Visualizing small molecules via transmission electron microscopy
Abstract
Transmission electron microscopy (TEM) is a cutting-edge characterization technique renowned for its ability to achieve atomic resolution. Owing to its exceptional capacity for microscopic characterization, TEM has emerged as an essential and powerful tool in the realms of structural characterization and chemical analysis. Its applications span a diverse array of fields, including materials science, chemistry, and biology, offering unprecedented insights into the fundamental structure understanding of various substances. The capability of TEM to facilitate direct observation of small molecules holds significant promise for advancing our understanding of molecular structures, host-guest interactions, and their dynamic behaviors. However, a couple of challenges hide this potential. Notably, issues such as electron beam irradiation can damage small molecules, while low contrast of small molecules compared to the background presents considerable obstacles during imaging. These factors necessitate the continued development of innovative techniques that enhance the efficacy of TEM. In this review, we offer a brief introduction to advanced TEM techniques aimed at directly imaging small molecules, including cryo-electron microscopy, low electron dose imaging, and in situ TEM techniques. We review recent advancements in atomic-resolution TEM studies involving small molecules, highlighting significant findings and methodological improvements that have emerged in the field. In addition, we provide an outlook on the future trajectory of TEM studies on small molecules, emphasizing the potential progress that could stimulate further scientific exploration in this realm.
Keywords
INTRODUCTION
A molecule represents the smallest unit of a substance that can exist independently while maintaining a relatively stable structure, along with its inherent physical and chemical properties. Molecules are composed of atoms that are intricately arranged in specific configurations, which are crucial for defining the characteristics and behavior of the substance they form. Generally, molecules can be classified into two main categories based on their size, structural complexity, and molecular weight: small molecules and macromolecules. These distinctions help to differentiate substances based on their fundamental properties and roles in various scientific disciplines. For example, small molecules typically possess dimensions smaller than 1.0 nanometer (nm) and have molecular weights below 400 Daltons (Da)[1]. Well-known examples of small molecules include carbon dioxide (CO2), water (H2O), and glucose (C6H12O6), which play essential roles in various chemical and biological processes. In contrast, macromolecules are significantly larger, surpassing the 1.0 nm threshold and exhibiting molecular weights that exceed 5,000 Da[2]. This category encompasses complex structures such as proteins and nucleic acids. Macromolecules often exhibit three-dimensional shapes and perform numerous critical tasks within biological systems, including catalyzing biochemical reactions, storing genetic information, and providing structural support to cells.
Understanding molecular structure is fundamental across various scientific disciplines, including materials science[3], chemistry[4], and biology[5]. The arrangement of atoms within a molecule plays a crucial role in determining its properties and behavior. For instance, Kekule's groundbreaking elucidation of benzene structure revealed its unique closed-ring configuration, which characterizes aromatic compounds[6]. This discovery effectively distinguished aromatic compounds from aliphatic compounds, which are composed of open-chain structures. Similarly, the landmark research conducted by Watson and Crick on the DNA double helix structure marked a pivotal moment in genetics[7]. Their insights not only elucidated the physical form of DNA but also provided a framework for understanding genetic replication. As they astutely noted, “It has not escaped our notice that the specific pairing we have postulated immediately suggests a possible copying mechanism for the genetic material”. This revelation underscored the intricate relationship between molecular structure and biological function. Moreover, grasping the behavior of macromolecular compounds within cellular environments is central to the field of molecular biology. Structural biology focuses on analyzing the three-dimensional arrangement of atoms in macromolecules, such as proteins and nucleic acids, to infer their functions within living organisms. By understanding these complex structures, researchers can unlock the mechanisms behind vital biological processes[8]. The global COVID-19 pandemic has further highlighted the critical importance of correlating molecular structure with functional properties. In the quest to develop effective antiviral drugs, scientists have prioritized acquiring detailed insights into the three-dimensional structures of viral proteins that facilitate cellular infection and viral replication[9]. This knowledge empowers chemists and pharmacologists to design targeted compounds that can inhibit the virus’s ability to replicate and spread, ultimately contributing to public health efforts. Additionally, studying molecular structures is also paramount in the fields of materials science and chemistry. For example, understanding the interactions between small molecules and active sites in catalysts is essential for optimizing materials used in applications such as adsorption and catalysis. These interactions can significantly influence the efficiency and effectiveness of catalytic processes, driving advancements in industrial chemistry and environmental science[10,11].
Currently, a wide array of experimental techniques is employed to study molecular structures, each providing valuable insights into different aspects of a molecule's properties and behavior. The most commonly used tools are X-ray diffraction (XRD), electron diffraction, nuclear magnetic resonance (NMR), and infrared spectroscopy (IR)[12]. For example, by analyzing XRD patterns produced by the scattering of X-rays from atoms in the molecular structure, scientists can determine the arrangement of atoms and derive information about their symmetry, size, and atomic positions. One of the landmark applications of XRD in molecular structure study was the famous diffraction of DNA obtained by Rosalind Franklin in 1951[13]. While XRD is widely used for crystalline materials, it requires high-quality single crystals, which limits its applicability to materials that are difficult to crystallize. Electron diffraction has emerged as a powerful atomic-scale characterization technique, particularly suited for nanometer-sized crystallites and electron beam-sensitive materials. It can be performed on extremely small crystal, making it applicable to a broader range of materials. Recent advancements in continuous rotation electron diffraction (cRED) have enabled researchers to acquire structural data within an extremely short time frame (< 1 min per dataset) and at ultralow electron dose rates (< 0.1 e-/Å2/s), significantly broadening its applications in characterizing highly sensitive materials such as metal-organic frameworks (MOFs) and covalent organic frameworks (COFs)[14]. For instance, Zhang et al. utilized cryogenic electron diffraction to resolve temperature-dependent pore size variations in COF-320[15], while Wang et al. employed three-dimensional electron diffraction to localize guest molecules within MOFs[16], highlighting its potential in studying host-guest interactions. NMR spectroscopy is another powerful analytical technique that provides in-depth information about the structure and behavior of molecules. NMR works by exploiting the magnetic properties of certain atomic nuclei, most commonly hydrogen (1H) and carbon (13C)[17]. When placed in a magnetic field, these nuclei absorb and re-emit radiofrequency radiation, producing spectra that can be analyzed to reveal a wealth of structure information about the molecule[18]. Infrared spectroscopy (IR) is also an important method for characterizing molecular structures, particularly by analyzing the vibrational modes of molecules[19]. When a molecule absorbs infrared radiation, its bonds undergo vibrational motion. The frequencies at which a molecule absorbs infrared light correspond to the vibrational frequencies of its chemical bonds. By measuring the absorption spectrum and comparing it to reference spectra or theoretical models, various structural characteristics of the molecules can be determined.
These mentioned techniques are essential for elucidating the structure and revealing the structure-property relationships of small molecules. For instance, Zhang et al. employed XRD and NMR to explore the conformation of the o-methylation modulation of N,N'-diphenyl-dihydrodibenzo[a,c]phenazine (DPAC), one of the small organic molecules[20]. It is confirmed that changes in the bending angle of the molecule directly affect its optical properties. Hong et al. employed infrared spectroscopy to analyze the structural features of polysaccharides[21]. The study identified the specific chemical bonds and functional groups present in different monosaccharide units, as well as the substitution patterns, including methylation, acetylation, and phosphorylation, which were distinguished by characteristic absorption bands in the infrared spectra.
While these techniques are undoubtedly powerful, they primarily focus on characterizing molecular structures through spectroscopy analysis. However, they often fall short of providing direct real-space information regarding the arrangement of atoms within a molecule, complicating the precise representation of molecular configurations. In addition, the spectroscopy characterization methods are inherently limited by their spatial resolution, as the signals obtained typically represent an average from a large ensemble of molecules. This averaging effect can obscure the unique structural features of individual molecules, making it challenging to accurately depict the specific architecture of any given structure[22,23].
To address these limitations, microscopy techniques have emerged as essential complementary tools to spectroscopy characterization. The advent of optical microscopy[24,25] revolutionized cytology and biological studies, following the first glimpse into the cellular world. In addition, electron microscopy has similarly had a profound impact across multiple scientific disciplines. When electron microscopy was first introduced, it offered groundbreaking progress in resolution, enabling scientists to explore materials science, chemistry, and physics with unparalleled clarity. This technological breakthrough significantly extended the capabilities of traditional optical microscopy, overcoming its inherent limitations in resolution. Electron microscopy offers detailed insights into molecular architectures by visualizing the spatial arrangement of atoms within a molecule. This capability is particularly valuable in fields like nanotechnology and biochemistry, where understanding molecular interactions and structures is critical for the development of new materials and drug therapies. Moreover, the integration of spectroscopic and microscopic techniques fosters a deeper, more nuanced understanding of molecular systems.
Actually, electron microscopy has made significant strides in the study of macromolecular structures, particularly in the imaging of biomolecules such as proteins and nucleic acids, providing crucial support for the advancement of fields like biology and antibody development. In addition, imaging of small molecules is equally important, particularly in fields such as materials science[26-28] and energy[29]. However, unlike the well-established approaches for macromolecular imaging in structural biology, electron microscopy studies on small molecules remain limited due to challenges such as beam sensitivity, sample preparation, and low contrast relative to the background. In this review, we present a brief introduction to advanced transmission electron microscopy (TEM) methodologies designed for direct imaging of small molecules, including cryo-EM, low electron dose imaging, and in situ TEM techniques. We review recent advancements in atomic-resolution TEM studies involving small molecules, highlighting significant findings and methodological improvements that have emerged in the field. In addition, we offer an outlook in this realm, emphasizing the potential progress and innovations that could stimulate further scientific exploration.
In the quest for ever more precise observations of the microscopic world, transmission electron microscopy (TEM) was first conceptualized in the 1920s. However, it was not until the integration of aberration correctors that atomic resolution imaging became feasible. At the inception of TEM, aberrations limited the achievable spatial resolution to approximately 50 times the wavelength of the imaging electrons[30]. The most intuitive solution involved increasing acceleration voltage; however, this approach introduces significant challenges due to beam damage from high-energy electrons.
The advent of aberration correctors[31,32] has revolutionized TEM capabilities by improving point resolution to 0.13 nm at 200 keV[33]. In addition, the introduction of an aberration corrector has also made it possible to achieve atomic resolution using low-voltage TEM. Graphene, a classic electron-beam-sensitive material, sustains damage at voltages exceeding 80 keV[34]. In contrast, low-energy electrons minimize or even avoid damage. Another advantage of using low-energy electron beams is their enhanced interaction with the sample, leading to increased contrast - a crucial factor for 2D materials like graphene, which consists of only a single monolayer[35].
As shown in Figure 1A, the interactions between the high-energy electron beam and the thin specimen generate a variety of signals, including secondary electrons, auger electrons, backscattered electrons, characteristic X-rays, cathodoluminescence, and penetrated electron signals[36]. These signals arise from different types of scattering processes. Elastic scattering, which occurs when incident electrons are deflected by the Coulomb (electrostatic) interactions when passing close to other electrons or the positive nucleus of the specimen[37], produces electron diffraction patterns, diffraction contrast, and phase contrast in TEM images. On the other hand, inelastic scattering results from interactions between the incident electrons and the electrons surrounding atomic nuclei. This process leads to the emission of X-rays and provides valuable information through techniques like electron energy loss spectroscopy (EELS)[38].
Figure 1. Schematic diagrams showing interactions between high-energy electrons and thin specimens. (A) Various signals excited from interactions between electrons and specimens. (B-E) Mechanisms of electron beam damage..
Despite the wealth of information TEM provides, these electron-molecule interactions can also induce various forms of electron beam damage. This interaction, essential for imaging, often results in temporary or permanent structural damage to the specimen. As shown in Figure 1B-E, the damage usually includes radiolysis (the breakdown of molecules due to the absorption of radiation), knock-on displacement (where atoms are displaced from their positions), electrostatic charging (which can affect the imaging quality), and heating effects (which may alter the specimen's properties)[39]. Consequently, TEM study always requires careful consideration of these potential damage mechanisms, especially when imaging delicate small molecules.
Knock-on displacement occurs in imaging of larger or inorganic materials, and plays a less dominant role for small organic molecules in TEM. Radiolysis is the dominant damage mechanism for small organic molecules. When high-energy electrons interact with the molecules, they can break chemical bonds, leading to molecular fragmentation and the formation of free radicals. This fragmentation is often accompanied by the generation of secondary reactive species, such as ions and radicals, which can cause further molecular damage. In some cases, this can lead to significant molecular decomposition, distorting or even completely destroying the sample's original structure. Charge effect[40] occurs when dealing with poorly conductive small molecules, as the accumulated charge can cause aberrations in the electron beam, hindering accurate data collection and fine structural observation. Heating damage can cause the temperature of the sample to rise above ambient levels, potentially altering its properties and causing thermal degradation[38]. These various forms of electron-induced damage highlight the delicate balance required in TEM, where the high spatial resolution of the technique must be carefully managed to minimize the adverse effects on different samples. In the case of biomolecules, charging and heating can combine to locally soften the specimen and tear it apart through electrostatic forces. For small molecules, radiolysis remains the primary damage mechanism.
To minimize electron beam damage, several methods are proposed, particularly for small molecular samples: cryo-EM and low-dose imaging techniques.
CRYO-TEM
Cryo-TEM has revolutionized the study of biomolecules (usually macromolecules) by enabling high-resolution imaging at unprecedented levels of detail. Dubochet et al. invented cryo-EM to effectively reduce the damage caused by electron beams on soft materials[41], and were awarded the 2017 Nobel Prize[42] for their contributions. One of the most important points is the protection of the sample with amorphous ice. Obviously, keeping the sample at liquid nitrogen temperature significantly reduces the heating effect. Meanwhile, the amorphous ice can have a “cage” effect by preventing the dissociated fragments from flying away[43]. This approach does not directly mitigate radiolysis, but rather offers support through a mechanical stabilization mechanism[44].
One of the most critical aspects of cryo-EM is sample preparation, an extremely delicate process that requires strict environmental control during preparation, transfer, and microscopic analysis. The specific procedure for sample preparation is illustrated in Figure 2. The TEM grids should be cleaned prior to sample loading. This process aims to prevent contamination, which remains a persistent issue that undermines the high-resolution imaging capabilities of TEM. This problem becomes even more pronounced when conducting atomic-level observations of small molecules. Contamination is generated from the polymerization of carbon-rich organic molecules on the sample surface induced by the electron beam[45]. These molecules originate from the sample preparation process, storage conditions, or residual gases adsorbed inside the electron microscope. To suppress contamination, researchers have developed multiple methods, including baking[46], cooling[47], plasma cleaning[48], beam showering[49], and UV/ozone exposure[50]. Among these, beam showering and plasma cleaning are commonly the most frequently used methods. Plasma cleaning also makes the surface of the carbon film become hydrophilic. A hydrophilic surface helps the sample liquid to spread evenly, which is important for the subsequent formation of a vitreous ice layer[51]. Approximately 3 μL of liquid-phase soft materials are placed onto a TEM grid, and the excess is blotted off with filter paper, leaving a thin layer of sample on the grid (typically less than 1 μm). The grid then undergoes a plunge freezing process, typically by immersion in liquid ethane to vitrify the remaining sample liquid. The necessity of plunge freezing lies in the three-stage phase change of water, which involves a nucleation event that seeds the growth of ice crystals. The rate of cooling determines the speed and morphology of crystal growth. Slower freezing leads to the formation of hexagonal ice, while rapid freezing prevents the nucleation event, forming cubic ice instead. This process prevents large ice crystals from forming, which would otherwise expel solutes and distort imaging results[52]. This is precisely the reason for not choosing liquid nitrogen- liquid ethane or liquid propane have a higher thermal conductivity than liquid nitrogen and can freeze the sample more rapidly. Ideal cryo-EM specimen is encased in a uniformly thin, vitrified layer of ice, a state in which water is closest to its liquid state and causes the least damage to the structure. This form of ice is amorphous and allows electrons to penetrate the sample without interference with the crystalline ice.
Figure 2. A classical method of specimen loading (A), blotting (B), and plunge freezing process (C) of cryo-EM sample preparation.
Cryo-EM is a highly effective technique for mitigating electron beam damage, a major concern in transmission electron microscopy studies on molecule structures. In cryo-EM, the sample is plunge-frozen by immersion in liquid ethane, which preserves its native structure at cryogenic temperatures. This low-temperature environment not only reduces the thermal damage from the electron beam but also suppresses radiation effects that can otherwise distort or degrade the sample. Moreover, the low temperatures increase the tolerance of proteins and other biological macromolecules to electron beam exposure, enabling the acquisition of high-resolution molecular structures without excessive damage.
While biological macromolecules are the primary focus of cryo-EM, this technique can also be applied to small molecules, which are similarly vulnerable to electron beam damage. For example, Li et al. pioneered a method to image carbon dioxide (CO2)[53], one of the most common small molecules, at the atomic scale. They first dispersed ZIF-8 onto a TEM grid, which was then vacuum-dried to remove all solvents present in the pore. The samples were then maintained in 1 mbar CO2 environment to introduce CO2 molecules within the ZIF-8 pore cavities. Subsequently, plunge freezing was adopted to prepare the cryo-EM sample, which was then imaged at -170 °C with an electron dose of 4.5 e-/Å2/s. This approach successfully achieved atomic-resolution imaging of CO2 molecules, providing valuable insights into the interactions of carbon dioxide molecules within the ZIF-8 framework. Figure 3 presents the contrast transfer function (CTF)-corrected TEM images of ZIF-8 taken along the <111> zone axis after vacuum drying and after CO2 filling, respectively. The CTF correction is conducted with the assistance of a deep learning framework[54]. This procedure helps to eliminate the contrast reversal in TEM imaging, making the contrast of the image more consistent with the mass density of the sample. Figure 3B and D presents the magnified images of the box marked in Figure 3A and C, respectively. In Figure 3B, three regions of the pore exhibit higher contrast, which may correspond to the three organoimidazole linkers present within the pore. However, the contrast of these linkers is not shown in Figure 3D, indicating that the linkers rotate to create a narrower window of the six-membered ring, thereby facilitating the adsorption and diffusion of CO2 molecules. As is shown in Figure 3D, the contrast at the center of the unit cell (indicated by the red arrow) likely corresponds to the single CO2 adsorbed within ZIF-8.
Figure 3. Cryo-EM imaging of CO2 molecules[53]. (A) Cryo-EM image of vacuum-dried, empty ZIF-8 along the <111> projection. (B) Magnified image of a single ZIF-8 monocyte from (A). The density near the inner edge of the single cell (blue circle) may correspond to the organic imidazolate linkers. (C) Cryo-EM image of CO2-filled ZIF-8 particle along the <111> projection. (D) Magnified image of a single ZIF-8 unit cell from (C). Density at the center of the unit cell (indicated by the red arrow) likely corresponds to CO2 adsorbed within ZIF-8.
LOW DOSE IMAGING TECHNIQUES
Although research can achieve atomic resolution imaging of small molecules like CO2 by cryo-EM, we have to note the main shortcoming of cryo-EM is sample drifting that arises from the boiling of liquid nitrogen. The bubbles formed during the boiling process, along with their bursting, can cause slight vibrations of the sample holder and drift of the sample, reducing the resolution. Thus, in addition to cryo-EM, another approach to minimizing electron beam damage is utilized by directly reducing the electron dose for imaging[55]. However, reducing the electron dose inevitably gives rise to a number of issues, including a decline in resolution and a reduction in the signal-to-noise ratio. To address these challenges, we can explore two potential solutions. One potential avenue for improvement is to enhance the capabilities of the TEM detection system. Traditionally, charge-coupled devices (CCDs) have been widely used in TEM due to their automation capabilities for high-throughput data acquisition. CCDs, however, cannot be placed directly in the electron beam path due to their susceptibility to irradiation damage and signal saturation[56]. Instead, an optical fiber or lens system is employed to relay the signal to the CCD camera. Unfortunately, this electron-to-photon conversion limits the efficiency of CCD systems[57]. In contrast, the development of direct detection device (DDD) cameras bypasses this limitation by directly capturing electrons without the need for conversion to photons, thereby improving the spatial resolution of the imaging system[32]. Figure 4A and B illustrates the fundamental principles of CCD and DDD technologies. DDD cameras detect quanta several to several tens of times more efficiently than conventional CCD cameras[56,58,59].
Figure 4. Schematics showing the principles of CCD/DDD cameras and iDPC-STEM detector. (A and B) Schematics of CCD and DDD cameras. (C) Schematics of iDPC-STEM detector in which the positions of the four detectors marked a-d, respectively[67].
Carbon nanotubes (CNTs), as one-dimensional nanomaterials with hollow structures, provide an ideal spatial confinement environment for small molecules. Koshino et al. achieved TEM imaging of long-chain organic molecules by tethering C12 or C22 alkyl chains to carborane end groups and embedding them within single-walled carbon nanotubes (SWCNTs)[60], as shown in Figure 5A. This confinement strategy not only effectively suppresses the thermal motion of the molecules but also mitigates charging effects and thermal damage through the electrical conductivity of SWCNTs. When molecules are densely packed inside SWCNTs, their dynamic freedom is severely restricted, enabling accurate structural analysis of stationary molecules[61]. In addition to confining small molecules within SWCNTs, organic molecules can be anchored to the outer walls of carbon nanotubes via chemical strategies. For instance, Umeyama et al. covalently immobilized pyrene molecules onto SWCNT sidewalls through direct radical addition, forming pyrene dimers stabilized by π-π stacking interactions[62]. The planar geometry of pyrene facilitated strong electronic coupling with the SWCNTs’ surface, as corroborated by high-resolution transmission electron microscopy (HR-TEM) imaging shown in Figure 5B. This chemical immobilization strategy, combined with the mechanical robustness of SWCNTs, enabled atomic-scale visualization of dynamic molecular interactions under TEM. Despite the advantages of using CNTs as molecular confinement platforms, the imaging resolution in these studies remained constrained by the limitations of CCD cameras. To achieve higher-resolution imaging of beam-sensitive materials, researchers have increasingly turned to DDD cameras, which provide enhanced quantum efficiency and enable low-dose imaging. This advancement has proven particularly beneficial for investigating small molecules in highly electron-sensitive frameworks such as metal-organic frameworks (MOFs) and covalent organic frameworks (COFs).
Figure 5. TEM imaging on carbon nanotube, MOF, and COF materials. (A) Experimental TEM image, simulated image, and corresponding model of a hydrocarbon chain covalently attached to an ortho-carborane end group[60]. Scale bar, 1 nm. (B) Experimental TEM image, simulated image, and corresponding model of 4-(1-pyrenyl)phenyl (PP) radicals onto the SWNT sidewall (Py-1-SWNT)[62]. Scale bar, 1 nm. (C) CTF-corrected and denoised (using a Wiener filter) HRTEM image of ZIF-8 along the [111] axis[63]. (D) Symmetry-imposed and lattice-averaged image with a structural model of ZIF-8 embedded[63]. (E) CTF-corrected denoised image of UiO-66, showing triangular channels, individual Zr atomic columns, and the BDC linkers. The benzene rings in the BDC linkers are indicated by the arrows. Overlays are simulated projected potential maps and a projected structural model of UiO-66 for comparison[55]. (F) Low-magnification TEM image of two oriented, attached ZIF-8 crystals[63]. (G) CTF-corrected HRTEM image of the region within the green square in (F). Yellow circles highlight Zn triplets with obvious distortions[63]. (H) CTF-corrected and denoised HRTEM image of TPA-COF[64]. (I) Left: Lattice-averaged, P3-symmetry imposed and CTF-corrected HRTEM image. Right: Simulated projected potential map with a point spread function width of 4 Å, in which the projected structural model in green and unit cell in red are embedded[64].
MOFs are composed of metal ions and organic linkers connected via coordination bonds, while COFs consist of organic molecules linked by covalent bonds, and both of them are known to be highly sensitive to electron irradiation. The low-dose imaging capabilities of DDD cameras offer excellent quantum detection efficiency, enabling high-resolution imaging of the intrinsic structures of these materials with minimized electron beam damage. For example, Zhu et al. used a DDD camera to acquire TEM images of ZIF-8, one of the popular MOF materials at an ultra-low dose of 4.1 e-/Å2, and identified imidazole rings with two different configurations (edge-on/face-on) in the ZIF-8 structure, as shown in Figure 5C and D[63]. The atomic-scale images are used to interpret the self-assembly mechanism, surface termination modes, and interfacial structure of the two assembled ZIF-8 crystals, as shown in Figure 5E and F. Zhang et al. performed atomically resolved TEM imaging of several MOFs using a DDD camera[55]. CTF-corrected and denoised TEM images of UiO-66 reveal the arrangement of 1,4-benzenedicarboxylic acid (BDC) molecules within the framework, as shown in Figure 5G. The total dose was as low as 20 e-/Å2. The benzene ring and two carboxyl groups in BDC molecules are clearly identified in the TEM image. In addition, Peng et al. employed a DDD camera to capture low-dose TEM images of a novel imine-conjugated COF[64]. Atomic-scale images of Tris(4-aminophenyl)amine (TAPA) and tris(4-formylphenyl)amine (TFPA) are presented in
In addition to TEM imaging under parallel beam conditions, scanning transmission electron microscopy (STEM) is another widely utilized imaging mode. However, STEM encounters more significant challenges related to electron beam damage. This is primarily due to the convergent beam conditions employed in STEM, which can result in higher electron doses, often reaching levels as high as 104~106 e-/Å2[65]. The increased intensity of the focused electron beam can lead to greater material degradation, particularly in sensitive samples. Moreover, traditional STEM imaging often struggles with a low signal-to-noise ratio. This results in the necessity to generate a sufficient number of scattered electrons for effective imaging, which can be difficult to achieve at low dose rates. Researchers are continuously exploring strategies to enhance the imaging capabilities of STEM, such as employing advanced detectors, optimizing imaging parameters, and developing innovative sample preparation techniques that can withstand higher doses without compromising structural integrity.
One of the most recent advances in the observation of small molecules using scanning transmission electron microscopy (STEM) is the integrated differential phase contrast scanning transmission electron microscopy (iDPC-STEM) technique[66]. In iDPC-STEM imaging, incident focused electrons are deflected by the electromagnetic field generated by the sample, as shown in Figure 4C[67], and these deflected electrons are detected by a four-quadrant detector (DF4). By performing a two-dimensional integration of the movement information collected by four sections of the detector, researchers can accurately determine the positions and species of the atoms within the sample. In addition, the image contrast of high-angle annular dark-field scanning transmission electron microscopy (HAADF-STEM) imaging is approximately proportional to the square of the atomic number (Z2)[68-70], whereas the contrast of iDPC-STEM is typically proportional to the atomic number. This fundamental difference in contrast mechanisms allows the iDPC-STEM to provide superior contrast and sensitivity, especially when imaging both heavy and light elements. As a result, iDPC-STEM is particularly advantageous for imaging small molecules composed primarily of light elements such as hydrogen (H), carbon (C), and oxygen (O).
Shen et al. utilized iDPC-STEM to explore the orientation changes of para-xylene (PX) molecules within the ZSM-5 zeolite framework[71]. Zeolites, as porous materials, possess intricate channel systems and a high density of acidic sites, which play a crucial role in binding and transforming adsorbed molecules. The combination of these confined pore channels and reactive acidic sites creates a unique environment that limits the movement of small molecules, offering ideal conditions for atomic-scale imaging. In this study, PX molecules are utilized as a “pointer”, while the quasi-two-dimensional ZSM-5 framework serves as a “dial”, together conceptualizing a “single-molecule compass”. This analogy effectively illustrates how the highly structured and confined environment of the ZSM-5 zeolite facilitates precise control over the orientation of PX molecules within its channels. These orientations are influenced primarily by van der Waals interactions between the small molecules and the pore walls. Moreover, the variation in orientation was found to be closely tied to the geometry of the channels themselves, highlighting the intricate relationship between molecular structure and the spatial constraints imposed by the zeolite. These insights contribute to a deeper understanding of molecular behavior in confined environments and demonstrate the potential of iDPC-STEM as a powerful tool for studying molecular arrangements in complex porous materials.
To further explore molecular behaviors and interactions, Shen et al. optimized probe molecules and utilized iDPC-STEM to observe the adsorption and desorption of individual pyridine and thiophene molecules within the ZSM-5 zeolite framework[72]. This approach enabled them to directly image the structures of these small organic molecules and their interactions with the zeolite at the atomic level. Pyridine, a nitrogen-containing molecule, interacts with the Brønsted acid sites in ZSM-5 through acid-base interactions. The iDPC-STEM images of the empty straight channels of ZSM-5 and the channels after adsorption of saturated pyridine are shown in Figure 6A and B. The spindle-shaped features appearing in the channels reflect the pyridine columns (3-5 pyridines) along channels. Due to the high silica-aluminum ratio (~200) of the sample, the majority of pyridine molecules were adsorbed primarily through van der Waals forces, rather than through the expected acid-base interactions. To differentiate between these two types of adsorptions, the researchers heated the samples to 200 °C under vacuum. This treatment effectively distinguished the energy requirements for van der Waals interactions versus acid-base interactions. During this process, pyridine molecules adsorbed through van der Waals forces were removed, while those bound more strongly to the Brønsted acid sites remained intact. After this treatment, the samples were prepared for electron microscopy observation, allowing for the clear visualization of the adsorbed molecules. As depicted in Figure 6C and D, the iDPC-STEM image of a single pyridine molecule is presented, with its corresponding atomic structure model illustrated in Figure 6E. Figure 6F presents the simulated iDPC-STEM image generated from the model, demonstrating distinct behavioral patterns based on interaction types. Pyridine molecules adsorbed via van der Waals interactions exhibit a vertical orientation within the zeolite pores, whereas those adsorbed through acid-base interactions adopt a near-horizontal configuration. Furthermore, Figure 6G displays the intensity profile of the iDPC-STEM image along the direction indicated by the red arrow in Figure 6D, further unraveling the spatial arrangement of the molecules within the pores.
Figure 6. Application of iDPC-STEM imaging on small molecules confined in pores of zeolites[72]. (A) iDPC-STEM images of ZSM-5 empty straight channels. Scale bar, 1 nm. (B) Channels after saturated pyridine adsorption. Scale bar, 1 nm. (C) Magnified iDPC-STEM image of a near-horizontal pyridine with an obvious ring structure in the ZSM-5 channel. Scale bar, 0.5 nm. (D) Marks for the C or N atoms (red dots) and the positions of intensity profiles (red arrow). (E) Calculated structural model of pyridine in the ZSM-5 channel that is closest to the observation in (C). The acid site is set at O2. (F) Simulated iDPC-STEM image based on the model in (E). (G) Intensity profiles of the experimental (solid line) and simulated (dashed line) iDPC-STEM images. The positions of intensity profiles are given in (D). (H-K) Enlarged iDPC-STEM images of two thiophenes in the ZSM-5 channel showing clear ring structures and corresponding intensity profiles of S and C atoms with different intensities. The errors in the S position between the simulation model and the imaging results are shown in red and blue dots, respectively. Scale bar, 0.5 nm. (L) Schematic representation of thiophene binding to the acid sites.
The rationale for selecting thiophene as the probe molecule in this experiment is based on its advantageous imaging characteristics and interaction properties. The sulfur atom (atomic number 16) in thiophene is more readily imaged by electron microscopy compared to the carbon atom (atomic number 6) in para-xylene or the nitrogen atom (atomic number 7) in pyridine. This enhanced visibility is crucial for accurately studying molecular interactions at the atomic level. Furthermore, sulfur atoms exhibit strong interactions with the acidic sites in ZSM-5, allowing researchers to effectively localize a single sulfur atom to investigate the properties of these acidic sites. In contrast, the localization of all atoms in pyridine must be detected, which can complicate the analysis. This unique advantage makes thiophene molecules particularly effective for probing the distribution and mechanisms of acid sites within zeolite frameworks. The increased signal intensity from sulfur atoms in these images facilitates their identification within the ring structure of thiophene, providing clear visual evidence of their presence. Figure 6H displays the iDPC-STEM image capturing the interaction between each thiophene molecule and ZIF-8. An intensity profile along the direction of the red line in Figure 6H is presented in Figure 6I, while the intensity profile along the blue line in Figure 6J is marked in the corresponding color within Figure 6I. The intensity profiles illustrated in Figure 6I reveal significant differences between the peak data for sulfur and carbon atoms, highlighting the distinct imaging capabilities of thiophene. This distinction allows for precise localization and determination of sulfur atom positions, enabling researchers to account for computational errors and infer the configuration of the thiophene molecule with greater accuracy. Figure 6K illustrates the S atom position error map between the model of a single thiophene molecule in the ZSM-5 channel and the imaging results. Additionally, Figure 6L provides the schematic diagram of the bonding between thiophene and acidic sites within the ZSM-5 channel.
As highlighted in the above advancements, the porous materials (e.g., zeolites and MOFs) were widely selected as hosts to stabilize the small molecules for TEM imaging, with a particular focus on the confinement effect[73]. The unique structure of these materials, with their well-defined pores, plays a crucial role in stabilizing small molecules by minimizing their inherent thermal motion. This stabilization occurs not under cryogenic conditions but at ambient or even elevated temperatures, owing to the interactions between the small molecules and the hosts. In addition, it is notable that small molecules are typically composed of light elements, which results in relatively weak contrast for TEM observation. Reducing the background contrast caused by the substrate as much as possible can help identify small molecules in the TEM images. Spatial confinement within the pores or channels in porous materials can create a vacuum background for the small molecules, facilitating their identification. Table 1 summarizes the comparison of the different low-dose imaging techniques.
Comparison of different low-dose imaging techniques
Techniques | Applications | Principles | Characteristics |
Low-dose TEM | Cryo-TEM Room-temperature low-dose TEM | Physical protection by amorphous ice High detector efficiency of DDD camera | Difficulty in TEM image interpretation Prevention of knock-on, heating, and charging damage |
Low-dose STEM | iDPC-STEM | Lower electron dose rate High electron utilization Lower background noise | Advantageous in light element imaging Prevention of radiolysis |
IN SITU TEM
In addition to spatial confinement, surface adsorption confinement involves the adsorption of small molecules onto the substrate surfaces, which helps anchor the molecules in place. This method, usually combined with in situ TEM techniques, has proven highly effective for observing small molecules under varying conditions. A key tool in this approach is environmental transmission electron microscope (ETEM), which allows for real-time observation of small molecules introduced in the microscope chamber. For instance, Yoshida et al. utilized ETEM to observe CO molecules adsorbed at the on-top sites of the reconstructed Au particle surface at room temperature[74]. Under reaction conditions (1 volume % CO in air at 100 Pa), the Au nanoparticle underwent reconstruction on its (100) surface compared to that under vacuum condition, as illustrated in Figure 7A. In the reconstructed surface, the Au atoms on the topmost surface layer form an undulating hexagonal lattice, whereas those on the second topmost surface layer form a normal square lattice with slight distortion. This unique surface configuration allows for CO molecule absorption with higher surface coverage on Au (100) surface. As highlighted in Figure 7B and C, an unusual contrast was found on the reconstructed Au facet. Bright and dark contrast features emerged above the reconstructed Au facet, and the corresponding TEM image simulation [Figure 7D] based on an energetically favorable model for CO adsorption fits well with the observed image. The direct observation of an array of CO molecules confirms the strong adsorption behavior of CO on the reconstructed Au surface, while such behavior is not evident on the flat Au (100) surface[75,76]. Meanwhile, the interaction between the gas molecules and the metal surface also clarifies the configuration of the Au active sites relevant to the catalysis reactions such as CO oxidation.
Figure 7. Adsorbed CO molecules on reconstructed Au (100) surface under catalytic conditions[74]. Aberration-corrected ETEM images in (A) vacuum and (B) a reaction environment (1.0% CO in air at 100 Pa at room temperature) taken using 80 kV. (C) The observed image in the rectangular region in (B) at higher magnification. (D) A simulated TEM image based on an energetically favorable model for CO adsorption.
Yuan et al. employed in situ ETEM technique to visualize the absorption configuration and dynamics of
Figure 8. ETEM observations showing the dynamics of H2O molecules absorbed on TiO2 (1 × 4) (001) surface under gaseous environments[77]. (A) HAADF-STEM image of the TiO2 (1 × 4) (001) surface viewed along the [010] direction. The image was collected at 700 °C in vacuum. (B) Atomic structure of the adsorbed H2O molecules on TiO2 (1 × 4) (001) surface (gray, Ti; red, O; cyan, H). (C) ETEM images showing the same area of TiO2 (001) surface at 700 °C under oxygen and water vapor conditions, respectively. Scale bar, 1 nm. (D) Dynamic structural evolution of the TiO2 (1 × 4) (001) surface in the water-gas shift reaction. Scale bar, 2 nm. (E) Enlarged ETEM images of the dotted rectangle region in (D). Scale bar, 0.5 nm. (F) Intensity profiles along the lines across the Ti rows of (E) marked in the inset. Blue arrows denote intensity valleys corresponding to the twin protrusions.
In addition to gas-phase in ETEM, recent developments of in situ cell for gas and liquid have already made high-pressure gas in situ observation and liquid phase transmission electron microscopy (LP-TEM) accessible. The cells enclosed with Si3N4 are the most widely adopted due to their superior practicality and ease of operation. These cells can greatly enhance the pressure of gas and isolate the liquid sample from the TEM vacuum environment. Xiong et al. imaged benzene molecules adsorbed in ZSM-5 in a closed cell consisting of a Si3N4 membrane in an atmosphere of 600 mbar and 10% benzene[79]. The corresponding gas environments were 0.45 and 2.5 mbar for CO and H2O imaging, respectively. However, due to the thicker Si3N4 membranes[80], the enclosed cell faces more pronounced challenges in achieving high imaging resolution for small molecular imaging compared with open systems like ETEM. Similarly, although LP-TEM has been reported to achieve imaging of DNA molecules[81] as well as polymer molecules[82], it is still difficult to image small molecules in a liquid phase for the same reason. Strategies to mitigate this issue include using alternative window materials; for example, graphene-based cells have demonstrated reduced electron scattering and enhanced resolution compared to Si3N4[83].
OUTLOOK
Advanced TEM imaging has provided direct insights into the configuration and interactions of small molecules in confined environments. For example, these techniques have revealed how acid-base sites in ZSM-5 influence guest molecule orientations, such as the vertical or horizontal adsorption of pyridine, which directly affects adsorption selectivity and catalytic performance. Real-time TEM observations have also captured transient intermediates, such as H2O species, on TiO2 during catalysis. In situ TEM studies further demonstrate structural flexibility in porous materials, such as linkers’ variations in ZIF-8 during different gas adsorption, which influence molecular transport and sieving behavior. These findings not only deepen our understanding of molecular interactions at the atomic scale but also provide valuable guidance for experimental design. For instance, in zeolites like ZSM-5, where the acid-base properties are tuned by the Si/Al ratio, selecting appropriate probe molecules can help systematically study the impact of acid site distribution on adsorption and catalytic behavior. Similarly, real-time tracking of reaction intermediates can assist in identifying optimal active sites and reaction conditions, facilitating the rational design of materials with tailored catalytic properties. By integrating these insights, researchers can develop more effective strategies for probing host-guest interactions, optimizing adsorption processes, and enhancing reaction selectivity.
In addition to the several techniques mentioned in the main text, there are also imaging techniques that have great potential for small molecule imaging, such as optimum bright-field scanning transmission electron microscopy (OBF-STEM), event response imaging, and 4D-STEM. OBF-STEM addresses specific challenges in light-element visualization by integrating frequency filters optimized for STEM's contrast transfer function (CTF), enabling selective extraction of high-contrast, noise-resistant components. As stated in the main text, one of the difficulties of imaging small molecules is the background noise, which sometimes exceeds the contrast of the small molecules themselves. Additionally, OBF-STEM, by using a segmented detector, has a much higher imaging efficiency in the entire spatial frequency domain than conventional techniques such as ABF- and BF-STEM[84]. OBF-STEM has about two orders of magnitude higher imaging dose compared to conventional STEM imaging methods, and is approximately 24% higher than that of iDPC-STEM[85]. Theoretically, the application of OBF-STEM to small molecule imaging is promising for obtaining better experimental images.
The precise control of the electron beam dose is another noteworthy advancement in TEM imaging.
Another significant advancement in TEM imaging is the development of 4D-STEM. This technique involves the collection of a two-dimensional diffraction pattern at each scanning position, resulting in a comprehensive dataset that captures extensive information about electron scattering[87]. Each dataset generated through this method can include anywhere from ten thousand to one million individual diffraction patterns, highlighting the method's capacity for detailed analysis[88]. Based on this vast amount of data, state-of-the-art ptychography algorithms enable the recovery of the electron probe function and elucidate the electron scattering processes occurring within the sample. This capability is crucial for resolving residual aberrations and enhances the spatial resolution of aberration-corrected electron microscopy. For example, Jiang et al. achieved a resolution of 39 pm in imaging single-atom defects in MoS2 in 2018[89]. Subsequently, Chen et al. reached a resolution of 23 pm in the characterization of PrScO3 in 2021[90]. In this year, Yang et al. further pushed the resolution limit to 14 pm[91]. Recent advancements in electron ptychography have also demonstrated a resolution of 44 pm[92] even in uncorrected electron microscopes. In addition, the reconstruction results obtained through electron ptychography provide depth resolution along the direction of electron beam propagation. This feature facilitates the effective removal of surface damage layers, allowing for the extraction of intrinsic structural information from the sample[93]. Another notable advantage of electron ptychography is its high dose efficiency. Since the detector captures nearly all scattered electrons, this technique minimizes electron irradiation damage, making it particularly well-suited for low-dose imaging applications, including biomaterials[94,95] and crystalline materials[96-98]. We anticipate that electron ptychography offers a promising solution to the challenges associated with microstructural analysis of small molecules, such as the detailed arrangement of functional optoelectronic molecules, as discussed in the introduction part, and the determination of the three-dimensional spatial configuration of non-planar small molecules. Such analysis is crucial for understanding the structural properties and behavior of these molecules, particularly in applications where precise molecular orientation and interaction are key factors, such as in organic semiconductors, photovoltaic devices, and other optoelectronic materials.
CONCLUSIONS
In this review article, we present recent advancements in real-space imaging of small molecules using advanced TEM techniques. As summarized, the current challenges associated with imaging small molecules can be primarily attributed to two key factors.
1. Minimizing electron beam damage on small molecules. Small molecules exhibit a pronounced sensitivity to electron beams, which can result in substantial damage during imaging processes. To address this critical issue, a variety of strategies have been designed to minimize beam-induced damage while maintaining the quality of the images obtained. One effective method is cryo-EM, which preserves the structural integrity of samples by imaging them at cryogenic temperatures. Additionally, low electron dose imaging techniques have been developed to further reduce the influence of the electron beam. For instance, TEM imaging utilizing DDD cameras enables high-quality imaging with reduced electron exposure. STEM imaging employing iDPC-STEM techniques minimizes electron beam intensity while achieving a favorable signal-to-noise ratio.
2. Stabilizing small molecules onto suitable hosts. In addition to cryogenic freezing, effectively immobilizing small molecules onto suitable hosts is crucial for TEM observation, particularly at ambient or even higher temperatures. One promising approach involves spatial confinement within pores and channels of porous materials (e.g., zeolites, MOFs, and COFs), minimizing the inherent thermal motion of the small molecules and enhancing their stability during observation. Another effective method is surface absorption on hosts under the catalysis reaction environment, which is particularly realized when utilizing in situ ETEM. Both these confinement strategies not only stabilize the small molecules on their respective hosts but also create a vacuum background that facilitates the identification of these molecules, even when they exhibit relatively weak contrast.
The real-space visualization of small molecules at atomic resolution has emerged as a rapidly evolving and captivating frontier in materials characterization. This burgeoning field, fueled by remarkable advancements in TEM instrumentations and innovative methodologies, is poised to revolutionize our understanding of molecular structures and dynamics. The current technological breakthroughs, coupled with the integration of artificial intelligence and machine learning for image processing and analysis, suggest that the field is on the verge of transformative discoveries. Future developments are expected to encompass not only enhanced spatial resolution but also the ability to probe molecular dynamics in real time, characterize chemical bonds with greater precision, and investigate molecular behavior under various environmental conditions.
DECLARATIONS
Authors’ contributions
Supervision and draft preparation: Dai, S.
Editing and reference preparation: Xie, S.
Availability of data and materials
Not applicable.
Financial support and sponsorship
This work was supported by the National Natural Science Foundation of China (22376062), the Science and Technology Commission of Shanghai Municipality (24DX1400200 and 22ZR1415700), and the Fundamental Research Funds for the Central Universities.
Conflicts of interest
Both authors declared that there are no conflicts of interest.
Ethical approval and consent to participate
Not applicable.
Consent for publication
Not applicable.
Copyright
© The Author(s) 2025.
REFERENCES
1. Chen, W.; Wunderlich, B. Nanophase separation of small and large molecules. Macromol. Chem. Phys. 1999, 200, 283-311.
2. Erickson, H. P. Size and shape of protein molecules at the nanometer level determined by sedimentation, gel filtration, and electron microscopy. Biol. Proced. Online. 2009, 11, 32-51.
3. Kong, G.; Liu, R.; Lu, J.; Che, C.; Zhong, Z. Insight for microstructure research of materials. Acta. Metall. Sin. 2010, 46, 487-93.
5. Niemeyer, C. M. Nanoparticles, proteins, and nucleic acids: biotechnology meets materials science. Angew. Chem. Int. Ed. 2001, 40, 4128-58.
6. De Clercq, P. We need to talk about Kekule: The 150th anniversary of the benzene structure. Eur. J. Org. Chem. 2022, 2022, e202200171.
7. Watson, J. D.; Crick, F. H. C. Molecular structure of nucleic acids: a structure for deoxyribose nucleic acid. JAMA. 1993, 269, 1966-7.
8. Bai, X. C.; McMullan, G.; Scheres, S. H. How cryo-EM is revolutionizing structural biology. Trends. Biochem. Sci. 2015, 40, 49-57.
9. Huang, Y.; Yang, C.; Xu, X. F.; Xu, W.; Liu, S. W. Structural and functional properties of SARS-CoV-2 spike protein: potential antivirus drug development for COVID-19. Acta. Pharmacol. Sin. 2020, 41, 1141-9.
10. Olsbye, U.; Svelle, S.; Lillerud, K. P.; et al. The formation and degradation of active species during methanol conversion over protonated zeotype catalysts. Chem. Soc. Rev. 2015, 44, 7155-76.
11. Dominguez-Soria, V. D.; Calaminici, P.; Goursot, A. Theoretical study of host-guest interactions in the large and small cavities of MOR zeolite models. J. Phys. Chem. C. 2011, 115, 6508-12.
12. Dias, D. A.; Jones, O. A.; Beale, D. J.; et al. Current and future perspectives on the structural identification of small molecules in biological systems. Metabolites 2016, 6, 46.
13. Franklin, R. E.; Gosling, R. G. Molecular configuration in sodium thymonucleate. Nature 1953, 171, 740-1.
14. Cichocka, M. O.; Ångström, J.; Wang, B.; Zou, X.; Smeets, S. High-throughput continuous rotation electron diffraction data acquisition via software automation. J. Appl. Crystallogr. 2018, 51, 1652-61.
15. Zhang, Y. B.; Su, J.; Furukawa, H.; et al. Single-crystal structure of a covalent organic framework. J. Am. Chem. Soc. 2013, 135, 16336-9.
16. Wang, B.; Rhauderwiek, T.; Inge, A. K.; et al. A Porous cobalt tetraphosphonate metal-organic framework: accurate structure and guest molecule location determined by continuous-rotation electron diffraction. Chemistry 2018, 24, 17429-33.
17. Morgan, T. J.; George, A.; Davis, D. B.; Herod, A. A.; Kandiyoti, R. O Optimization of 1H and 13C NMR methods for structural characterization of acetone and pyridine soluble/insoluble fractions of a coal tar pitch. Energy. Fuels. 2008, 22, 1824-35.
18. Kwan, A. H.; Mobli, M.; Gooley, P. R.; King, G. F.; Mackay, J. P. Macromolecular NMR spectroscopy for the non-spectroscopist. FEBS. J. 2011, 278, 687-703.
19. Singh, K. S.; Majik, M. S.; Tilvi, S. Chapter 6 - Vibrational spectroscopy for structural characterization of bioactive compounds. Compr. Anal. Chem. 2014, 65, 115-48.
20. Zhang, Z.; Chen, C. L.; Chen, Y. A.; et al. Tuning the conformation and color of conjugated polyheterocyclic skeletons by installing ortho-methyl groups. Angew. Chem. Int. Ed. 2018, 57, 9880-4.
21. Hong, T.; Yin, J. Y.; Nie, S. P.; Xie, M. Y. Applications of infrared spectroscopy in polysaccharide structural analysis: progress, challenge and perspective. Food. Chem. X. 2021, 12, 100168.
22. Cai, Z.; Kumar, N.; Zenobi, R. Probing on-surface chemistry at the nanoscale using tip-enhanced raman spectroscopy. CCS. Chem. 2023, 5, 55-71.
23. Park, H.; DiMaio, F.; Baker, D. The origin of consistent protein structure refinement from structural averaging. Structure 2015, 23, 1123-8.
25. Wang, Y.; Zhang, X.; Xu, J.; et al. The development of microscopic imaging technology and its application in micro- and nanotechnology. Front. Chem. 2022, 10, 931169.
26. Tanaka, N. Present status and future prospects of spherical aberration corrected TEM/STEM for study of nanomaterials. Sci. Technol. Adv. Mater. 2008, 9, 014111.
27. Guzzinati, G.; Altantzis, T.; Batuk, M.; et al. Recent advances in transmission electron microscopy for materials science at the EMAT lab of the university of Antwerp. Materials 2018, 11, 1304.
28. Rauch, E. F.; Véron, M.; Nicolopoulos, S.; Bultreys, D. Orientation and phase mapping in TEM microscopy (EBSD-TEM like): applications to materials science. Solid. State. Phenomena. 2012, 186, 13-5.
29. Pennycook, S. J.; Chisholm, M. F.; Lupini, A. R.; et al. Aberration-corrected scanning transmission electron microscopy: from atomic imaging and analysis to solving energy problems. Philos. Trans. A. Math. Phys. Eng. Sci. 2009, 367, 3709-33.
30. Scherzer, O. The theoretical resolution limit of the electron microscope. J. App. Phys. 1949, 20, 20-9.
31. Krivanek, O. L.; Corbin, G. J.; Dellby, N.; et al. An electron microscope for the aberration-corrected era. Ultramicroscopy 2008, 108, 179-95.
32. Spence, J. The future of atomic resolution electron microscopy for materials science. Mater. Sci. Eng. R. Rep. 1999, 26, 1-49.
33. Haider, M.; Uhlemann, S.; Schwan, E.; Rose, H.; Kabius, B.; Urban, K. Electron microscopy image enhanced. Nature 1998, 392, 768-9.
34. Rummeli, M. H.; Ta, H. Q.; Mendes, R. G.; et al. New frontiers in electron beam-driven chemistry in and around graphene. Adv. Mater. 2019, 31, e1800715.
35. Bachmatiuk, A.; Zhao, J.; Gorantla, S. M.; et al. Low voltage transmission electron microscopy of graphene. Small 2015, 11, 515-42.
36. Gong, X.; Gnanasekaran, K.; Chen, Z.; et al. Insights into the structure and dynamics of metal-organic frameworks via transmission electron microscopy. J. Am. Chem. Soc. 2020, 142, 17224-35.
37. Williams, D. B.; Carter, C. B. Transmission electron microscopy: a textbook for materials science; New York, NY: Springer, 2009.
39. Egerton, R. F.; Li, P.; Malac, M. Radiation damage in the TEM and SEM. Micron 2004, 35, 399-409.
40. Ding, Z. J.; Li, C.; Da, B.; Liu, J. Charging effect induced by electron beam irradiation: a review. Sci. Technol. Adv. Mater. 2021, 22, 932-71.
41. Dubochet, J.; Adrian, M.; Chang, J. J.; et al. Cryo-electron microscopy of vitrified specimens. Q. Rev. Biophys. 1988, 21, 129-228.
42. Chari, A.; Stark, H. Prospects and limitations of high-resolution single-particle cryo-electron microscopy. Annu. Rev. Biophys. 2023, 52, 391-411.
43. Li, Y.; Kang, D.; Dai, J.; Wang, L. The cage effect of electron beam irradiation damage in cryo-electron microscopy. NPJ. Comput. Mater. 2024, 10, 1299.
44. Henderson, R.; Clarke, B. C. Cryo-protection of protein crystals against radiation damage in electron and X-ray diffraction. Proc. R. Soc. Lond. B. 1990, 241, 6-8.
45. Hugenschmidt, M.; Adrion, K.; Marx, A.; Müller, E.; Gerthsen, D. Electron-beam-induced carbon contamination in STEM-in-SEM: quantification and mitigation. Microsc. Microanal. 2023, 29, 219-34.
46. McGilvery, C. M.; Goode, A. E.; Shaffer, M. S.; McComb, D. W. Contamination of holey/lacey carbon films in STEM. Micron 2012, 43, 450-5.
47. Egerton, R. F.; Rossouw, C. J. Direct measurement of contamination and etching rates in an electron beam. J. Phys. D. Appl. Phys. 1976, 9, 659-63.
48. Zaluzec, N. J.; Kestel, B. J.; Henriks, D. Reactive gas plasma specimen processing for use in microanalysis and imaging in analytical electron microscopy. Microsc. Microanal. 1997, 3, 983-4.
49. Hettler, S.; Dries, M.; Hermann, P.; Obermair, M.; Gerthsen, D.; Malac, M. Carbon contamination in scanning transmission electron microscopy and its impact on phase-plate applications. Micron 2017, 96, 38-47.
50. Mitchell, D. R. Contamination mitigation strategies for scanning transmission electron microscopy. Micron 2015, 73, 36-46.
51. Dobro, M. J.; Melanson, L. A.; Jensen, G. J.; Mcdowall, A. W. Chapter three - plunge freezing for electron cryomicroscopy Methods Enzymol 2010. pp. 63-82.
52. Hurbain, I.; Sachse, M. The future is cold: cryo-preparation methods for transmission electron microscopy of cells. Biol. Cell. 2011, 103, 405-20.
53. Li, Y.; Wang, K.; Zhou, W.; et al. Cryo-EM structures of atomic surfaces and host-guest chemistry in metal-organic frameworks. Matter 2019, 1, 428-38.
54. Lee, J.; Lee, Y.; Kim, J.; Lee, Z. Contrast transfer function-based exit-wave reconstruction and denoising of atomic-resolution transmission electron microscopy images of graphene and Cu single atom substitutions by deep learning framework. Nanomaterials 2020, 10, 1977.
55. Zhang, D.; Zhu, Y.; Liu, L.; et al. Atomic-resolution transmission electron microscopy of electron beam-sensitive crystalline materials. Science 2018, 359, 675-9.
56. Milazzo, A. C.; Leblanc, P.; Duttweiler, F.; et al. Active pixel sensor array as a detector for electron microscopy. Ultramicroscopy 2005, 104, 152-9.
57. Faruqi, A. R.; Mcmullan, G. Direct imaging detectors for electron microscopy.In: Nuclear instruments and methods in physics research section a: accelerators, spectrometers, detectors and associated equipment; 2018, pp. 180-90.
58. Kuijper, M.; van Hoften, G.; Janssen, B.; et al. FEI's direct electron detector developments: Embarking on a revolution in cryo-TEM. J. Struct. Biol. 2015, 192, 179-87.
59. Levin, B. D. A. Direct detectors and their applications in electron microscopy for materials science. J. Phys. Mater. 2021, 4, 042005.
60. Koshino, M.; Tanaka, T.; Solin, N.; Suenaga, K.; Isobe, H.; Nakamura, E. Imaging of single organic molecules in motion. Science 2007, 316, 853.
61. Skowron, S. T.; Chamberlain, T. W.; Biskupek, J.; Kaiser, U.; Besley, E.; Khlobystov, A. N. Chemical reactions of molecules promoted and simultaneously imaged by the electron beam in transmission electron microscopy. ACC. Chem. Res. 2017, 50, 1797-807.
62. Umeyama, T.; Baek, J.; Sato, Y.; et al. Molecular interactions on single-walled carbon nanotubes revealed by high-resolution transmission microscopy. Nat. Commun. 2015, 6, 7732.
63. Zhu, Y.; Ciston, J.; Zheng, B.; et al. Unravelling surface and interfacial structures of a metal-organic framework by transmission electron microscopy. Nat. Mater. 2017, 16, 532-6.
64. Peng, Y.; Huang, Y.; Zhu, Y.; et al. Ultrathin two-dimensional covalent organic framework nanosheets: preparation and application in highly sensitive and selective DNA detection. J. Am. Chem. Soc. 2017, 139, 8698-704.
65. Egerton, R. F. Radiation damage to organic and inorganic specimens in the TEM. Micron 2019, 119, 72-87.
66. Lazić, I.; Bosch, E. G. T.; Lazar, S. Phase contrast STEM for thin samples: integrated differential phase contrast. Ultramicroscopy 2016, 160, 265-80.
67. Lin, Y.; Zhou, M.; Tai, X.; Li, H.; Han, X.; Yu, J. Analytical transmission electron microscopy for emerging advanced materials. Matter 2021, 4, 2309-39.
68. Bosch, E. G.; Lazić, I. Analysis of HR-STEM theory for thin specimen. Ultramicroscopy 2015, 156, 59-72.
69. Lazić, I.; Bosch, E. G. T. Chapter Three - Analytical review of direct stem imaging techniques for thin samples Adv Imaging Electron Phys 2017. pp. 75-184.
70. Cowley, J. M. Scanning transmission electron microscopy of thin specimens. Ultramicroscopy 1976, 2, 3-16.
71. Shen, B.; Chen, X.; Wang, H.; et al. A single-molecule van der Waals compass. Nature 2021, 592, 541-4.
72. Shen, B.; Wang, H.; Xiong, H.; et al. Atomic imaging of zeolite-confined single molecules by electron microscopy. Nature 2022, 607, 703-7.
73. Feng, J.; Ma, M.; Song, B.; Shen, B. Atomic single-molecule imaging by the confinement methods in advanced microscopy. Fundam Res 2024.
74. Yoshida, H.; Kuwauchi, Y.; Jinschek, J. R.; et al. Visualizing gas molecules interacting with supported nanoparticulate catalysts at reaction conditions. Science 2012, 335, 317-9.
75. Molina, L. M.; Hammer, B. Theoretical study of CO oxidation on Au nanoparticles supported by MgO(100). Phys. Rev. B. 2004, 69, 155424.
77. Yuan, W.; Zhu, B.; Li, X. Y.; et al. Visualizing H2O molecules reacting at TiO2 active sites with transmission electron microscopy. Science 2020, 367, 428-30.
78. Panagiotopoulou, P.; Kondarides, D. I. Effect of morphological characteristics of TiO2-supported noble metal catalysts on their activity for the water-gas shift reaction. J. Catal. 2004, 225, 327-36.
79. Xiong, H.; Liu, Z.; Chen, X.; et al. In situ imaging of the sorption-induced subcell topological flexibility of a rigid zeolite framework. Science 2022, 376, 491-6.
80. de Jonge, N.; Poirier-Demers, N.; Demers, H.; Peckys, D. B.; Drouin, D. Nanometer-resolution electron microscopy through micrometers-thick water layers. Ultramicroscopy 2010, 110, 1114-9.
81. Wang, H.; Li, B.; Kim, Y. J.; Kwon, O. H.; Granick, S. Intermediate states of molecular self-assembly from liquid-cell electron microscopy. Proc. Natl. Acad. Sci. USA. 2020, 117, 1283-92.
82. Nagamanasa, K. H.; Wang, H.; Granick, S. Liquid-cell electron microscopy of adsorbed polymers. Adv. Mater. 2017, 29, 1703555.
83. Dahmke, I. N.; Verch, A.; Hermannsdörfer, J.; et al. Graphene liquid enclosure for single-molecule analysis of membrane proteins in whole cells using electron microscopy. ACS. Nano. 2017, 11, 11108-17.
84. Findlay, S. D.; Shibata, N.; Sawada, H.; Okunishi, E.; Kondo, Y.; Ikuhara, Y. Dynamics of annular bright field imaging in scanning transmission electron microscopy. Ultramicroscopy 2010, 110, 903-23.
85. Ooe, K.; Seki, T.; Yoshida, K.; et al. Direct imaging of local atomic structures in zeolite using optimum bright-field scanning transmission electron microscopy. Sci. Adv. 2023, 9, eadf6865.
86. Peters, J. J. P.; Reed, B. W.; Jimbo, Y.; et al. Event-responsive scanning transmission electron microscopy. Science 2024, 385, 549-53.
87. Ophus, C. Four-dimensional scanning transmission electron microscopy (4D-STEM): from scanning nanodiffraction to ptychography and beyond. Microsc. Microanal. 2019, 25, 563-82.
88. Yu, R.; Sha, H.; Cui, J.; Yang, W. Introduction to electron ptychography for materials scientists. Microstructures 2024, 4, 2024056.
89. Jiang, Y.; Chen, Z.; Han, Y.; et al. Electron ptychography of 2D materials to deep sub-ångström resolution. Nature 2018, 559, 343-9.
90. Chen, Z.; Jiang, Y.; Shao, Y. T.; et al. Electron ptychography achieves atomic-resolution limits set by lattice vibrations. Science 2021, 372, 826-31.
91. Yang, W.; Sha, H.; Cui, J.; Mao, L.; Yu, R. Local-orbital ptychography for ultrahigh-resolution imaging. Nat. Nanotechnol. 2024, 19, 612-7.
92. Nguyen, K. X.; Jiang, Y.; Lee, C. H.; et al. Achieving sub-0.5-angstrom-resolution ptychography in an uncorrected electron microscope. Science 2024, 383, 865-70.
93. Gao, S.; Wang, P.; Zhang, F.; et al. Electron ptychographic microscopy for three-dimensional imaging. Nat. Commun. 2017, 8, 163.
94. Zhou, L.; Song, J.; Kim, J. S.; et al. Low-dose phase retrieval of biological specimens using cryo-electron ptychography. Nat. Commun. 2020, 11, 2773.
95. Pei, X.; Zhou, L.; Huang, C.; et al. Cryogenic electron ptychographic single particle analysis with wide bandwidth information transfer. Nat. Commun. 2023, 14, 3027.
96. Sha, H.; Cui, J.; Li, J.; et al. Ptychographic measurements of varying size and shape along zeolite channels. Sci. Adv. 2023, 9, eadf1151.
97. Pennycook, T. J.; Martinez, G. T.; Nellist, P. D.; Meyer, J. C. High dose efficiency atomic resolution imaging via electron ptychography. Ultramicroscopy 2019, 196, 131-5.
Cite This Article
How to Cite
Download Citation
Export Citation File:
Type of Import
Tips on Downloading Citation
Citation Manager File Format
Type of Import
Direct Import: When the Direct Import option is selected (the default state), a dialogue box will give you the option to Save or Open the downloaded citation data. Choosing Open will either launch your citation manager or give you a choice of applications with which to use the metadata. The Save option saves the file locally for later use.
Indirect Import: When the Indirect Import option is selected, the metadata is displayed and may be copied and pasted as needed.
About This Article
Copyright
Data & Comments
Data
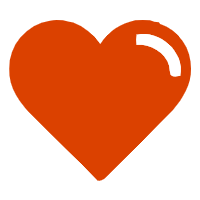
Comments
Comments must be written in English. Spam, offensive content, impersonation, and private information will not be permitted. If any comment is reported and identified as inappropriate content by OAE staff, the comment will be removed without notice. If you have any queries or need any help, please contact us at [email protected].