Tailoring the microstructure of lead-free KNN piezoelectric ceramics for force-sensitive smart windows
Abstract
Ferro/piezoelectric transparent materials with both excellent piezoelectricity and transparency are of great interest for irreplaceable applications in numerous fields such as photoacoustic imaging and transparent robots. Nevertheless, developing a lead-free ferroelectric ceramic that meets both distinct transparency and high piezoelectricity has always been challenging. Herein, the simple single Sm3+ doped KNN ferroelectric ceramics
Keywords
INTRODUCTION
Following the heightened emphasis on environmental protection and energy conservation, substantial progress has been achieved in the reasonable utilization of energy and the development of energy-saving products. Within this context, electrochromic materials were first designed for application in energy-saving lighting automatic control systems, including buildings, cars, and aerospace, forming “smart windows” that can adjust the transmittance of solar radiation in real time. Subsequently, smart windows related to photochromism and thermochromism gradually emerged[1-5]. Actually, in addition to windows with intelligent color regulation, windows that can intelligently sense signals such as temperature and force in the surrounding environment without changing their transparency are also urgently needed products in the high-tech era.
Owing to the unique combination of transparency, mechanical strength, and ferroelectric/piezoelectric/dielectric properties, transparent ferro/piezoelectric materials can be used not only in traditional energy harvesters, ultrasonic transducers, and microdisplacements[6], but also show great developmental advantages and potentials in high-tech photoacoustic imaging sensors, electro-optical devices, transparent robots, and even force-sensitive smart windows[7-10]. The PMN-PT crystals are representative ferro/piezoelectric transparent materials. Although their transparency is relatively poor because of their complex and disordered domain structure, they have attracted massive attention for their high piezoelectricity near the morphotropic phase boundaries (MPBs)[11,12]. In 2020, Qiu et al. showed that for the rhombohedral PMN-PT crystals with [001] orientation, repeated polarization by alternating-current (AC) electric fields can effectively eliminate the light scattering from 71° domain walls, achieving both near-saturation optical transmittance and ultrahigh piezoelectricity[13]. Nevertheless, considering the difficulty of preparing single-crystal materials and the human health issues caused by the toxicity of lead, the development of low-cost and high-performance lead-free environmentally friendly ferro/piezoelectric transparent polycrystalline ceramics is urgently needed[14,15].
The influence on the optical transmittance of ceramics can be generally summed up as following aspects[16,17]. Firstly, powders with high purity, uniform particle size distribution, and appropriate pre-sintering temperature are prerequisites for preparing transparent ceramics. Secondly, in order to minimize light scattering losses, it is necessary to prevent the formation of obvious impurity phases, macroscopic pores and defects inside the ceramic. Suitable doping concentrations, as well as optimal heating/cooling rates, sintering temperature, and holding time, are crucial for achieving high-density and pure-phase ceramics during sintering. Additionally, the light scattering theory suggests that when the scale of the scattering center is equivalent to the wavelength of the incident light, it will result in the strongest scattering and weakest optical transmittance. Hence, regulating grain size to deviate from the wavelength range of incident light has been widely used to ameliorate the optical transmittance of ceramics in the visible light region. Last but not least, the surface roughness of ceramics also directly affects their optical transmittance, so the surface treatment needs to be carried out by polishing technology to more accurately reflect the true transparency of the sample. To date, potassium sodium niobate (K0.5Na0.5NbO3, KNN)-based ceramics are the most promising candidates for the preparation of high-transparency functional ceramics and have been extensively studied in comparison with other representative lead-free ferro/piezoceramics, such as bismuth sodium titanate (BNT)-based, barium titanate (BT)-based, and bismuth ferrite (BiFeO3)-based ceramics[15-17].
The transparency of (1-x)K0.5Na0.5NbO3-xSrTiO3 ceramics was first discovered and reported by Kosec et al. as early as 2004[18], but it was not until 2008 that KNN-based transparent ceramics gradually attracted people's attention[15-17,19]. For example, Li et al. reported the Li/Bi comodified KNN-based ceramics with high optical transmittance of 60%-70% (near-IR region) and considerable electro-optic response
Rare-earth (RE) ion doping is an extremely effective strategy for optimizing both optical transmittance and electrical properties in KNN-based ferro/piezoceramics. Here, x mol% Sm3+-doped K0.5Na0.5NbO3 (abbreviated as xSm) ferroelectric transparent ceramics with simultaneously superior transparency, high Curie temperature, and reasonable piezoelectricity were successfully developed via the simple single RE ion doping strategy, and their optical/electrical properties and the multidimensional microstructure were also systematically studied. After appropriate Sm doping, the xSm ceramics possess a dense structure, finer grain size, more highly symmetric pseudo-cubic phase, and higher bandgap, thereby demonstrating prominent transparency. It is worth noting that although the doping of Sm also enhances the relaxor behavior of KNN ceramics and weakens the piezoelectricity, reasonable piezoelectricity can still be obtained in the xSm transparent ceramics with appropriate Sm doping, while not significantly affecting the Curie temperature. Ultimately, high transparency, high Curie temperature, and good piezoelectricity were simultaneously achieved in the 1.0 Sm ceramic. Leveraging the piezoelectric effect, the 1.0 Sm transparent ceramic was developed to exhibit excellent circulable electrical signal output characteristics under low pressure, demonstrating outstanding force response behavior. This innovation conceptualizes the application of smart windows that require real-time pressure detection [Figure 1].
MATERIALS AND METHODS
Sample preparation: A series of x mol% Sm3+-doped K0.5Na0.5NbO3 (0 ≤ x ≤ 3, abbreviated to xSm) ceramics were prepared via conventional solid-state reaction method and pressureless sintering with high-purity raw materials, including Na2CO3, K2CO3, Nb2O5, and Sm2O3. The powder mixtures weighed according to the formula were first ball milled with ethyl alcohol and ZrO2 balls for 12 h, then successively dried and calcined (850 °C/4 h). After being ball-milled again for 12 h and dried, the calcined powers were granulated through the 6 wt% polyvinyl alcohol (PVA) aqueous adhesive, and then pressed under uniaxial pressure of
Characterization: UV-Vis-IR spectroscopy (PerkinElmer Lambda 950) was used to characterize the optical transmittance of the ceramics. Photoluminescence (PL) spectra were measured via the spectrofluorometer (FluoroMax-4). The crystal structure for the samples at room temperature was recorded via X-ray diffraction (XRD) analysis (Rigaku Ultima III). The surface/cross-section and distribution of elements for the ceramics were measured by field emission scanning electron microscopy (FESEM, Zeiss Supra 55, Germany) and EDS (Oxford X-Max50), respectively. In addition, 350 grains were randomly selected from the SEM micrographs using the “Nano Measurer” software to determine the average grain size and corresponding distribution. Density was recorded according to the Archimedes method. After polarization with a DC electric field (20-30 kV cm-1) at 90 °C, the piezoelectric coefficient (d33) was measured at room temperature by the quasi-static d33 meter (ZJ-6A, Institute of Acoustics, China). Polarization (P-E) and strain (S-E) hysteresis loops were measured using a ferroelectric test system (Precision Premier II) at
Simulation method: For accurate band structure simulations, density functional theory (DFT) simulations were performed via the Vienna ab initio simulation package (VASP) in combination with the projector augmented-wave (PAW) pseudopotentials of generalized gradient approximations (GGA) in the Perdew-Burke-Ernzerhof (PBE) functional form. The modified shell-GGA-1/2 (shGGA-1/2) was introduced for electronic structure calculations to conquer the inherent band gap underestimation problem of DFT. To closely simulate the dopant atoms (i.e., Sm) and 1:1 randomly occupations of K/Na atoms at the A site in KNN, a 4 × 4 × 3 orthorhombic-phase ABO3 supercell including 240 atoms was built by using the special quasi-random structure (SQS). The GPU-accelerated VASP was used for all the calculations, and the Γ centered 2 × 2 × 3 K-point set and 600 eV cutoff energy were chosen. Meanwhile, the relaxation convergence for ions and electrons was 1 × 10-5 and 1 × 10-6 eV, respectively. In addition, the self-energy correction was carried out on O anions, with a self-energy potential derived from V(O: 2s2 2p4)-
RESULTS AND DISCUSSION
Transparency of the xSm ceramics
Figure 2A shows the photos of the investigated samples that have been thinned and polished to ~0.3 mm. It can be noticed that with the increase in Sm, the milky white opaque pure KNN ceramic gradually turns into yellowish transparent ceramics. When 1 ≤ x ≤ 2, the font at the bottom and even distant objects can be clearly observed through the xSm ceramics, especially in 2Sm, exhibiting the “window” feature. By conducting the optical transmittance (T) tests [Figure 2B], it can be found that the T of xSm ceramics gradually improves and then deteriorates with the increase in Sm, which is in accordance with the naked eye observation. When 1 ≤ x ≤ 2, the optical transmittance is greater than 65% in the near-IR region
Figure 2. (A) Photographs and (B) optical transmittance spectra of the xSm transparent ceramics with a thickness of ~0.3 mm. (C) The optical transmittance spectra and photoluminescence excitation spectra monitored at 597 nm of the 1.0 Sm ceramic. (D) The photoluminescence emission spectra excitation at 407 nm of the xSm ceramics. (E) The schematic energy level diagram of Sm3+. (F) The plots of (hv)2 versus hv of the xSm transparent ceramics.
On the other hand, due to the absorption generated by the interband transition of electrons from the valence band to the conduction band, the optical transmittance of the xSm ceramics begins to drop to zero when it is less than ~400 nm [Figure 2B][26]. For KNN-based ceramics, the band gap (Eg) is defined as the transition from the top of the valence bands occupied by the O2p electron state to the bottom of the conduction bands dominated by the empty Nb4d electron state. A smaller Eg facilitates electronic transitions and reduces the energy used for light transport, leading to greater light losses and poorer optical transmittance. Therefore, the Eg plays a crucial role in transparency, which can be evaluated based on the Tauc equation according to the absorption band (at ~400 nm) for the optical transmittance spectra
Microstructure of the xSm transparent ceramics
In addition to the optical bandgap, the superior transparency of the xSm ceramics is also closely related to their microstructure. The SEM micrographs of the surface/cross-section for the xSm ceramics are shown in Figure 3A-C and Supplementary Figure 2. Appropriate increase in Sm content not only reduces the porosity of the xSm ceramics, but also significantly refines the grain size and improves the corresponding homogeneity. In addition, remarkable transgranular fracture characteristics can be reflected in the cross-section of the xSm ceramics with fine grains (x ≥ 1), indicating high compactness and excellent mechanical properties [Figure 3B1,C2 and Supplementary Figure 2A1,C1]. The relative density of the xSm ceramics with
Figure 3. (A-C) The free and (A1-C1) fracture surface of the xSm transparent ceramics. (D) EDS elemental analysis via the SEM micrographs of the 1.0 Sm transparent ceramic.
Figure 4A displays the XRD patterns for the xSm ceramics. Several main peak positions of the xSm ceramics are consistent with the PDF card (#77-0038) of pure KNN ceramics, indicating that the doped Sm3+ has been incorporated into the KNN matrix. Due to the A-site ions with large ionic radii (K+: 1.64 Å/CN = 12, Na+: 1.39 Å/CN = 12) are replaced by the Sm3+ ions with small ionic radii (1.24 Å/CN = 12), lattice shrinkage occurred. As a result, the diffraction peaks of xSm ceramics tend to shift to higher angles as x increases. Meanwhile, lattice shrinkage can also force the atomic spacing to shorten, inducing changes in bond angles/ lengths and suppressing ferroelectric distortion in the low-symmetry phase, leading to the phase transition. From the split (002)/(200) diffraction peaks near 2θ~45°, it can be observed that the (200) peak gradually disappears with the increase of x and eventually forms a unimodal peak, which indicates that the introduction of Sm gradually induces the formation of highly symmetric pseudo-cubic phase, labeled as the PC phase[31,34]. Due to the high optical symmetry of the pseudo-cubic phase, its increased content also helps to improve the optical transmittance. Further analysis of the Rietveld refinement results of XRD patterns shows that compared with pure KNN ceramics with a single orthorhombic phase, the proportion of pseudo-cubic phase in the 1.0 Sm sample is increased to 19.8%, and when x ≥ 2, it becomes a single pseudo-cubic phase [Figure 4B and Supplementary Figure 6]. In fact, when sintered under high-temperature conditions (e.g., > 1,100 °C), alkali metal ions (especially K) are prone to evaporate in KNN-based ceramics, so weak second phase (K6Nb10.8O30, PDF#32-0821) can be detected in the range of 2θ~24-30° when
Figure 4. (A) XRD patterns of the xSm ceramics. (B) Rietveld refinements for the 1.0 Sm ceramic. (C) Temperature-dependent dielectric constant and loss curves of the xSm ceramics. (D) PFM phase images of the xSm ceramics without and with +10 V DC tip biases applied.
Figure 4C displays the dielectric constant and dielectric loss as a function of the temperature of the xSm ceramics recorded at 10 kHz. Two peaks can be seen in all ceramics corresponding to the phase transitions from the orthorhombic to tetragonal phase (TO-T) and from the tetragonal to cubic phase (TC), respectively. When the content of Sm increases, the position of dielectric peaks in the xSm ceramics does not change obviously (especially TO-T), but the diffusion characteristics become more pronounced, indicating enhanced relaxor behavior and weakened ferroelectricity. Meanwhile, the doping of Sm also significantly reduces the dielectric loss, which originates from the improvement of the microstructure density
Ferro/piezoelectricity of the xSm transparent ceramics
P-E loops of the xSm ceramics were tested at room temperature and 10 Hz, as shown in Figure 5A. It is evident that all ceramics exhibit a typical P-E loop, and as x increases, the ferroelectric properties of the xSm ceramics first increase and then decrease. When x = 0.5, the increase in ferroelectricity (mainly for the Pmax) is due, on the one hand, to the increase in densification and, on the other hand, to the improvement of local heterogeneity caused by the formation of phase coexistence induced by trace Sm doping[38]. Both aspects improve the room temperature dielectric response, thereby enhancing the Pmax of 0.5 Sm. When
Figure 5. (A) P-E hysteresis loops and (B) I-E curves at 10 Hz of the xSm ceramics with a thickness of ~0.3 mm. (C) P-E hysteresis loops under different electric fields at 10 Hz of the 1.0 Sm ceramics with a thickness of ~0.25 mm. (D) d33 value and optical transmittance (at ~900 nm) of the xSm ceramics. (E) P-E hysteresis loops under different temperatures at 10 Hz of the 1.0 Sm ceramics with a thickness of ~0.3 mm. (F) The comprehensive performance comparisons of the 1.0 Sm ceramic with other representative KNN-based transparent ceramics.
Figure 5D displays the piezoelectric coefficient (d33) and optical transmittance at 900 nm of the xSm ceramics. The piezoelectric coefficient of the piezoelectric material is typically closely related to the residual polarization and dielectric constant[40], so the d33 of the xSm ceramics also increases first and then decreases, and reaches the optimal value in the 0.5 Sm ceramic (~112 pC/N). It is noteworthy that although the piezoelectric coefficient of the xSm ceramics decreases at x > 0.5, it remains at ~69 pC/N in the
Force sensitivity of the xSm transparent ceramics
The environmental protection, intelligence, and multi-functional integration of materials are the trends of science and technology development in the new era. For instance, observation windows in high-pressure environments such as high altitude (e.g., aerospace) or deep sea (e.g., diving) require the use of smart materials that are sensitive to external forces (e.g., piezoelectric transparent ceramics, TPC) to issue timely warnings in overpressure environments [Figure 6A], thereby avoiding damage to personnel and property caused by window rupture, which is not possible with traditional glass windows. Smart windows can be made by integrating TPC in the form of a sandwich inside a high-strength conventional transparent material to act as a transparent force-sensitive layer. For the xSm TPC with high Curie temperature, high optical transmittance, and good piezoelectricity, there is potential in this regard, so their pressure sensitive properties were further evaluated. Figure 6B displays the schematic diagram of the experimental apparatus for testing the output piezoelectric current signal; more detailed information can be found in the previous report[48]. The piezoelectric effect is a process of electromechanical conversion, and the piezoelectric output signal of the test can be divided into contact, compression, release, and separation of four stages[48,49]. It is generally assumed that the durations of contact and separation, as well as compression and release, are equal. In the contact stage, no current flows through the circuit, and this duration is represented as aT (T is the time of the entire cycle; a is related to the stimulus distance: the longer the stimulus distance, the larger a. Subsequently, as external force begins to compress the sample, the positive and negative charges separate and accumulate respectively on both sides of the sample. When there is an external circuit, an electric current is generated. After the duration of [(1-2a)/2]T, the external force reaches its maximum value, and the sample will undergo extreme deformation and produce peak current. Finally, external force begins to be released, generating a reverse peak current with a duration of [(1-2a)/2]T.
Figure 6. (A) The application concept of the piezoelectric transparent ceramics (TPC) in smart windows. (B) The diagram of pressure-electric response test. (C) Output voltage curves of the 1.0 Sm transparent ceramics under different pressures at 1 Hz. (D) The forward and reverse output voltage curves of the 1.0 Sm transparent ceramics under 1 Hz and 12.5 N. (E) The comparisons of the output voltage curves under 1 Hz and 2.5 N for the 1.0 and 1.5 Sm transparent ceramics.
Figure 6C shows the short-circuit voltage variation over time of the 1.0 Sm TPC with different uniaxial pressures and a frequency of 1 Hz. It can be observed that all short-circuit voltage curves exhibit nearly symmetrical positive and negative distributions over time, which is consistent with the response behavior of the opaque BCTHx ceramics and commercial PZT-based ceramics. This tendency is not influenced by the forward or reverse connection, as is the short-circuit current [Figure 6D, Supplementary Figure 8A]. This results from the force’s loading and unloading times being virtually identical. In addition, larger uniaxial pressures lead to greater deformation and thus more charge. Therefore, the short-circuit voltage of the
CONCLUSIONS
In summary, the transparent KNN-based ferro/piezoelectric ceramics (abbreviated as xSm) were developed based on simple single rare earth doping combined with traditional solid-state reaction method, which can be used for smart windows with superior force-sensitive properties by using piezoelectric effect. Through multidimensional microstructure analysis, it was found that proper Sm3+ doping induced finer grain size, higher densification and bandgap, and more pseudo-cubic phase, effectively reducing the incident light loss caused by the central scattering and optical anisotropy, thereby improving the optical transmittance. When 1 ≤ x ≤ 2, the optical transmittance is greater than ~65% at ~900 nm. As the Sm content further increased, the appearance of the impurity phase and the decrease in densification did not lead to a further increase in the optical transmittance. On the other hand, the doping of Sm also leads to the enhancement of relaxor behavior, resulting in the deterioration of piezoelectricity. Despite this, the 1.0 Sm ceramic still maintains good piezoelectricity. More importantly, due to the small degree of doping, the Curie temperature of KNN ceramics did not decrease significantly. High optical transmittance (~65%, 900 nm), high Curie temperature (~395 °C), and good piezoelectricity (~65 pC/N) make the 1.0 Sm ceramic competitive among KNN-based transparent ceramics. Interestingly, based on the force-sensitive testing, it was found that the 1.0 Sm ceramic produces excellent electrical signal output (~0.34 V) under low force action (10 N), and good cycling, which has great potential in the application of smart windows.
DECLARATIONS
Authors’ contributions
The conception and design of the work: Lin, J.; Li, P.; Yang, Z.
The acquisition and analysis of data: Lin, J.; Peng, J.; Huang, X.; Lin, Q.; Wu, X.
The interpretation of data: Lin, J.; Peng, J.; Lin, Q.
The writing and revising: Lin, J.; Peng, J.
The supervision: Li, P.; Yang, Z.
Availability of data and materials
The raw data are listed in the Supplementary Materials. Further data used to support the findings of this study are available from the corresponding authors upon reasonable request.
Financial support and sponsorship
This work was supported by the Fujian Province Young and Middle-aged Teacher Education Research Project (JZ240009) and the Innovation Team of Fujian Normal University (Y07204080K13).
Conflicts of interest
All authors declared that there are no conflicts of interest.
Ethical approval and consent to participate
Not applicable.
Consent for publication
Not applicable.
Copyright
© The Author(s) 2025.
Supplementary Materials
REFERENCES
1. Ke, Y.; Chen, J.; Lin, G.; et al. Smart windows: electro-, thermo-, mechano-, photochromics, and beyond. Adv. Energy. Mater. 2019, 9, 1902066.
2. Ke, Y.; Zhou, C.; Zhou, Y.; Wang, S.; Chan, S. H.; Long, Y. Emerging thermal-responsive materials and integrated techniques targeting the energy-efficient smart window application. Adv. Funct. Mater. 2018, 28, 1800113.
3. Chen, X.; Luo, L.; Zeng, Z.; et al. Bio-inspired flexible vibration visualization sensor based on piezo-electrochromic effect. J. Materiomics. 2020, 6, 643-50.
4. Kim, K.; Jeong, S.; Koo, B.; Ahn, H. Surface amending effect of N-doped carbon-embedded NiO films for multirole electrochromic energy-storage devices. Appl. Surf. Sci. 2021, 537, 147902.
5. Wu, X.; Lin, J.; Xu, Z.; et al. Defect management and multi-mode optoelectronic manipulations via photo-thermochromism in smart windows. Laser. Photonics. Rev. 2021, 15, 2100211.
6. Li, J.; Qu, W.; Daniels, J.; et al. Lead zirconate titanate ceramics with aligned crystallite grains. Science 2023, 380, 87-93.
7. Wang, L. V.; Yao, J. A practical guide to photoacoustic tomography in the life sciences. Nat. Methods. 2016, 13, 627-38.
8. Yan, P.; Qin, Y.; Xu, Z.; et al. H Highly transparent Eu-doped 0.72PMN-0.28PT ceramics with excellent piezoelectricity. ACS. Appl. Mater. Interfaces. 2021, 13, 54210-6.
9. Gao, X.; Qiao, L.; Qiu, C.; et al. A robust, low-voltage driven millirobot based on transparent ferroelectric crystals. Appl. Phys. Lett. 2022, 120, 032902.
10. Fang, Z.; Jiang, X.; Tian, X.; et al. Ultratransparent PMN-PT electro-optic ceramics and its application in optical communication. Adv. Opt. Mater. 2021, 9, 2002139.
11. Deng, C.; Ye, L.; He, C.; et al. Reporting excellent transverse piezoelectric and electro-optic effects in transparent rhombohedral PMN-PT single crystal by engineered domains. Adv. Mater. 2021, 33, e2103013.
12. Sun, E.; Cao, W. Relaxor-based ferroelectric single crystals: growth, domain engineering, characterization and applications. Prog. Mater. Sci. 2014, 65, 124-210.
13. Qiu, C.; Wang, B.; Zhang, N.; et al. Transparent ferroelectric crystals with ultrahigh piezoelectricity. Nature 2020, 577, 350-4.
14. Huangfu, G.; Zeng, K.; Wang, B.; et al. Giant electric field-induced strain in lead-free piezoceramics. Science 2022, 378, 1125-30.
15. Lin, J.; Wang, Y.; Xiong, R.; et al. Tailoring micro-structure of eco-friendly temperature-insensitive transparent ceramics achieving superior piezoelectricity. Acta. Mater. 2022, 235, 118061.
16. Ren, X.; Peng, Z.; Chen, B.; et al. A compromise between piezoelectricity and transparency in KNN-based ceramics: the dual functions of Li2O addition. J. Eur. Ceram. Soc. 2020, 40, 2331-7.
17. Ren, X.; Chai, Q.; Zhao, X.; et al. Relaxor behaviors and electric response in transparent 0. Ceram. Int. 2019, 45, 3961-8.
18. Kosec, M.; Bobnar, V.; Hrovat, M.; Bernard, J.; Malic, B.; Holc, J. New lead-free relaxors based on the K0.5Na0.5NbO3-SrTiO3 solid solution. J. Mater. Res. 2004, 19, 1849-54.
19. Du, H.; Zhou, W.; Zhu, D.; et al. Sintering characteristic, microstructure, and dielectric relaxor behavior of (K0.5Na0.5)NbO3-
20. Li, F.; Kwok, K.; Gupta, S. K0.5Na0.5NbO3-based lead-free transparent electro-optic ceramics prepared by pressureless sintering. J. Am. Ceram. Soc. 2013, 96, 3557-62.
21. Li, F.; Kwok, K. Fabrication of transparent electro-optic (K0.5Na0.5)1-xLixNb1-xBixO3 lead-free ceramics. J. Eur. Ceram. Soc. 2013, 33, 123-30.
22. Qu, B.; Du, H.; Yang, Z. Lead-free relaxor ferroelectric ceramics with high optical transparency and energy storage ability. J. Mater. Chem. C. 2016, 4, 1795-803.
23. Hutchinson, M.; Widom, M. VASP on a GPU: application to exact-exchange calculations of the stability of elemental boron. Comput. Phys. Commun. 2012, 183, 1422-6.
24. Hacene, M.; Anciaux-Sedrakian, A.; Rozanska, X.; Klahr, D.; Guignon, T.; Fleurat-Lessard, P. Accelerating VASP electronic structure calculations using graphic processing units. J. Comput. Chem. 2012, 33, 2581-9.
25. Liu, C.; Wang, Q.; Wu, X.; et al. Boosting upconversion photoluminescence and multielectrical properties via er-doping-modulated vacancy control in Ba0.85Ca0.15Ti0.9Zr0.1O3. ACS. Omega. 2019, 4, 11004-13.
26. Dai, Z.; Li, D.; Zhou, Z.; et al. A strategy for high performance of energy storage and transparency in KNN-based ferroelectric ceramics. Chem. Eng. J. 2022, 427, 131959.
27. Rahman, A.; Park, S.; Min, Y.; et al. An easy approach to obtain large piezoelectric constant in high-quality transparent ceramics by normal sintering process in modified potassium sodium niobate ceramics. J. Eur. Ceram. Soc. 2020, 40, 2989-95.
28. Sun, H.; Liu, J.; Wang, X.; Zhang, Q.; Hao, X.; An, S. (K,Na)NbO3 ferroelectrics: A new class of solid-state photochromic materials with reversible luminescence switching behavior. J. Mater. Chem. C. 2017, 5, 9080-7.
29. Zhang, Q.; Chen, K.; Wang, L.; Sun, H.; Wang, X.; Hao, X. A highly efficient, orange light-emitting (K0.5Na0.5)NbO3:Sm3+/Zr4+ lead-free piezoelectric material with superior water resistance behavior. J. Mater. Chem. C. 2015, 3, 5275-84.
30. Qiao, X.; Sheng, A.; Wu, D.; et al. A novel multifunctional ceramic with photoluminescence and outstanding energy storage properties. Chem. Eng. J. 2021, 408, 127368.
31. Yang, D.; Yang, Z.; Zhang, X.; Wei, L.; Chao, X.; Yang, Z. High transmittance in lead-free lanthanum modified potassium-sodium niobate ceramics. J. Alloy. Compd. 2017, 716, 21-9.
32. Chao, X.; Ren, X.; Zhang, X.; et al. Excellent optical transparency of potassium-sodium niobate-based lead-free relaxor ceramics induced by fine grains. J. Eur. Ceram. Soc. 2019, 39, 3684-92.
33. Zhao, X.; Chao, X.; Wu, D.; Liang, P.; Yang, Z. Simultaneous realization of high transparency and piezoelectricity in low symmetry KNN -based ceramics. J. Am. Ceram. Soc. 2019, 102, 3498-509.
34. Liu, H.; Wang, J.; Wang, H.; Xu, J.; Zhou, C.; Qiu, W. Er3+ and Sr(Bi0.5Nb0.5)O3-modified (K0.5Na0.5)NbO3: a new transparent fluorescent ferroelectric ceramic with high light transmittance and good luminescence performance. Ceram. Int. 2022, 48, 4230-7.
35. Cheng, L.; Wang, K.; Yao, F.; Zhu, F.; Li, J.; Zhang, S. Composition inhomogeneity due to alkaline volatilization in Li-modified
36. Yang, W.; Li, P.; Wu, S.; Li, F.; Shen, B.; Zhai, J. A study on the relationship between grain size and electrical properties in
37. Ren, X.; Jin, L.; Peng, Z.; et al. Regulation of energy density and efficiency in transparent ceramics by grain refinement. Chem. Eng. J. 2020, 390, 124566.
38. Pinho, R.; Tkach, A.; Zlotnik, S.; et al. Spark plasma texturing: a strategy to enhance the electro-mechanical properties of lead-free potassium sodium niobate ceramics. Appl. Mater. Today. 2020, 19, 100566.
39. Yang, Z.; Du, H.; Qu, S.; et al. Significantly enhanced recoverable energy storage density in potassium-sodium niobate-based lead free ceramics. J. Mater. Chem. A. 2016, 4, 13778-85.
40. Li, P.; Fu, Z.; Wang, F.; et al. High piezoelectricity and stable output in BaHfO3 and (Bi0.5Na0.5)ZrO3 modified
41. Zhang, X.; Yang, D.; Yang, Z.; et al. Transparency of K0.5N0.5NbO3-Sr(Mg1/3Nb2/3)O3 lead-free ceramics modulated by relaxor behavior and grain size. Ceram. Int. 2016, 42, 17963-71.
42. Yang, D.; Ma, C.; Yang, Z.; et al. Optical and electrical properties of pressureless sintered transparent (K0.37Na0.63)NbO3-based ceramics. Ceram. Int. 2016, 42, 4648-57.
43. Kwok, K. W.; Li, F.; Lin, D. A novel lead-free transparent ceramic with high electro-optic coefficient. Funct. Mater. Lett. 2011, 4, 237-40.
44. Wu, X.; Lu, S.; Kwok, K. Photoluminescence, electro-optic response and piezoelectric properties in pressureless-sintered Er-doped KNN-based transparent ceramics. J. Alloy. Compd. 2017, 695, 3573-8.
45. Zhao, X.; Chai, Q.; Chen, B.; Chao, X.; Yang, Z. Improved transmittance and ferroelectric properties realized in KNN ceramics via SAN modification. J. Am. Ceram. Soc. 2018, 101, 5127-37.
46. Liu, Y.; Yi, W.; Yan, C.; Ma, J.; Fan, L. A novel transparent material based on KNN ferroelectric ceramics. Ferroelectrics 2021, 573, 173-8.
47. Chai, Q.; Zhao, X.; Chao, X.; Yang, Z. Enhanced transmittance and piezoelectricity of transparent K0.5Na0.5NbO3 ceramics with Ca(Zn1/3Nb2/3)O3 additives. RSC. Adv. 2017, 7, 28428-37.
48. Huang, X.; Dong, G.; Zhang, Y.; et al. Decoding the intrinsic frequency response behaviors of piezoelectric output current toward advanced sensing and monitoring applications. Nano. Energy. 2025, 134, 110544.
Cite This Article
How to Cite
Download Citation
Export Citation File:
Type of Import
Tips on Downloading Citation
Citation Manager File Format
Type of Import
Direct Import: When the Direct Import option is selected (the default state), a dialogue box will give you the option to Save or Open the downloaded citation data. Choosing Open will either launch your citation manager or give you a choice of applications with which to use the metadata. The Save option saves the file locally for later use.
Indirect Import: When the Indirect Import option is selected, the metadata is displayed and may be copied and pasted as needed.
About This Article
Special Issue
Copyright
Data & Comments
Data
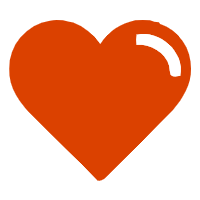
Comments
Comments must be written in English. Spam, offensive content, impersonation, and private information will not be permitted. If any comment is reported and identified as inappropriate content by OAE staff, the comment will be removed without notice. If you have any queries or need any help, please contact us at [email protected].