Cathodic dehalogenation polymerization of 2′,3′,5′,6′-tetrafluoro-4,4″-diiodo-1,1′:4′,1″-terphenyl on zinc metal surface for anode protection
Abstract
Fluorine-containing poly(p-phenylene) (CityU-42) films on zinc surfaces were directly synthesized using a cathodic dehalogenation C-C coupling strategy. The as-prepared polymers can effectively protect the zinc substrate in aqueous zinc-ion batteries. Because CityU-42 is rich in the electronegative fluorine group, it can attract the uniform deposition and rapid diffusion of Zn2+ on the surface of the anode. Moreover, a large number of benzene rings provide certain mechanical strength, enabling the protective layer to inhibit the growth of dendrites. As a result, the symmetric Zn Zn cell used CityU-42@Zn can stably cycle for over 1,900 h under
Keywords
INTRODUCTION
Conjugated polymers have attracted significant attention in materials science and engineering in recent years, owing to their distinctive electronic and optical properties[1,2]. These polymers exhibit considerable potential in various applications, including transistors, energy storage devices, thermoelectric materials, sensors and catalysts, primarily due to their tunable physical and chemical characteristics[3-10]. Notably, the construction of C-C bonds between aryl repeating units is crucial in the synthesis of polymers, as this process not only influences the structure and properties of polymers, but also directly influences their performance in various applications. To achieve C-C bond construction, researchers have developed various synthetic methodologies, including the Ullmann reaction, Grignard reaction, Suzuki reaction, and the Stille and Negishi coupling[11-14]. Although these methods have made some progress in synthesizing conjugated polymers, they often necessitate a stringent anhydrous and anaerobic environment and the use of heavy metal catalysts[15]. Such requirements not only complicate the synthesis process and increase costs but also pose potential environmental risks. Furthermore, conjugated polymers produced via these conventional methods frequently exhibit poor solubility, which limits their ability to form uniform and dense films, thereby compromising their performance in practical applications[16]. Therefore, the development of novel methods for clean C-C bond construction under mild conditions is of particular importance.
Electrochemical techniques are recognized as a promising method for polymer synthesis, owing to their precise and controllable nature. Many organic reactions involve the transfer and coordination of electrons, making electrochemical polymerization - where electrons act as catalysts - an efficient and environmentally sustainable method[17-19]. Furthermore, electrochemical deposition (ECD) and electrophoretic deposition (EPD) are regarded as versatile and reliable methods for thin film preparation[20-22]. Thus, employing electrochemical techniques for the growth of polymer films represents an ideal solution to effectively address some limitations of conventional methods while facilitating the formation of C-C bond-coupled polymers. Despite the significant potential of electrochemical methods in organic polymer synthesis, traditional coupling through electrochemical oxidation on anodic surfaces encounters challenges when preparing thin films on metal electrodes[23,24]. Conversely, utilizing the negative potential of the cathodic metal electrodes can effectively protect the electrode surface, enabling the successful preparation of conjugated polymer films on various metal substrates. Our research group’s prior works have demonstrated that this approach offers the advantage of achieving C-C coupling via cathodic dehalogenation reactions, eliminating the need for heavy metal catalysts and resulting in the construction of stable polymer structures[18,19]. This advancement opens a new pathway for the synthesis of conjugated polymers with substantial application potential.
Aqueous zinc-ion batteries (AZIBs) have garnered significant attention for their high safety, affordability, and environmental friendliness, positioning them as a highly promising option for energy storage devices[25-27]. However, the performance of zinc metal anodes is hindered by challenges such as poor cycling stability, zinc dendrite formation, hydrogen evolution reactions (HER), corrosion, and surface passivation[28]. Addressing these issues is critical for the commercial viability of AZIBs, necessitating the design and modification of zinc metal anodes. To overcome these obstacles, various strategies have been developed, among which interface modification stands out as one of the most straightforward and effective approaches[29-31]. This method involves applying a protective or functional layer on the surface of the zinc anode to suppress dendrite growth and mitigate hydrogen evolution, ensuring more uniform Zn2+ deposition and enhancing the anode's reversibility[32]. Organic polymers, favored for their excellent flexibility, high mechanical strength, and chemical stability, are widely employed in the interface modification of zinc anodes[33]. Moreover, in-situ chemically formed protective layers offer superior mechanical stability and adhesion compared to ex-situ coatings[34-41]. As a result, the direct fabrication of polymer films on zinc metal surfaces through electrochemical methods presents a highly advantageous solution.
Herein, we synthesized fluorine-containing poly(p-phenylene) (named CityU-42) using a cathodic dehalogenation C-C coupling strategy with 2′,3′,5′,6′-tetrafluoro-4,4''-diiodo-1,1′:4′,1″-terphenyl (TFDITP). Since the cathodic dehalogenation method is effective in protecting metal substrates, we directly synthesized this polymer film on a zinc metal surface with the aim of using it for electrode protection in AZIBs [Scheme 1]. The film is composed of a large number of benzene rings and F atoms altering with each other. The introduction of rigid aromatic rings in the polymer chain improves the overall rigidity and stability of the polymer network, which can enhance the surface strength of the electrode sheet after coating. This can inhibit the growth of sharp dendrites and prevent the rupture of the protective layer[42,43]. In addition, F atoms exhibit electronegativity, which can attract Zn2+ to deposit uniformly and diffuse rapidly on the anode. Owing to these significant functions of the CityU-42 film, the Zn Zn symmetric cell achieves a long cycle life of 1,900 h at 5 mA cm-2 and 1 mAh cm-2. Furthermore, the CityU-42@Zn NVO full cell exhibits high-capacity retention at 5 A g-1. The experimental results show that CityU-42 on the zinc anode surface can effectively inhibit the growth of zinc dendrites, which significantly extends the service life of the battery. This result provides a new material choice and strategy for improving the performance of AZIBs.
MATERIALS AND METHODS
Synthesis of CityU-42@Zn
The dehalogenated C-C coupling synthesis of CityU-42 films was carried out in a three-electrode electrochemical cell (zinc foil as working electrode, platinum wire as counter electrode, Ag/Ag+ as reference electrode) containing dimethylsulfoxide (DMSO) as the electrolyte solution and 0.05 M tetrabutylammonium hexafluorophosphonate (TBAPF6) as the supporting electrolyte. TFDITP was first synthesized prior to this according to the synthetic route shown in Supplementary Figure 1. The electrochemical synthesis was conducted by multicycle cyclic voltammetry (CV) in the potential range from -1.8 to -3 V (scanning rate: 50 mV/s) on CH Instruments 760E [Supplementary Figure 2]. A uniform and dense CityU-42 film was deposited on the surface of zinc metal foil (working electrode) (CityU-42@Zn).
RESULTS AND DISCUSSION
Successful coupling of CityU-42 was demonstrated by Fourier-transform infrared spectroscopy (FTIR) and Raman spectroscopy. As shown in Figure 1A, the characteristic peak located at 814 cm-1 is the “out-of-plane” C-H stretching of para-disubstituted phenylenes in CityU-42. A characteristic peak of hybridized C-H vibrations on the aromatic ring also appears at 2,924 cm-1[16]. The original vibration peak of the C-I bond located at 498 cm-1 disappeared compared with that of the TFDITP monomer, proving the breakage of the C-I bond[18]. Meanwhile, the C-F vibration peak at 971 cm-1 still existed[44,45]. The Raman spectra further proved the successful preparation of CityU-42 [Figure 1B]. The three peaks located at 1,194, 1,320, and 1,612 cm-1 are characteristic peaks of poly(p-phenylene)[16,18]. In addition, the peaks located at 406, and
Figure 1. (A) FTIR spectra of the precursor TFDITP and the as-prepared CityU-42 film. (B) Raman spectra of CityU-42. (C) XPS survey of CityU-42. High-resolution XPS spectra of (D) C 1s and (E) F 1s for CityU-42. (F) SEM image of CityU-42@Zn.
A scanning electron microscopy (SEM) image showed that CityU-42 formed a smooth and dense film on the Zn surface [Figure 1F]. The thickness of the CityU-42 film deposited on the Zn surface was about
The superiority of CityU-42@Zn was further elucidated through comprehensive electrochemical characterization. As depicted in Figure 2A, the symmetric cell based on CityU-42@Zn exhibits a smaller and more stable polarization voltage compared to bare Zn, maintaining stable cycling for nearly 2,000 h. In contrast, the bare Zn cell has a significantly shorter lifespan of only 311 h due to dendrite-induced short circuits (Figure 2A insert). The rate performance of Zn Zn symmetric cells, measured at progressively increasing current densities from 0.5 to 10 mA cm-2 while maintaining a fixed area capacity of
Figure 2. (A) Cycling performance and (B) Rate performance of bare Zn and CityU-42@Zn symmetric batteries. SEM plots of (C) Bare Zn and (D) CityU-42@Zn after cycling.
To further evaluate the electrochemical performance, a series of tests were conducted. The linear scanning voltammetry (LSV) curves, spanning a voltage range from -0.5 to 3.0 V, demonstrated changes in decomposition potential and current response [Figure 3A]. The oxygen evolution reaction (OER) and HER at the CityU-42@Zn electrode were significantly suppressed compared to bare Zn, confirming that CityU-42 films improve the electrochemical stability of the electrolyte at the anode surface [Figure 3B and C]. Notably, the response current on the CityU-42@Zn working electrode was consistently lower than that on the bare Zn electrode across the entire voltage range. The electrochemical performance of Zn deposition and stripping was evaluated using Zn Ti half cells. The voltage profiles during the Zn nucleation stage are shown in Figure 3D. The CityU-42@Ti electrodes exhibit a significantly lower nucleation overpotential compared to bare Ti. This reduction is primarily attributed to the enhanced interfacial smoothness, which facilitates zinc ion deposition and mitigates energy fluctuations. Furthermore, the CityU-42 film, characterized by its strong zincophilicity and linear structure, enhances ion diffusion and improves ionic conductivity. This improvement ensures a more uniform delivery of zinc ions to the electrode surface, promoting stable zinc deposition and minimizing the risk of localized oversaturation at specific sites. Consequently, the nucleation energy is reduced, and uniform deposition is achieved. Additionally, electrochemical impedance spectroscopy (EIS) measurements [Supplementary Figure 8] reveal that the CityU-42@Zn symmetric cell exhibits significantly lower charge-transfer resistance than bare Zn, suggesting improved Zn2+ transfer kinetics. Supplementary Figure 9 illustrates the enhanced rate capability of the CityU-42@Zn cell. At a low current density of 0.5 A g-1, it delivers an average capacity similar to that of initial cycles. However, at higher current densities of 1, 2, 3, and 5 A g-1, the CityU-42@Zn cell exhibits superior capacities compared to the bare Zn. Upon returning to the 0.5 A g-1 current density, the cell maintains a capacity of 240 mAh g-1, consistent with the first several cycles. In contrast, the bare Zn cell demonstrates a lower capacity of 230 mAh g-1 under the same conditions. Finally, full cells were assembled to assess the practicality of using commercial V2O5 as the cathode for the CityU-42@Zn anode. The Zn
Figure 3. (A) Electrochemical stability window of Zn Zn symmetric cells assembled with bare Zn and CityU-42@Zn. The corresponding zoomed-in curves: (B) Low potential area and (C) High potential area. (D) Voltage profiles during initial Zn plating on the Ti foil and CityU-42@Ti at 1 mA cm-2. (E) Long-term cycling stability tests of Zn V2O5 full cells assembled with bare Zn and CityU-42@Zn.
CONCLUSIONS
Employing the cathodic dehalogenation strategy, we directly prepared a polymer film (CityU-42) on the surface of zinc metal for electrode protection in AZIBs. As a result, the symmetric Zn Zn cell used CityU-42@Zn can cycle stably for 1,900 h under the condition of 5 mA cm-2 and 1 mAh cm-2. Impressively, the CityU-42@Zn V2O5 full cell also exhibits a reversible capacity and high capacity retention. These results confirm that CityU-42@Zn can effectively inhibit the growth of Zn dendrites, significantly extending the service life of the battery. This achievement provides new material selection and strategies for improving the performance of AZIBs and other energy storage devices.
DECLARATIONS
Acknowledgments
Zhang, Q. acknowledges financial support from the City University of Hong Kong, Hong Kong Branch of the National Precious Metals Material Engineering Research Center (NPMM), Hong Kong, China. Zhang, Q. also thanks the Innovation and Technology Fund, the National Natural Science Foundation of China, and the Shenzhen Science and Technology Program.
Authors’ contributions
The conception and design of the work: Wu, J.; Naren, T.; Chen, L.; Zhang, Q.
Materials synthesis: Wu, J.; Zhang, S.
Data acquisition: analysis and interpretation: Wu, J.; Naren, T.; Zhang, S.; Jiang, R.; Wang, X.; Gu, Q.;
Availability of data and materials
The data supporting the findings of this study are available within this Article and its
Financial support and sponsorship
This work was supported by the City University of Hong Kong (9380117 and 7020089), Hong Kong Branch of the National Precious Metals Material Engineering Research Center (NPMM), Hong Kong, China; the Innovation and Technology Fund (ITF, ITS/322/22), the National Natural Science Foundation of China (NSFC, 22475183), and the Shenzhen Science and Technology Program (JCYJ20240813153135046).
Conflicts of interest
All authors declared that there are no conflicts of interest.
Ethical approval and consent to participate
Not applicable.
Consent for publication
Not applicable.
Copyright
© The Author(s) 2025.
Supplementary Materials
REFERENCES
1. Zhuo, Z.; Ni, M.; Yu, N.; et al. Intrinsically stretchable fully π-conjugated polymer film via fluid conjugated molecular external-plasticizing for flexible light-emitting diodes. Nat. Commun. 2024, 15, 7990.
2. Fu, M.; Chen, Y.; Jin, W.; et al. A donor-acceptor (D-A) conjugated polymer for fast storage of anions. Angew. Chem. Int. Ed. 2024, 63, e202317393.
3. Yin, J.; Li, J.; Chen, H.; et al. Programmable zigzag π-extension toward graphene-like molecules by the stacking of naphthalene blocks. Nat. Synth. 2023, 2, 838-47.
4. Kong, J.; Song, S.; Yoo, M.; et al. Long-term stable polymer solar cells with significantly reduced burn-in loss. Nat. Commun. 2014, 5, 5688.
5. Xiao, M.; Ren, X.; Ji, K.; et al. Achieving ideal transistor characteristics in conjugated polymer semiconductors. Sci. Adv. 2023, 9, eadg8659.
6. Wu, X.; Wang, S.; Huang, W.; Dong, Y.; Wang, Z.; Huang, W. Wearable in-sensor reservoir computing using optoelectronic polymers with through-space charge-transport characteristics for multi-task learning. Nat. Commun. 2023, 14, 468.
7. Wu, J.; Liu, J.; Rao, L.; et al. A covalent organic polymer containing dative B ← N bonds: synthesis, single crystal structure, and physical properties. Inorg. Chem. Front. 2024, 11, 8285-9.
8. Shi, Y.; Li, J.; Sun, H.; et al. Thiazole imide-based all-acceptor homopolymer with branched ethylene glycol side chains for organic thermoelectrics. Angew. Chem. Int. Ed. 2022, 61, e202214192.
9. Wu, J.; Zhang, S.; Gu, Q.; Zhang, Q. Recent progress in covalent organic frameworks for flexible electronic devices. FlexMat 2024, 1, 160-72.
10. Cheng, L.; Ma, T.; Zhang, B.; et al. Steering the topological defects in amorphous laser-induced graphene for direct nitrate-to-ammonia electroreduction. ACS. Catal. 2022, 12, 11639-50.
11. Milstein, D.; Stille, J. K. A general, selective, and facile method for ketone synthesis from acid chlorides and organotin compounds catalyzed by palladium. J. Am. Chem. Soc. 1978, 100, 3636-8.
12. Miyaura, N.; Yamada, K.; Suzuki, A. A new stereospecific cross-coupling by the palladium-catalyzed reaction of 1-alkenylboranes with 1-alkenyl or 1-alkynyl halides. Tetrahedron. Lett. 1979, 20, 3437-40.
13. King, A. O.; Okukado, N.; Negishi, E. Highly general stereo-, regio-, and chemo-selective synthesis of terminal and internal conjugated enynes by the Pd-catalysed reaction of alkynylzinc reagents with alkenyl halides. J. Chem. Soc. Chem. Commun. 1977, 19, 683-4.
14. Ullmann, F.; Bielecki, J. Ueber synthesen in der biphenylreihe. Ber. Dtsch. Chem. Ges. 1901, 34, 2174-85.
15. Pouliot, J. R.; Grenier, F.; Blaskovits, J. T.; Beaupré, S.; Leclerc, M. Direct (Hetero)arylation polymerization: simplicity for conjugated polymer synthesis. Chem. Rev. 2016, 116, 14225-74.
16. Abdulkarim, A.; Hinkel, F.; Jänsch, D.; Freudenberg, J.; Golling, F. E.; Müllen, K. A new solution to an old problem: synthesis of unsubstituted poly(para-phenylene). J. Am. Chem. Soc. 2016, 138, 16208-11.
18. Wang, X.; Wu, J.; Liu, H.; Kang, F.; Yan, F.; Zhang, Q. Cathodic polymerization through electrochemical dehalogenation. Macromolecules 2023, 56, 10198-205.
19. Wang, X.; Zhang, L.; Wu, J.; et al. Constructing N-containing poly(p-Phenylene) (PPP) films through a cathodic-dehalogenation polymerization method. Small. Methods. 2024, 8, e2400185.
20. Zeng, C.; Zheng, W.; Xu, H.; et al. Electrochemical deposition of a single-crystalline nanorod polycyclic aromatic hydrocarbon film with efficient charge and exciton transport. Angew. Chem. Int. Ed. 2022, 61, e202115389.
21. Zeng, C.; Wang, B.; Zhang, H.; et al. Electrochemical synthesis, deposition, and doping of polycyclic aromatic hydrocarbon films. J. Am. Chem. Soc. 2021, 143, 2682-7.
22. Besra, L.; Liu, M. A review on fundamentals and applications of electrophoretic deposition (EPD). Prog. Mater. Sci. 2007, 52, 1-61.
23. Shi, G.; Jin, S.; Xue, G.; Li, C. A conducting polymer film stronger than aluminum. Science 1995, 267, 994-6.
24. Ambade, R. B.; Ambade, S. B.; Shrestha, N. K.; et al. Controlled growth of polythiophene nanofibers in TiO2 nanotube arrays for supercapacitor applications. J. Mater. Chem. A. 2017, 5, 172-80.
25. Li, G.; Zhao, Z.; Zhang, S.; et al. A biocompatible electrolyte enables highly reversible Zn anode for zinc ion battery. Nat. Commun. 2023, 14, 6526.
26. Han, M.; Chen, D.; Lu, Q.; Fang, G. Aqueous rechargeable Zn-iodine batteries: issues, strategies and perspectives. Small 2024, 20, e2310293.
27. Sui, B. B.; Sha, L.; Wang, P. F.; et al. Salt solution etching to construct micro-gullies on the surface of Zn anodes enhances anodes performance in aqueous zinc-ion batteries. J. Colloid. Interface. Sci. 2024, 653, 159-69.
28. Zhao, L.; Zhao, S.; Zhang, N.; et al. Construction of stable Zn metal anode by inorganic functional protective layer toward long-life aqueous Zn-ion battery. Energy. Storage. Mater. 2024, 71, 103628.
29. Gao, J.; Xie, Y.; Zeng, P.; Zhang, L. Strategies for optimizing the Zn anode/electrolyte interfaces toward stable Zn-based batteries. Small. Methods. 2023, 7, e2300855.
30. Chen, P.; Yuan, X.; Xia, Y.; et al. An artificial polyacrylonitrile coating layer confining zinc dendrite growth for highly reversible aqueous zinc-based batteries. Adv. Sci. 2021, 8, e2100309.
31. Zhang, Z.; Xi, B.; Ma, X.; Chen, W.; Feng, J.; Xiong, S. Recent progress, mechanisms, and perspectives for crystal and interface chemistry applying to the Zn metal anodes in aqueous zinc-ion batteries. SusMat 2022, 2, 114-41.
32. Liu, Z.; Li, G.; Xi, M.; et al. Interfacial engineering of Zn metal via a localized conjugated layer for highly reversible aqueous zinc ion battery. Angew. Chem. Int. Ed. 2024, 63, e202319091.
33. Wang, Y.; Li, G.; Wang, X.; et al. Recent advances of organic polymers for zinc-ion batteries. Sustain. Energy. Fuels. 2022, 6, 5439-58.
34. Li, J.; He, B.; Zhang, Y.; et al. In situ constructing coordination compounds interphase to stabilize Zn metal anode for high-performance aqueous Zn-SeS2 batteries. Small 2022, 18, e2200567.
35. Ye, P.; Li, X.; He, K.; et al. A semi-interpenetrating network polymer coating for dendrite-free Zn anodes. J. Power. Sources. 2023, 558, 232622.
36. Zheng, J.; Liu, X.; Zheng, Y.; et al. AgxZny protective coatings with selective Zn2+/H+ binding enable reversible Zn anodes. Nano. Lett. 2023, 23, 6156-63.
37. Ma, G.; Chen, K.; Qiao, H.; Liu, J.; Dong, H.; Gao, Y. Porous V2CTx MXene as a High stability zinc anode protective coating. Nano. Lett. 2024, 24, 14552-8.
38. Li, G.; Wang, X.; Lv, S.; et al. In situ constructing a film-coated 3D porous Zn anode by iodine etching strategy toward horizontally arranged dendrite-free Zn deposition. Adv. Funct. Mater. 2023, 33, 2208288.
39. Xia, S.; Luo, Q.; Liu, J.; et al. In situ spontaneous construction of zinc phosphate coating layer toward highly reversible zinc metal anodes. Small 2024, 20, e2310497.
40. Fu, H.; Wen, Q.; Li, P.; et al. In-situ chemical conversion film for stabilizing zinc metal anodes. J. Energy. Chem. 2022, 73, 387-93.
41. Liu, H.; Li, Z.; Sui, B.; et al. Calcium alginate hydrogel coating comprehensively optimizes Zn deposition behavior of aqueous zinc-ion batteries anode. Ind. Eng. Chem. Res. 2024, 63, 13611-22.
42. Li, Q.; Yan, B.; Wang, D.; et al. Mechanistic study of interfacial modification for stable Zn anode based on a thin separator. Small 2022, 18, e2201045.
43. Naren, T.; Kuang, G. C.; Jiang, R.; et al. Reactive polymer as artificial solid electrolyte interface for stable lithium metal batteries. Angew. Chem. Int. Ed. 2023, 62, e202305287.
44. Zhu, Q.; Han, C. C. Synthesis and crystallization behaviors of highly fluorinated aromatic polyesters. Polymer 2007, 48, 3624-31.
45. Krishnakumar, V.; Mathammal, R. A joint FTIR, FT-Raman and scaled quantum mechanical study of 1,3-dibromo-2,4,5,6-tetra-fluoro benzene (DTB) and 1,2,3,4,5-pentafluoro benzene (PB). J. Raman. Spectrosc. 2009, 40, 1104-9.
46. Zang, L.; Ren, Y.; He, M.; Chen, B.; Hu, B. Fluorine-functionalized covalent-organic-framework-coated stir bar for the extraction of benzoylurea insecticides in pear juice and beverage followed by high-performance liquid chromatography-ultraviolet detection. J. Agric. Food. Chem. 2022, 70, 12689-99.
47. Huang, P.; Xiong, T.; Zhou, S.; et al. Advanced Tri-layer carbon matrices with π-π stacking interaction for binder-free lithium-ion storage. ACS. Appl. Mater. Interfaces. 2021, 13, 16516-27.
48. Li, W.; Wang, J.; Jia, C.; Chen, J.; Wen, Z.; Huang, A. Covalent organic framework-derived fluorine, nitrogen dual-doped carbon as metal-free bifunctional oxygen electrocatalysts. J. Colloid. Interface. Sci. 2023, 650, 275-83.
Cite This Article
How to Cite
Download Citation
Export Citation File:
Type of Import
Tips on Downloading Citation
Citation Manager File Format
Type of Import
Direct Import: When the Direct Import option is selected (the default state), a dialogue box will give you the option to Save or Open the downloaded citation data. Choosing Open will either launch your citation manager or give you a choice of applications with which to use the metadata. The Save option saves the file locally for later use.
Indirect Import: When the Indirect Import option is selected, the metadata is displayed and may be copied and pasted as needed.
About This Article
Special Issue
Copyright
Data & Comments
Data
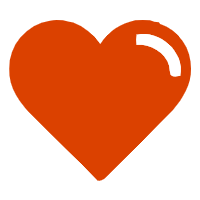
Comments
Comments must be written in English. Spam, offensive content, impersonation, and private information will not be permitted. If any comment is reported and identified as inappropriate content by OAE staff, the comment will be removed without notice. If you have any queries or need any help, please contact us at [email protected].