. Spam, offensive content, impersonation, and private information will not be permitted. If any comment is reported and identified as inappropriate content by OAE staff, the comment will be removed without notice. If you have any queries or need any help, please contact us at support@oaepublish.com.