First-principles study on crystal structures and superconductivity of ternary metal hydride La-Sr-H under high pressure
Abstract
The discovery of cage hydrides (Y,Ca)H6, (La,Ce)H9, and (La,Y)H10 indicates the appeal of ternary hydrides as contenders for high-temperature superconductors. Herein, we systematically studied a La-Sr-H system and predicted interesting stable and metastable hydrides at 200 GPa by first-principles calculations. The results revealed that LaSrH21 has a high superconducting transition temperature (Tc) of 211 K at 200 GPa, mainly attributed to its unique H12 rings. LaSrH12, LaSr2H18, and LaSr3H24 contain distorted H24 cages and exhibit high Tc values of 160, 167, and 169 K, respectively. Notably, LaSrH12 maintained dynamic stability down to 30 GPa, which is extremely low for H24 cage hydrides. In addition, the Tcs of LaSrH12 increased at a rate of 0.43 K/GPa with the increase in pressure. Further analysis revealed that the positive pressure-dependent Tc was mainly due to the softening of the low-frequency phonon modes and Lifshitz transition. Our work will guide the study of ternary rare- and alkaline-earth hydrides.
Keywords
INTRODUCTION
The Bardeen-Cooper-Schrieffer (BCS) theory suggests that high Debye temperature and pronounced electron-phonon coupling (EPC) of metallic hydrogen make it a promising candidate for high-temperature superconductivity[1-3]. Pressure is an important thermodynamic parameter that can shorten atomic distance and regulate the electronic structure of materials[4,5]. It is well known that it can transform the insulating hydrogen to a metallic state. However, a pressure of at least 500 GPa is required for the atomic metallic phase of hydrogen[6,7], which is not feasible under the current experimental conditions. In 2004, Ashcroft proposed the concept of chemical precompression[8] to attain high-temperature superconductivity at lower pressures[9]. Based on this principle, earlier studies could only focus on naturally occurring hydrides such as AlH3[10] and SiH4[11]. Although these studies revealed the need for effective reductions in pressure to enter the superconducting state, the superconducting transition temperatures (Tc) failed to reach satisfactory levels. With the development of crystal structure prediction[12-19], numerous nonstoichiometric superhydrides with high Tc have been theoretically predicted, and some have been experimentally synthesized[20-32]. Hydrogen-based superconductors H3S[20-23] and LaH10[24-28] are widely concerned. H3S is a covalent hydride in which
Thus far, the superconductivities of almost all binary hydrides have been systematically predicted, and most of them, such as CaH6[31] (215 K at 172 GPa), CeH9[35] (57 K at 85 GPa), and ThH10[36] (159-161 K at
In previous studies of binary hydrides, strontium (Sr)[48-52] and lanthanum (La) hydrides have attracted considerable attention because of their unique crystal structures and remarkable superconducting properties. Sr hydrides include several compounds with a high hydrogen content. SrH6, with linear and bent H3 units, transforms into spiral polymer H chains at 250 GPa[53]; SrH10 possesses a graphene-like H-layer at 300 GPa, and SrH22, which is the metal hydride with the highest hydrogen content discovered thus far, contains H2 units at 146 GPa. The rare-earth metal La contains more valence electrons than the alkaline-earth Sr and can transfer more electrons to hydrogen atoms. This condition enables H to obtain sufficient electrons to fill the antibonding σ* orbitals, which weakens the H-H bonds in the H2 and H3 units. Therefore, introducing La into binary Sr-H systems can form new ternary hydrides, which may exhibit novel properties, such as high Tc, under high pressure.
In this work, we determined the crystal structures and superconducting properties of a La-Sr-H system at 200 GPa and observed the thermodynamically stable LaSrH_P
COMPUTATIONAL DETAILS
The structure of the La-Sr-H system at 200 GPa was searched using the USPEX[16,18] (Universal Structure Predictor: Evolutionary Xtallography) structure prediction method coupled with ab initio geometry optimizations implemented in Vienna ab initio simulation packages (VASP) code[54]. During the variable-component search, the maximum number of atoms was set to 28. The number of generations was set to 30, in which the first generation contained 200 individuals and each subsequent generation involved 50 individuals. After the structural search, we evaluated the thermodynamic and structural stability of the system using the convex hull method and phonon calculations. The crystal structure was optimized using the VASP code, which was used to calculate the electronic properties. We selected Perdew-Burke-Ernzerhof (PBE)[55] of the generalized gradient approximation (GGA)[56] as the exchange-correlation function. In addition, the all-electron projector-augmented wave method[57] was used to describe ion-electron interactions. To ensure that the enthalpy converged to less than 1 meV/atom, we set the cutoff energy to
Phonon calculations were performed using the supercell method implemented in the PHONOPY[59] code, which was also used for zero-point energy (ZPE) corrections. The EPC and superconducting properties were calculated using density functional perturbation theory, as implemented in the Quantum-ESPRESSO code[59,60]. Ultrasoft pseudopotential[61] and GGA-PBE were used with a kinetic energy cutoff of 85 Ry and a charge density cutoff of 850 Ry. During EPC calculation, the numbers of q- and k-points were as follows:
Allen-Dynes formula was used to calculate the Tc of La-Sr-H compounds[62]:
where μ* refers to the Coulomb pseudopotential; λ and ω1og represent the EPC constant and logarithmic average frequency, respectively.
Here, α2F(ω) is an Eliashberg function.
when λ < 1.5, f1f2 = 1. Meanwhile, when λ > 1.5, the f1, f2 can be calculated as follows:
where
RESULTS AND DISCUSSIONS
A structural search of LaxSryHz (x = 1-3, y = 1-3, z = 1-24) was performed within 60,000 structures at
Figure 1. Ternary-phase diagram of the La-Sr-H system at 200 GPa with ZPE. Dark cyan dots represent stable structures, and other colored dots denote metastable structures.
Figure 2. Crystal structures of the La-Sr-H system at 200 GPa. (A) LaSrH_P
Compounds LaSrH and LaSrH4 were structurally stable with P
Figure 3. (A) Electronic band structures and projected DOS (PDOS) and (B) calculated phonon dispersion curves, Eliashberg spectral function α2F(ω) with electron-phonon integral λ(ω), and projected phonon DOS (PHDOS) for LaSrH8_Pmma 200 GPa.
Superconducting parameters of La-Sr-H system compounds
Phase | Pressure (GPa) | NEf (states/spin/Ry/f.u.) | ω1og (K) | λ | Tc (K) |
LaSrH8_Pmma | 200 | 11.48 | 933.2 | 1.00 | 60-71 |
LaSrH12_R3m | 30 | 5.39 | 659.5 | 1.39 | 80-90 |
LaSrH12_R3m | 50 | 5.14 | 854.0 | 1.21 | 84-95 |
LaSrH12_R3m | 100 | 4.84 | 1,002.8 | 1.25 | 104-117 |
LaSrH12_R3m | 150 | 4.99 | 944.5 | 1.53 | 133-146 |
LaSrH12_R3m | 200 | 5.55 | 859.3 | 1.70 | 146-160 |
LaSr2H18_C2/m | 200 | 8.27 | 921.6 | 1.52 | 152-167 |
LaSr3H24_R | 200 | 11.99 | 1,168.2 | 1.25 | 148-169 |
LaSrH21_Cm | 200 | 7.11 | 892.1 | 2.11 | 196-211 |
We also discovered an interesting metastable compound, LaSrH21, with a layered structure and Cm symmetry. The layered structure containing La atoms was stacked in an ABA fashion, in which the A layer consisted of wrinkled H12 rings, and the B layer comprised La atoms located below the center of the H12 ring [Figure 2G]. The H12 ring contained four different H-H bonds with lengths of 0.94, 1.04, 1.10, and 1.20 Å at 200 GPa, longer than the H-H bond length (~0.8 Å) in the H2 molecular units of SrH22. This result was due to the electrons obtained by hydrogen occupying the antibonding orbitals, which weakened the interactions between the hydrogen atoms and increased the bond length. In order to investigate the H-H bonding character in more detail, we calculated the electron localization function (ELF) and COHP of H12 rings, as shown in Figure 4. As provided in Figure 4A, the ELF values of H atoms equal 0.6-0.8 indicating covalent bonds among H atoms. In Figure 4B, the left sides with negative values represent bonding states, and the right sides are antibonding states. The integrated COHP (ICOHP) up to the Fermi level represents the bonding interactions between H atoms; larger negative values represent stronger H-H bonds. The ICOHP values of those H-H distances such as 0.94, 1.04, 1.10, and 1.20 Å are -2.45, -1.62, -1.64 and -0.85 eV, respectively, indicating this unique H12 ring was connected by weak covalent interactions. Furthermore, the band structures (left panel) and PDOS (right panel) of LaSrH21 were calculated [Figure 5A]. The conduction and valence bands overlapped near the Fermi level, implying the metallic nature of this structure. The total DOS at the Fermi level is 7.11 states/spin/Ry/f.u., higher than that of LaSrH12. However, the DOS value of
Figure 4. (A) ELF of LaSrH21_Cm at 200 GPa of H12 rings and (B) the COHP of four types of H-H bonds in H12 rings for LaSrH21_Cm at
Figure 5. (A) Electronic band structures and PDOS and (B) calculated phonon dispersions, Eliashberg spectral function α2F(ω), electron-phonon integral λ(ω), and projected PHDOS for LaSrH21_Cm at 200 GPa.
We also observed a series of hexahydrides (LaSrH12, LaSr2H18, and LaSr3H24) with R3m, C2/m, and R
Figure 6. Electronic band structures and PDOS of LaSrH12_R3m at (A) 50, (B) 100, (C) 150, and (D) 200 GPa. The sizes of circles in the band structures denote the contributions of H atoms and the f orbital of La atoms. The red and blue dots represent H and La atoms, respectively.
Figure 7. Calculated electronic band structures and PDOS of (A) LaSr2H18_C2/m and (B) LaSr3H24_R
Figure 8. Phonon band structures, Eliashberg spectral function α2F(ω) with electron-phonon integral λ(ω), and projected PHDOS of (A) LaSr2H18_C2/m and (B) LaSr3H24_R
The crystal structure of LaSrH12 at 50 GPa is depicted in Figure 9A. We found half of the H atoms existed in the form of H2 units. The H-H distance in H2 units is 0.89 Å at 50 GPa, which elongates with pressure. At 200 GPa, it extends to 1.04 Å, much longer than the typical H-H bond length of 0.74 Å in pure solid hydrogen. Besides, we calculated its ELF map and COHP to study the H-H bonding character in detail. As shown in Figure 9B, the ELF and negative ICOHP values between H-H in H2 units are 1.0 and
Figure 9. (A) The crystal structure of LaSrH12 at 50 GPa. (B) the ELF of LaSrH12 on the (111) plane at 50 and 200 GPa. (C) The COHP of the nearest distances of H-H for LaSrH12_R3m at 50, 100, 150 and 200 GPa.
To examine the electronic properties of LaSrH12 with increasing pressure, its electronic structures were calculated at 50, 100, 150, and 200 GPa [Figure 6]. As the pressure rose to 100 GPa, the lowest conduction band at the Γ point gradually descended but did not exceed the Fermi energy. In addition, the valence band located on the Fermi surface at the H-point moved upward, which caused the total electronic DOS at the Fermi level to decrease to 4.84 states/spin/Ry/f.u. Remarkably, with further pressure elevation, the lowest conduction band at the Γ point accepted electrons, which formed a new electronic pocket at the Fermi level. The Fermi surface had undergone geometric changes, marking Lifshitz transition. As a result, the total electron DOS at the Fermi level increased to 5.55 states/spin/Ry/f.u. at 200 GPa[66].
We further calculated the phonon band structures, Eliashberg spectral functions α2F(ω), and the EPC parameter λ(ω) for LaSrH12 under various pressures [Figure 10]. La and Sr atoms have heavy atomic masses; thus, the low-frequency vibrational modes below 500 cm-1 mainly corresponded to the vibrations of La and Sr atoms with a contribution below 30% of the total λ. The high-frequency regions above 1,700 cm-1 were primarily dominated by the H2 stretching vibration mode, which accounted for less than 2% of the total λ. The lighter H atoms, which drove the phonon modes in the middle-frequency region, contributed greatly to the EPC and accounted for more than 65% of the total λ. It is noted that the vibration modes in the high-frequency parts are obviously down with rising pressure, and until 200 GPa, there is no clear boundary between the high- and intermediate-frequency regions. The reason is the weakening interaction between H atoms in H2 units. Besides, we observed that the lowest frequency transverse acoustic phonon mode at points A and M gradually softened with increasing pressure. The emergence of soft modes was beneficial for enhancing the EPC and Tc.
Figure 10. Phonon band structures, Eliashberg spectral function α2F(ω) with electron-phonon integral λ(ω), and projected PHDOS of LaSrH12 at (A) 50, (B) 100, (C) 150, and (D) 200 GPa.
Table 1 lists the critical temperature Tc of LaSrH12 at various pressures obtained by solving the Eliashberg equations using typical Coulomb pseudopotential parameters µ* = 0.1 and 0.13. TheTc of LaSrH12 is estimated to be within 84-95 K at 50 GPa, almost twice that of LaCaH12(40.1-46.6 K at 50 GPa)[67] and it increased nearly linearly with pressure (104-117 K at 100 GPa, 133-146 K at 150 GPa, and 146-160 K at
CONCLUSION
We used evolutionary algorithms to explore the structures of solid La-Sr-H systems at 200 GPa for structural analysis. Thermodynamically stable LaSrH, LaSrH4, LaSrH12, and LaSr3H24 and metastable LaSrH8, LaSr2H18, and LaSrH21 were identified. However, LaSrH and LaSrH4 did not exhibit superconductivity. LaSrH8 became a superconductor near the liquid-nitrogen temperature. LaSrH21 exhibited a high Tc of 211 K, where hydrogen atoms around the La atoms were arranged in H12 rings, which played an important role in attaining a high Tc. LaSrH12, LaSr2H18, and LaSr3H24 contained La- and Sr-centered H24 cages and exhibited high Tc of 160, 167, and 169 K at 200 GPa, respectively. Further calculations showed that LaSrH12 can maintain its dynamic stability up to 30 GPa. In addition, the Tc of LaSrH12 increased with pressure at a rate of 0.43 K/GPa, and this finding was mainly attributed to the softening mode and Lifshitz transition.
DECLARATIONS
Authors’ contributions
Contributed to the study’s conception and design, investigation, original draft writing, editing and review: Chen, L.; Jiang, Q.; Zhang, Z.; Liu, Z.; Huo, Z.; Ma, H.; Guo, S.; Yang, S.; Duan, D.
Availability of data and materials
The data supporting the findings of this study are available within this Article and its Supplementary Material. Further data are available from the corresponding authors upon request.
Financial support and sponsorship
This work was supported by the National Natural Science Foundation of China (No. 12122405,
Conflicts of interest
All authors declared that there are no conflicts of interest.
Ethical approval and consent to participate
Not applicable.
Consent for publication
Not applicable.
Copyright
© The Author(s) 2025.
Supplementary Materials
REFERENCES
1. Ashcroft, N. W. Metallic hydrogen: a high-temperature superconductor? Phys. Rev. Lett. 1968, 21, 1748-9.
2. Bardeen, J.; Cooper, L. N.; Schrieffer, J. R. Microscopic theory of superconductivity. Phys. Rev. 1957, 106, 162-4.
3. Zhao, W.; Song, H.; Du, M.; et al. Pressure-induced high-temperature superconductivity in ternary Y-Zr-H compounds. Phys. Chem. Chem. Phys. 2023, 25, 5237-43.
4. Edalati, K.; Bachmaier, A.; Beloshenko, V. A.; et al. Nanomaterials by severe plastic deformation: review of historical developments and recent advances. Mater. Res. Lett. 2022, 10, 163-256.
5. Kang, S.; Zheng, H.; Liu, T.; et al. A ferromagnetically coupled Fe42 cyanide-bridged nanocage. Nat. Commun. 2015, 6, 5955.
6. Dalladay-Simpson, P.; Howie, R. T.; Gregoryanz, E. Evidence for a new phase of dense hydrogen above 325 gigapascals. Nature 2016, 529, 63-7.
7. Loubeyre, P.; Occelli, F.; Dumas, P. Synchrotron infrared spectroscopic evidence of the probable transition to metal hydrogen. Nature 2020, 577, 631-5.
8. Ashcroft, N. W. Hydrogen dominant metallic alloys: high temperature superconductors? Phys. Rev. Lett. 2004, 92, 187002.
9. Du, M.; Zhao, W.; Cui, T.; Duan, D. Compressed superhydrides: the road to room temperature superconductivity. J. Phys. Condens. Matter. 2022, 34, 173001.
10. Goncharenko, I.; Eremets, M. I.; Hanfland, M.; et al. Pressure-induced hydrogen-dominant metallic state in aluminum hydride. Phys. Rev. Lett. 2008, 100, 045504.
11. Eremets, M. I.; Trojan, I. A.; Medvedev, S. A.; Tse, J. S.; Yao, Y. Superconductivity in hydrogen dominant materials: silane. Science 2008, 319, 1506-9.
12. Pickard, C. J.; Needs, R. J. Ab initio random structure searching. J. Phys. Condens. Matter. 2011, 23, 053201.
13. Wang, Y.; Lv, J.; Zhu, L.; Ma, Y. Crystal structure prediction via particle-swarm optimization. Phys. Rev. B. 2010, 82, 094116.
14. Wang, Y.; Lv, J.; Zhu, L.; Ma, Y. CALYPSO: a method for crystal structure prediction. Comput. Phys. Commun. 2012, 183, 2063-70.
15. Gao, H.; Wang, J.; Han, Y.; Sun, J. Enhancing crystal structure prediction by decomposition and evolution schemes based on graph theory. Fundam. Res. 2021, 1, 466-71.
16. Oganov, A. R.; Glass, C. W. Crystal structure prediction using ab initio evolutionary techniques: principles and applications. J. Chem. Phys. 2006, 124, 244704.
17. Oganov, A. R.; Lyakhov, A. O.; Valle, M. How evolutionary crystal structure prediction works-and why. ACC. Chem. Res. 2011, 44, 227-37.
18. Lyakhov, A. O.; Oganov, A. R.; Stokes, H. T.; Zhu, Q. New developments in evolutionary structure prediction algorithm USPEX. Comput. Phys. Commun. 2013, 184, 1172-82.
19. Xia, K.; Gao, H.; Liu, C.; et al. A novel superhard tungsten nitride predicted by machine-learning accelerated crystal structure search. Sci. Bull. 2018, 63, 817-24.
20. Duan, D.; Liu, Y.; Tian, F.; et al. Pressure-induced metallization of dense (H2S)2H2 with high-Tc superconductivity. Sci. Rep. 2014, 4, 6968.
21. Duan, D.; Huang, X.; Tian, F.; et al. Pressure-induced decomposition of solid hydrogen sulfide. Phys. Rev. B. 2015, 91, 180502.
22. Drozdov, A. P.; Eremets, M. I.; Troyan, I. A.; Ksenofontov, V.; Shylin, S. I. Conventional superconductivity at 203 kelvin at high pressures in the sulfur hydride system. Nature 2015, 525, 73-6.
23. Einaga, M.; Sakata, M.; Ishikawa, T.; et al. Crystal structure of the superconducting phase of sulfur hydride. Nat. Phys. 2016, 12, 835-8.
24. Peng, F.; Sun, Y.; Pickard, C. J.; Needs, R. J.; Wu, Q.; Ma, Y. Hydrogen clathrate structures in rare earth hydrides at high pressures: possible route to room-temperature superconductivity. Phys. Rev. Lett. 2017, 119, 107001.
25. Liu, H.; Naumov, I. I.; Hoffmann, R.; Ashcroft, N. W.; Hemley, R. J. Potential high-Tc superconducting lanthanum and yttrium hydrides at high pressure. Proc. Natl. Acad. Sci. USA. 2017, 114, 6990-5.
26. Drozdov, A. P.; Kong, P. P.; Minkov, V. S.; et al. Superconductivity at 250 K in lanthanum hydride under high pressures. Nature 2019, 569, 528-31.
27. Somayazulu, M.; Ahart, M.; Mishra, A. K.; et al. Evidence for superconductivity above 260 K in lanthanum superhydride at megabar pressures. Phys. Rev. Lett. 2019, 122, 027001.
28. Hong, F.; Yang, L.; Shan, P.; et al. Superconductivity of lanthanum superhydride investigated using the standard four-probe configuration under high pressures. Chinese. Phys. Lett. 2020, 37, 107401.
29. Troyan, I. A.; Semenok, D. V.; Kvashnin, A. G.; et al. Anomalous high-temperature superconductivity in YH6. Adv. Mater. 2021, 33, e2006832.
30. Kong, P.; Minkov, V. S.; Kuzovnikov, M. A.; et al. Superconductivity up to 243 K in the yttrium-hydrogen system under high pressure. Nat. Commun. 2021, 12, 5075.
31. Ma, L.; Wang, K.; Xie, Y.; et al. High-temperature superconducting phase in clathrate calcium hydride CaH6 up to 215 K at a pressure of 172 GPa. Phys. Rev. Lett. 2022, 128, 167001.
32. Jiang, Q.; Zhang, Z.; Song, H.; et al. Ternary superconducting hydrides stabilized via Th and Ce elements at mild pressures. Fundam. Res. 2024, 4, 550-6.
33. Errea, I.; Calandra, M.; Pickard, C. J.; et al. Quantum hydrogen-bond symmetrization in the superconducting hydrogen sulfide system. Nature 2016, 532, 81-4.
34. Errea, I.; Belli, F.; Monacelli, L.; et al. Quantum crystal structure in the 250-kelvin superconducting lanthanum hydride. Nature 2020, 578, 66-9.
35. Chen, W.; Semenok, D. V.; Huang, X.; et al. High-temperature superconducting phases in cerium superhydride with a Tc up to 115 K below a pressure of 1 megabar. Phys. Rev. Lett. 2021, 127, 117001.
36. Semenok, D. V.; Kvashnin, A. G.; Ivanova, A. G.; et al. Superconductivity at 161 K in thorium hydride ThH10: synthesis and properties. Mater. Today. 2020, 33, 36-44.
37. Sun, Y.; Lv, J.; Xie, Y.; Liu, H.; Ma, Y. Route to a superconducting phase above room temperature in electron-doped hydride compounds under high pressure. Phys. Rev. Lett. 2019, 123, 097001.
38. Zhang, Z.; Cui, T.; Hutcheon, M. J.; et al. Design principles for high-temperature superconductors with a hydrogen-based alloy backbone at moderate pressure. Phys. Rev. Lett. 2022, 128, 047001.
39. Song, Y.; Bi, J.; Nakamoto, Y.; et al. Stoichiometric ternary superhydride LaBeH8 as a new template for high-temperature superconductivity at 110 K under 80 GPa. Phys. Rev. Lett. 2023, 130, 266001.
40. Heil, C.; di, C. S.; Bachelet, G. B.; Boeri, L. Superconductivity in sodalite-like yttrium hydride clathrates. Phys. Rev. B. 2019, 99, 220502.
41. Xie, H.; Duan, D.; Shao, Z.; et al. High-temperature superconductivity in ternary clathrate CaYH12 under high pressures. J. Phys. Condens. Matter. 2019, 31, 245404.
42. Liang, X.; Bergara, A.; Wang, L.; et al. Potential high- Tc superconductivity in CaYH12 under pressure. Phys. Rev. B. 2019, 99, 100505.
43. Zhao, W.; Duan, D.; Du, M.; et al. Pressure-induced high-Tc superconductivity in the ternary clathrate system Y-Ca-H. Phys. Rev. B. 2022, 106, 014521.
44. Chen, W.; Huang, X.; Semenok, D. V.; et al. Enhancement of superconducting properties in the La-Ce-H system at moderate pressures. Nat. Commun. 2023, 14, 2660.
45. Bi, J.; Nakamoto, Y.; Zhang, P.; et al. Giant enhancement of superconducting critical temperature in substitutional alloy (La,Ce)H9. Nat. Commun. 2022, 13, 5952.
46. Semenok, D. V.; Troyan, I. A.; Ivanova, A. G.; et al. Superconductivity at 253 K in lanthanum-yttrium ternary hydrides. Mater. Today. 2021, 48, 18-28.
47. Ma, T.; Zhang, Z.; Du, M.; et al. High-throughput calculation for superconductivity of sodalite-like clathrate ternary hydrides MXH12 at high pressure. Mater. Today. Phys. 2023, 38, 101233.
48. Hooper, J.; Terpstra, T.; Shamp, A.; Zurek, E. Composition and constitution of compressed strontium polyhydrides. J. Phys. Chem. C. 2014, 118, 6433-47.
49. Wang, Y.; Wang, H.; Tse, J. S.; Iitaka, T.; Ma, Y. Structural morphologies of high-pressure polymorphs of strontium hydrides. Phys. Chem. Chem. Phys. 2015, 17, 19379-85.
50. Semenok, D. V.; Kruglov, I. A.; Savkin, I. A.; Kvashnin, A. G.; Oganov, A. R. On distribution of superconductivity in metal hydrides. Curr. Opin. Solid. State. Mater. Sci. 2020, 24, 100808.
51. Tanaka, K.; Tse, J. S.; Liu, H. Electron-phonon coupling mechanisms for hydrogen-rich metals at high pressure. Phys. Rev. B. 2017, 96, 100502.
52. Semenok, D. V.; Chen, W.; Huang, X.; et al. Sr-doped superionic hydrogen glass: synthesis and properties of SrH22. Adv. Mater. 2022, 34, e2200924.
53. Chen, Y.; Liu, Z.; Lin, Z.; et al. High Tc superconductivity in layered hydrides XH15 (X = Ca, Sr, Y, La) under high pressures. Front. Phys. 2022, 17, 63502.
54. Kresse, G.; Furthmüller, J. Efficient iterative schemes for ab initio total-energy calculations using a plane-wave basis set. Phys. Rev. B. Condens. Matter. 1996, 54, 11169-86.
55. Perdew, J. P.; Burke, K.; Ernzerhof, M. Generalized gradient approximation made simple. Phys. Rev. Lett. 1996, 77, 3865-8.
56. Perdew, J. P.; Wang, Y. Pair-distribution function and its coupling-constant average for the spin-polarized electron gas. Phys. Rev. B. Condens. Matter. 1992, 46, 12947-54.
57. Blöchl, P. E. Projector augmented-wave method. Phys. Rev. B. Condens. Matter. 1994, 50, 17953-79.
58. Maintz, S.; Deringer, V. L.; Tchougréeff, A. L.; Dronskowski, R. LOBSTER: a tool to extract chemical bonding from plane-wave based DFT. J. Comput. Chem. 2016, 37, 1030-5.
59. Togo, A.; Oba, F.; Tanaka, I. First-principles calculations of the ferroelastic transition between rutile-type and CaCl2-typeSiO2 at high pressures. Phys. Rev. B. 2008, 78, 134106.
60. Giannozzi, P.; Baroni, S.; Bonini, N.; et al. QUANTUM ESPRESSO: a modular and open-source software project for quantum simulations of materials. J. Phys. Condens. Matter. 2009, 21, 395502.
61. Vanderbilt, D. Soft self-consistent pseudopotentials in a generalized eigenvalue formalism. Phys. Rev. B. Condens. Matter. 1990, 41, 7892-5.
63. Wu, Y.; Lazic, P.; Hautier, G.; Persson, K.; Ceder, G. First principles high throughput screening of oxynitrides for water-splitting photocatalysts. Energy. Environ. Sci. 2013, 6, 157-68.
64. Hinuma, Y.; Hatakeyama, T.; Kumagai, Y.; et al. Discovery of earth-abundant nitride semiconductors by computational screening and high-pressure synthesis. Nat. Commun. 2016, 7, 11962.
65. Song, H.; Zhang, Z.; Cui, T.; Pickard, C. J.; Kresin, V. Z.; Duan, D. High Tc superconductivity in heavy rare earth hydrides. Chinese. Phys. Lett. 2021, 38, 107401.
66. Lifshitz, I. M. Anomalies of electron characteristics in the high pressure region. 1960. Available from: https://www.osti.gov/biblio/4173345 [Last accessed on 15 Jul 2024].
Cite This Article
How to Cite
Download Citation
Export Citation File:
Type of Import
Tips on Downloading Citation
Citation Manager File Format
Type of Import
Direct Import: When the Direct Import option is selected (the default state), a dialogue box will give you the option to Save or Open the downloaded citation data. Choosing Open will either launch your citation manager or give you a choice of applications with which to use the metadata. The Save option saves the file locally for later use.
Indirect Import: When the Indirect Import option is selected, the metadata is displayed and may be copied and pasted as needed.
About This Article
Special Issue
Copyright
Data & Comments
Data
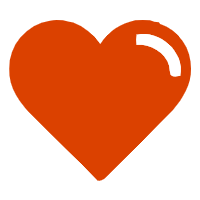
Comments
Comments must be written in English. Spam, offensive content, impersonation, and private information will not be permitted. If any comment is reported and identified as inappropriate content by OAE staff, the comment will be removed without notice. If you have any queries or need any help, please contact us at [email protected].