Advances in functional applications of biomass-derived carbon composites for phase change materials
Abstract
The existence of biomass materials as the only renewable carbon source is an extremely important resource in the realm of modern energy and materials science. Biomass-derived carbon composites (BDCCs), with their unique advantages and applications, such as wide sources, low cost, high carbon content and tunable structure, have promoted progress and innovation in several technological fields and played an indispensable part in mitigating environmental pollution and promoting sustainable development in energy storage and conversion applications. Phase change materials (PCMs), which possess latent heat storage and release properties, have been widely applied in the field of energy storage and utilization. Nonetheless, several obstacles limit the application of PCMs, including the occurrence of leakage during operation and the relatively low thermal conductivity. The porous structure of BDCCs enhances the thermal conductivity of PCMs and effectively prevents leakage. Consequently, there has been growing interest among researchers in BDCCs/PCMs. Nevertheless, there is a paucity of literature providing a comprehensive and detailed introduction to BDCCs and their applications in PCMs. On this basis, this review will provide an overview of the feedstock classes and synthesis methods based on recent research advances, followed by an investigation of the potential functional applications of BDCCs. Ultimately, this paper provides a targeted summary of the development advantages and challenging opportunities of BDCCs in functional applications based on the current state of research and emerging needs, providing constructive references for the future development and efficient utilization of BDCCs/PCMs.
Keywords
INTRODUCTION
The accelerated pace of scientific and technological advancement has given rise to a multitude of energy and environmental concerns. Sustainable social development is threatened by issues that vary from energy consumption to environmental pollution[1-4]. Fossil energy, including coal, oil and natural gas, is a non-renewable resource whose reserves are diminishing annually. On the other hand, the utilization and exhaustion of fossil fuels result in critical environmental problems, such as increased greenhouse gas emissions, global warming, atmospheric pollution and so forth[5]. Therefore, it is necessary and urgent to exploit clean energy and environmentally friendly materials that improve the situation of energy depletion and environmental damage. To address these challenges, the global energy structure is evolving towards decarbonization, while the development and utilization of renewable energy has become a strategic choice for many countries in the world, including solar energy, wind energy, water energy, biomass energy, geothermal energy and ocean energy[6-10]. Hence, there is no denying that the utilization of clean energy and eco-friendly materials assumes a crucial role in enhancing the efficiency of energy storage and conversion, guaranteeing system security, promoting technological innovation, and fulfilling the demand for versatility. Meanwhile, the storage and conversion of energy is vitally essential to ensure the stability and sustainability of the energy supply, such as electrochemical energy storage and electromagnetic interference (EMI) shielding[11-18]. In this regard, the identification of appropriate energy storage materials is of paramount importance, with phase change materials (PCMs) emerging as a particularly promising avenue due to their distinctive energy storage characteristics.
The defects of PCMs can be effectively overcome by confining them to carbon-based materials with high thermal conductivity. This includes enhancing their low thermal conductivity to facilitate accelerated heat transfer rate, reducing phase separation during melting, mitigating the risk of leakage, augmenting cycle stability, optimizing shape stability, and diversifying PCMs functions such as building insulation and microwave absorption[19-24]. Despite the superior electrical conductivity, thermal stability and mechanical strength of traditional carbon-based materials such as graphene, carbon nanotubes (CNTs), etc., which are suitable for PCMs, they are non-renewable resources, and their high manufacturing cost and process energy consumption limit their large-scale applications and sustainable development[25-27]. Due to the requirements of functional applications on the structure, performance and potential of materials, biomass has become a key and promising resource and material for achieving sustainability and carbon neutrality because of its high carbon content, renewability, environmental-friendliness and wide application potential[28,29]. As a renewable resource, biomass-derived carbon composites (BDCCs) are conducive to reducing the impact on the environment due to their high natural abundance, low cost, environmental-friendliness, naturally existing hierarchical structure and simple processability[30-34]. They have been extensively applied in lithium-ion batteries (LIBs)[35-37], supercapacitors[38,39], fuel cells[40], solar cell[41], etc. Furthermore, their porous structure can effectively boost the thermal conductivity and energy storage efficiency of PCMs. Consequently, BDCCs have attracted academic interest and have been widely used in the industrial field. Currently, research on PCMs has concentrated on functional applications such as thermal management, solar thermal energy conversion and storage, and electromagnetic shielding[42-46]. Owing to the existence of pore size distribution, high specific surface area (SSA), etc., BDCCs can offer numerous adsorption sites for PCMs, and they possess remarkable advantages as functional supporting materials for phase change energy storage materials[47-49]. It can be definitely concluded that the porous structure of BDCCs has an impact on the energy storage system of PCMs, resulting in differences in performance of functional applications. Thus, the synthesis of BDCCs featuring appropriate pore structures is an extremely crucial task to facilitate the optimization of multifunctional applications.
At present, BDCCs have become a significant research direction in the field of PCMs due to their unrivaled morphological structure and other characteristics. It is noteworthy that BDCCs fulfill multiple roles within the PCM field, including the provision of supporting carriers to reinforce mechanical properties, as a phase change substrate for the storage and release of heat energy, and as a thermal conductivity material to improve heat transfer efficiency[44,50,51]. Indeed, although many review articles have focused on the utilization of PCMs in conjunction with carbon-based materials, they frequently do not adequately cover the progress in the preparation of BDCCs and their vast potential for diverse applications in PCMs. This bias may have caused us to miss out on exploring the unique benefits and innovative opportunities of BDCCs in improving PCM performance and expanding their range of applications, such as building energy efficiency[23], solar thermal utilization systems[44], and battery thermal management[48]. These materials absorb and release a lot of heat through a phase change procedure, which can effectively regulate the temperature and improve the energy efficiency of the system. Porous BDCCs usually have high SSA and thermal conductivity, which can increase the contact area with PCMs and enhance heat exchange efficiency. They can also serve as a stable carrier of PCMs to reduce the volume change of PCMs during the phase change process, so as to prevent the leakage of the phase change matrix and maintain the cycle stability of composites. Furthermore, adjusting the pore structure and chemical properties of porous carbon (PC) composites can also be combined with other functional materials, such as polymers[52], conductive materials[53,54], magnetic materials[51], etc., to give PCMs more additional functions, to achieve more complex energy management.
The pursuit of sustainable energy practices has become a critical priority and the sustainable management of energy has become increasingly important as we address the challenges of rising energy demand and pressing environmental issues. This review concentrates on advances in BDCCs from preparation to functional applications, especially their performance and potential in PCMs. Through an in-depth examination of the structure and characteristics of these materials, we anticipate enhancements in their applicability across energy storage, thermal management, and electromagnetic wave absorption domains, thereby fostering the advancement of new energy technologies. Consequently, at the beginning of this paper, the source, classification and various preparation methods of BDCCs are introduced and analyzed. The microstructure of a material is pivotal in determining its macroscopic properties, which are intrinsically linked to its effectiveness and adaptability in practical multifunctional applications. For instance, bagasse biomass-derived carbon (BBC) serves as a supporting material to address issues such as agglomeration, leakage and low thermal conductivity of hydrated salts[55], and the honeycomb structure of cork powder (CP) can better encapsulate the phase change matrix inside the battery[56]. Subsequently, we meticulously review recent progress made in BDCC research concerning these four applications about PCMs: solar energy utilization, building materials, thermal management, and electromagnetic shielding. Notably, BDCCs have demonstrated considerable potential and advantages across PCMs. Ultimately, drawing upon current research findings, we provide insights into future opportunities and challenges pertaining to the functionalization of BDCCs in PCMs, hoping to provide a valuable reference point for future investigations into biochar in PCMs.
FUNDAMENTALS OF BDCCs
Carbon, a ubiquitous element in the natural world, is integral to the composition of various biological and non-biological entities. Carbon materials manifest themselves in a diverse array of forms, such as diamond, graphite, fullerenes, CNTs, graphene, activated carbon (AC), biochar and carbon composites. However, not all carbon materials are readily available and low-cost. BDCCs are an ideal material in the field of sustainable development and environmental technologies due to their renewability, environmental friendliness, cost-effectiveness, structural diversity, chemical functionality, and high efficiency in energy storage and environmental remediation.
Source of BDCCs
BDCCs originate from a multitude of sources, as illustrated in Figure 1, which depicts the principal categories of biomass precursors. Following the implementation of suitable processing and synthesis techniques, these biomass resources can be transformed into high-performance PC composites characterized by a high SSA, a porous structure and a rich surface chemistry.
Plant
In the long course of evolution, plants have formed unique channel structures and pore structures, which are the key for plants to adapt to the environment and maintain life activities. The main components of plants are generally cellulose, hemicellulose and lignin with natural structure, which are arranged and interact in a specific way in the plant cell wall to form a complex and sophisticated network. It can possibly exist in the form of lotus[61], hemp stems[62], teak[58], sunflower[63], hazelnut shell[64], rose[65], opuntia humifusa[66], rice husk[67], water hyacinth[68], bagasse[69], and corn stalk[70]. After carbonization of these plant-derived biomass, these natural structures are able to retain some of their original morphological characteristics and even have a hierarchical structure, providing the possibility of customization for the microstructure and macroscopic properties of the material[71,72]. Yang et al. directly co-pyrolyzed agroforestry biomass with potassium oxalate, customized the pore structure by adjusting the pyrolysis temperature and nitrogen doping, and prepared two-dimensional (2D) carbon composites with high SSA, developed pore structure and controllable carbon layer thickness[70]. In addition, the special interlacing tubular structures and extra-hierarchical porous structures of Ganoderma lucidum-derived porous CNTs contributed to an extensive SSA of 1731.51 m² g-1 and a significant pore volume of 0.76 cm³ g-1[73]. With diversified conversion pathways and sophisticated preparation techniques, these plants can be shaped into PC composites with a wide range of pore sizes and shapes.
Animals
Animal waste generally refers to the waste generated during the life cycle of animals and after their death, including carcasses, excrement and industrial processing by-products. Typically, animal biomass encompasses a variety of waste, such as manure, feathers, horns, hides, blood, bone, and cells[74]. For animal-based biomass, the organic components such as protein and carbohydrates can be converted into carbon in an anaerobic or aerobic environment by pyrolysis of bone or excreta. For instance, the complex internal structure of animal bones creates natural porosity at the microscopic level due to the specific arrangement of minerals and organic matter in the bones. After undergoing a series of specialized treatments, such as defatting, demineralization, and carbonization, the organic and inorganic components are removed, leaving behind a PC skeleton with a high SSA and tunable physicochemical properties[75,76]. Tarimo et al. transformed cooked chicken bone waste into high-performance PC composites through chemical activation[77]. Additionally, carbon precursors can also be combined with biomass from plant and animal sources. Yang et al. prepared BDCCs with hierarchical porous structure, high SSA and large pore volume by using sugarcane bagasse and beef bone powder through hydrothermal carbonization (HTC) and KOH activation[78]. The temperature, time and atmosphere of this process will affect the degree of graphitization and pore characteristics of carbon materials. The above BDCCs derived from living organisms are generally biocompatible and biodegradable.
Microorganisms
Microorganisms are omnipresent in various environments, and their diversity and functionality play an irreplaceable role in ecosystems. A multitude of microorganisms can be used to prepare BDCCs, including but not limited to bacteria, fungi, and yeasts, which are converted into biochar through pyrolysis and other methods, and then combined with functional materials to prepare carbon composites with specific properties. Among them, yeast, as a cost-effective microorganism, benefits from its widespread use in numerous industrial applications. It is not only readily available but also inexpensive, and these properties make it the preferred material for obtaining carbon sources. Besides, Yuan et al. have prepared biomass-derived PC composite with microbial fermentation assistance, which can improve the activation efficiency and lower the carbonization temperature[79].
Human waste
Biomass serves as a vital resource in our daily existence, extensively utilized and converted into a diverse array of industrial commodities. However, the production process will bring different forms of waste, which, if not properly handled, will cause serious environmental damage and pollution. In order to reduce environmental pollution and improve the recycling rate of resources, it is necessary to effectively transform waste into valuable resources. Egun et al. converted waste tires into carbon materials with high SSA, optimized pore structure and excellent electrochemical activity through thermochemical treatment methods such as pyrolysis, carbonization and activation[80]. Furthermore, agricultural wastes such as garlic stem[81], pecan nutshells[82] and solanum melongena[83] also can be used to prepare BDCCs with certain pore structures. Through continuous technological innovation, we can make full use of biomass resources, transform human waste and industrial by-products into high-value-added BDCCs.
Classification of BDCCs
BDCCs can be classified as zero-dimensional (0D), one-dimensional (1D), 2D, and three-dimensional (3D) materials according to different dimensions. Varying from 0D to 3D, each dimension of the material exhibits different properties due to its unique internal structure and external shape, which is suitable for specific fields. During the preparation of BDCCs, the physical and chemical properties of materials can be improved by adjusting the activation method and optimizing the preparation process[84,85]. This multi-dimensional structural optimization strategy covers BDCCs ranging from zero to three dimensions, aiming to fully exploit the unique advantages of each dimension of carbon materials by precisely regulating the morphology, pore structure, and surface properties of these materials. Figure 2 illustrates the typical structure of carbon materials in different dimensions.
Zero-dimensional carbon materials generally exhibit spherical or similarly granular morphologies with similar nanostructures in all directions and a high SSA. These materials mainly include carbon spheres[88], carbon dots[86], fullerenes[89], and graphene quantum dots[90], which feature regular small geometric shapes and tunable pore structures. Meanwhile, biomass, including agricultural and forestry waste, is a rich renewable precursor for these materials. Its utilization helps reduce environmental pollution and promotes resource recycling. For 1D carbon materials, they exhibit a high length-to-diameter ratio, namely the high aspect ratio. Typically, they have a linear, tubular, or fibrous structure, formed by carbon atoms arranged in different ways. These materials can be obtained through methods such as chemical vapor deposition, pyrolysis and template-assisted carbonization[73,91]. One-dimensional materials are typically categorized into three types based on their diameter: CNTs, carbon nanofibers, and carbon fibers. Then, based on the size of the pore, pores are mainly classified into micropores (< 2 nm), mesopores (2-50 nm) and macropores (> 50 nm). Additionally, 2D carbon materials are single or multiple layers of carbon atoms on the plane in a specific arrangement of 2D structure of the material. Firstly, the sp2 hybrid carbon atoms in 2D carbon materials form a stable and unique planar structure through covalent bonds, which confer excellent electrochemical properties and mechanical strength. They can accelerate the propagation of electrons through the plane. Secondly, the high SSA of the 2D layered porous structure provides rich active sites for electrochemical reactions, which can shorten the ion transport distance in the dimension. The structure has been demonstrated to enhance the thermal conductivity and overall performance of the PCMs by constructing continuous thermal conductivity channels, thereby facilitating enhanced interfacial heat conduction, improved thermal diffusion efficiency, and augmented mechanical properties. Two-dimensional carbon materials such as carbon nanosheets, graphene and graphyne have unique microscopic structures of layered, flake-like, and ribbon-like, and are widely used in the field of electrochemistry due to their high conductivity and large SSA[92-94]. In contrast, 3D carbon materials composed of multilayers of other dimensional materials, such as layered PC, carbon aerogel and flexible 3D carbon film, etc., have a wider application range. In contrast to carbon materials of other dimensions, the internal network structure of 3D carbon materials can provide a more stable support framework and larger SSA, offering abundant electrical contacts that are conducive to the transfer of electrons and ions. Additionally, with thermal and chemical stability, 3D carbon materials are capable of maintaining reliable performance under harsh conditions. They hold a broad and promising application prospect in the domains of energy storage, sensors, adsorption, and separation. The BDCCs for the different dimensions are shown in Figure 3.
PREPARATION OF BDCCs
BDCCs are substances derived from living organisms or organic polymers formed through photosynthesis. They are mainly composed of chemical elements such as carbon, hydrogen, and oxygen. Biomass is the only renewable source of carbon and an important precursor of carbon materials[101]. As shown in Figure 2, BDCCs can be obtained mainly through processes such as pyrolysis, HTC, activation and template-assisted carbonization[102-104]. According to the feedstock type, choosing an appropriate measure to prepare BDCCs with high yield and required microstructure is crucial, rendering them applicable in a multitude of fields.
Pyrolysis
Proper heat treatment of biomass waste can improve resource utilization, enable waste utilization, and promote the use of renewable energy sources, such as agroforestry residues and industrial waste[60,104-107]. Pyrolysis is a direct carbonization approach wherein biomass is heated and decomposed within a high-temperature inert atmosphere to acquire carbon materials. Nevertheless, the pore structure of the obtained carbon materials will influence properties such as SSA and electrical conductivity, thereby leading to utilization deficiencies. Thus, it is especially important that the properties of raw materials and reaction conditions will affect the final yield and physical and chemical properties of the product to some extent[108-110]. Due to the differences in residence time, peak temperature and heating rate, the pyrolysis process can be further divided into slow, intermediate and fast pyrolysis. Slow pyrolysis, often referred to as carbonization, occurs over a relatively low-temperature range with low heating rate (< 10 °C min-1), long residence times and high biocarbon production, while fast pyrolysis is the opposite, with intermediate pyrolysis in between[111,112]. Fast pyrolysis is typically kept at a heating rate of 10-200 °C s-1 with a short residence time, and is well-suited for the production of bio-oil with a yield of about 70 wt%[113-115].
Activation
The procedure of carbonization and pyrolysis results in a significant reduction in the size of biochar, which in turn leads to a decline in porosity and a reduction in the SSA. Consequently, the thermal conductivity and photothermal conversion efficiency of PCMs undergo a change in different degrees. For this reason, activation was applied to obtain high-performance BDCCs, and activated carbonization can be mainly divided into physical and chemical activation. The resulting material exhibits a high SSA and porosity, rendering it a suitable candidate for utilization in a multitude of applications, such as thermal management and EMI[42,116-118].
Physical activation
Physical activation can be precisely classified into two distinct processes: carbonization and activation. The carbonization stage is usually pyrolysis in an inert atmosphere with a temperature of less than 800 °C, and then the activation stage is conducted in an activated gas atmosphere in the temperature range of 600-1200 °C, such as CO2, O2, air and steam, so it is also known as gas-assisted pyrolysis. High SSA and uniformly distributed porous structure are obtained under the effect of these activated gases[84,114,119,120]. However, compared with air activation, steam and CO2 activation occur at higher temperatures, corresponding to greater SSA of carbon materials. For example, Pallarés et al. used barley straw as raw material for physical activation, and the residence time under CO2 atmosphere at 800 °C was one hour. The surface area and micropore volume of the prepared AC were 789 m2 g-1 and 0.3268 cm3 g-1, respectively, which increased by 43% and 42% compared with steam atmosphere[121]. Combined with machine learning technology, the analysis shows that the activators and activation temperature have an effect on the pore structure of PC, and the morphology and porosity of the composites can be regulated under appropriate conditions to make it used in thermal energy storage[122,123]. However, the activation process has the disadvantages of high energy consumption in the preparation process, long activation time required for AC, and low adsorption capacity.
Chemical activation
To acquire carbon materials featuring larger surface area and higher yield, the mixture of activators and carbon precursor is usually subjected to pyrolysis through chemical activation in an inert gas atmosphere within the temperature range of 400-900 °C. The gases produced during the chemical activation process contribute to the formation of a large number of micropores and mesoporous pores, and these pore structures are key factors in increasing the SSA. After the excess activator was removed by acid or water treatment, the porous BDCCs could be formed[118,124]. Generally, activators in the treatment process include acids (HCl, HNO3, H2SO4 and H3PO4)[125-128], alkalis (KOH, NaOH)[129,130], salts (K2CO3, ZnCl2)[131,132], and oxidants (KMnO4)[133,134]. Meanwhile, the pore textures and carbonization degree of activated products have a significant influence on the properties of BDCCs, which is closely related to the types of activators used in the activation procedure. Among diverse activators, KOH is the most prevalently utilized in the preparation of PC for functional applications[123,129,135-137]. Biochar contains plenty of O-containing functional groups, which provide active sites for adsorption and reaction. Since KOH is easy to react with O-containing active groups in biomass, it is simple to activate biomass raw materials to generate more and homogeneously distributed micropores and obtain carbon materials with higher yields[138]. However, KOH is corrosive and toxic, posing significant environmental harm, and potassium salt can be considered as a substitute[139]. In contrast to physical activation, chemical activation is a procedure that involves one-step direct activation or two-step activation and carbonization for the fabrication of AC. It features a low heating temperature, a short activation time, excellent porosity control, and a larger pore volume of the resultant carbon material. However, the waste water and activator residues in the process of acid or water washing will cause serious danger to the environment.
HTC
Unlike traditional carbonization processes, HTC is a process in which biomass raw materials are converted to carbon materials in hydrothermal conditions of high temperature and internal pressure (< 10 MPa), usually in the temperature range of 180-250 °C without the use of harmful surfactants or catalysts, and the carbonization product is hydrochar[140,141]. HTC is a kind of environmentally friendly method, and the biomass raw materials with high moisture content can be carbonized without drying, eliminating this energy consumption step. The main working principles in the HTC process are shown in Figure 4[142]. What is more, the product yield is 70%-80% higher than that of pyrolysis and chemical activation[102]. Additionally, the morphology and structure of carbonization products are related to hydrothermal conditions including temperature, relation time and rate, which indicate that functional groups exert influence on physicochemical performance. Hydrochar is a partially carbonized product with high density of oxygen-containing functional groups and low polycondensation, and has a highly stable aromatic structure[143]. Besides, the surface functional groups can be removed by heat treatment. PC can be prepared from hydrochar by chemical activation, and the porosity is related to the elemental composition of hydrochar[144,145].
Figure 4. The main process of HTC[142]. HTC: hydrothermal carbonization.
Template-assisted carbonization
Utilizing template-assisted carbonization techniques, it is feasible to fabricate PC with precisely tailored nanostructures and an optimal pore size distribution. This methodology offers an effective approach for the synthesis of advanced materials with controlled porosity characteristics. The pore size of the obtained PC ranges from 1 to 200 nm, and the pore structure and distribution are highly well-ordered or disordered, which cannot be achieved by activation. Meanwhile, template-assisted carbonization mainly includes three kinds of templates: hard template, soft template and self-template[146].
The structural characteristics of PC largely determined the properties of the templates. Normally, the biomass carbon precursor was put into the prepared hard template, dehydrated, polymerized and carbonized under high-temperature conditions in an inert atmosphere, and finally the PC was obtained by the removal of the template with acid or base[147,148]. Hard templates such as SiO2[149], MgO[150,151],
Besides the above methods, the self-template method does not need to use additional templates, but uses the characteristics of the substance itself to form the required porous structure, mainly including biomass and MOFs. It can leverage biomass (such as cellulose, lignin, peel, eggshell, etc.) as a precursor, through the pyrolysis and activation process to prepare PC with high SSA and customizable structure[146,158]. Because it is difficult to control the pore size and pore distribution of biomass self-template, the pore volume and SSA of PC prepared by the self-template method are lower than those of soft templates. Overall, the hard-template method has a wide range of practicability and application.
APPLICATIONS FOR PCMs
Biomass, as a renewable resource with high cost-effectiveness, possesses a highly-stable naturally porous or hierarchical structure that plays an important role in the functional applications of BDCCs. Such structures are difficult to achieve through traditional chemical synthesis and artificial design. Its unique structure gives the material rich adsorption sites and complex coupled conductive network, but also significantly improves the key performance parameters such as thermal conductivity, shape stability and photothermal conversion efficiency of BDCCs in PCM applications. Through their inherent phase change process, PCMs can change the state of matter and provide latent heat at constant temperature, which is environmentally friendly and biodegradable. On this basis, the following sections of this review will critically analyze and systematically compare the progress of BDCCs in functional applications for PCMs such as solar energy utilization, building materials, thermal management and EMI.
In the context of the current global energy crisis, geopolitical conflicts, global climate change and economic fluctuations have compounded the tension between energy supply and demand and led to sharp fluctuations in energy prices, which together pose a serious threat to global economic and environmental security[159-161]. PCMs are special substances that can change the state of matter and provide latent heat while maintaining a constant temperature. Hence, they show great potential in improving solar energy utilization efficiency due to their distinctive thermal storage characteristics, which offer a novel approach for alleviating the energy crisis and fostering sustainable development. With their excellent heat absorption and release properties, PCMs have demonstrated significant potential for application across various fields such as waste heat recovery[162], building energy efficiency[163], solar energy utilization[44], thermal management of electronic devices[116], healthcare[42], seawater desalination[164], and automotive industry[165-167]. Due to the inherent limitations of single PCMs in practical applications, including low thermal conductivity, insufficient energy conversion efficiency and potential leakage issues in some cases, researchers have been integrating PCMs with various functional fillers or support materials to develop composites with multiple energy conversion capabilities, as shown in Figure 5[168]. These composites not only enhance the thermal performance of PCMs but also endow them with functionalities such as photo/electric/magnetic-thermal conversion. Consequently, the prevailing research trend in PCMs is increasingly oriented toward the development of multifunctional composites rather than individual PCMs for comprehensive applications.
Figure 5. Preparation and applications of photo/electric/magnetic-thermal conversion functional PCMs[168]. PCM: Phase change material.
Solar energy utilization
Photothermal conversion is a common application feature of PCMs; it absorbs solar energy during the day and converts it into heat energy storage, efficiently stores and releases heat energy at night or when needed, effectively utilizes abundant solar energy resources, and achieves energy efficiency and balanced energy supply and demand through store and release heat energy, playing an important role in solar energy utilization. Thereinto, functional carrier materials are crucial to the performance of photothermal conversion phase change composites (PCCs), such as various carbon-based materials and metal nanoparticles, because of their unique optical and thermal properties, can effectively absorb sunlight, thereby improving the light absorption capacity of composites.
Currently, PCMs are presenting new opportunities for the effective utilization of solar energy. The advancement of high-performance PCMs characterized by superior heat transfer capabilities and enhanced latent thermal stability during shape phase change via BDCCs holds significant importance for thermal energy storage. Wang et al. incorporated organic PCMs and palmitic acid into biochar aerogel by a simple vacuum impregnation method. The resulting composite maintained almost constant phase change enthalpy after 50 melting/freezing cycles, up to 207.9 to 271 kJ kg-1, with excellent thermal stability and recyclability, and thermal conductivity is correspondingly enhanced[169]. Additionally, the one-step strategy constructs PCCs composed of sodium alginate and MXene-wrapped polyethylene glycol (PEG), which form a gel network through Ca2+ crosslinking, thereby endowing the material with shape stability. Simultaneously, the composite significantly increases in temperature to 75.4 °C under illumination for 780 s, and is capable of storing solar energy as latent heat during the illumination period. As shown in Figure 6A and B, The enthalpy reduction of shape-stable biomass/MXene PCCs with 4% MXene (SMPCC-4) was negligible even after 100 cooling-heating cycles and 20 light-thermal cycles[170]. Huang et al. developed a series of biomass-derived shape-stable organic PCCs with high latent heat capacity by using paraffin wax (PW), introducing poly(styrene-b-ethylene-co-butylene-b-styrene) (SEBS) and expanded perlite (EP) as dual supporting components, as well as hexagonal boron nitride (BN) and carbonized lignin (CL) to enhance thermal conductivity and photothermal conversion capability [Figure 6C]. Due to the high thermal conductivity of BN itself and its ability to be uniformly dispersed within the composite to form an effective thermal conduction pathway, the thermal conductivity of multi-component PCCs also increases from 0.407 to 0.596 W·m-¹·K-¹ with the increasing loading of BN[171]. Based on this, Huang et al. also exploited a high enthalpy efficiency and flame retardant porous composite aerogel PCM (ZrP-PI@PEG) for efficient solar-thermal conversion and storage. In-situ biochar produced by sodium lignosulfonate-zirconium phosphate was used to enhance the solar-thermal conversion and flame-retardant properties, and the adsorption ratio reached 95.5%. ZrP-PI@PEG exhibits excellent shape stability at 80 ℃ and remarkable thermal stability at around 300 ℃[172]. Aiming to further promote the high-value utilization of lignin, Shen et al. showed that the thermal reliability after 300 heating and cooling cycles and the high solar-thermal conversion capacity after 30 cycles were excellent when three PCMs were encapsulated in a lignin-based chemically crosslinked sponge used as a supporting material[173]. Cellulose, a type of plant fiber, serves as a carbon precursor used in the preparation of 3D directional cellulose-based carbon aerogels (CBCA). These aerogels are then melt-blended with stearic acid (SA) and graphene, followed by a vacuum-impregnating process to form 3D PCCs. The thermal storage capacity of the composite is greater than 96%; the highest thermal conductivity is 1.17 W·m-¹·K-¹, which exceeds 266% of CBCA, as illustrated in Figure 6D. The photothermal conversion efficiency is up to 90.3% and the maximum light-thermal-electric energy conversion output power can achieve 1.80 mW, providing a feasible path for efficient solar energy storage and thermal energy utilization[50]. Liu et al. utilized biomass-derived SiC ceramics for rapid thermal energy storage. Figure 6E demonstrates that the continuous SiC skeleton, consisting of tightly stacked grains, loaded with PW resulted in a PCC porosity of 85%. It indicated a high thermal conductivity of 10.34 W·m-¹·K-¹ and did not exhibit significant decay in thermal conductivity and energy storage density after 500 charging-discharging cycles[174]. Particularly, Hu et al. constructed a PC framework derived from Pleurotus eryngii with an ultra-low volume shrinkage rate of 2% using a NaCl-assisted carbonization. They observed the impact of pore size on thermal performance, and hence innovatively conducted simulation studies on heat transfer and storage mechanisms using COMSOL Multiphysics software, thereby confirming that adjusting the NaCl concentration to regulate pore size is an effective strategy for the controllable modulation of the thermal energy storage capacity in PCCs. As shown in Figure 6F, the temperature distribution and phase change distribution of PEG in PCCs with different pore size models vary during the heating procedure[175]. In another work, Hou et al. successfully prepared a series of bamboo-derived PC as a supporting carrier and photosensitizer for 1-octadecanol (OC) using Ca(OH)2 as a precursor by combining high-temperature carbonization with template-assisted and activation methods. When the carbonization temperature is
Figure 6. (A) Phase change enthalpy of SMPCC-4 before and after 100 cool-heating cycles; and (B) before and after 20 light-thermal cycles[170]; (C) Schematic graph of the fabrication process of EP-BN, EP-BN@CL, and n-Docosane-based biomass-derived shape-stable organic PCCs[171]; (D) Light-thermal conversion curves of all samples[50]; (E) Cross-sectional and longitudinal-sectional magnified SEM images of S-SiC porous ceramics[174]; (F) Sample thermal performance characterization and COMSOL Multiphysics software simulation graphs[175]; (G) SEM of the sample containing 70 wt% OC[176]; (H) Schematic diagram of the enhancement of thermal conductivity anisotropy with the addition of GF and the heat conduction of the PCCs[177]. GF: gravity force; PCC: phase change composite.
The main challenges faced by solar energy utilization applications include low energy conversion efficiency and thermal conductivity of storage systems, poor structural stability, and limited light absorption capacity. These issues restrict the effective capture and long-term stable use of solar energy, leading to a mismatch between energy supply and demand, and potentially increasing the complexity and cost of systems. Additionally, traditional PCMs may encounter leakage risks, slow thermal response rates, and inadequate photothermal conversion performance in practical applications. Therefore, the research and development of new and highly efficient photothermal conversion PCCs are crucial for solar energy utilization. Photothermal conversion PCCs represent an innovative class of materials designed for solar energy harvesting, heat storage, and energy transformation. By integrating BDCCs, such as PC, CNTs, carbon aerogel and graphene, these materials enable efficient absorption and conversion of solar energy. Due to their high SSA and porous structure, these BDCCs significantly enhance the photothermal conversion efficiency and thermal energy storage density, not only providing excellent light absorption properties, but also improving the thermal conductivity and structural stability of the composites.
Building materials
As the global population grows and living standards improve, the demand for comfortable indoor environments increases, resulting in rising building energy consumption. The construction sector is one of the major sources of greenhouse gas emissions, which exacerbates the global climate change problem, thus driving the demand for low-carbon and environmentally friendly building materials. PCMs can help regulate room temperature by storing and releasing large amounts of latent heat during phase change. This means that heat is absorbed during the day and released at night, thereby reducing reliance on mechanical heating and cooling systems and reducing the heat load on buildings, thereby reducing energy consumption[179,180].
In order to develop a lightweight building material with excellent thermal insulation and energy-saving properties and sufficient mechanical strength, Qi et al. used PEG as PCMs to fill polyvinyl chloride (PVC) tubes, resulting in phase-change hollow wood composites (PHWC). The phase change temperature of PEG is between 23.4 °C and 29.6 °C, which matches the comfortable temperature range inside buildings, helping to absorb heat during the day and release it at night, thereby improving indoor thermal comfort. PHWC demonstrates excellent thermal insulation performance, with a thermal conductivity ranging from 0.06 to 0.07 W·m-¹·K-¹ at 33 °C, making it suitable for building insulation. Additionally, samples with added PVC tubes present higher values of Modulus of Rupture and Elasticity, indicating that their mechanical properties are enhanced[181]. In the research where polymers are similarly utilized for the preparation of PCCs, Xu et al. incorporated high-density polyethylene (HDPE) into the composite. They used AC with a dendritic pore structure as a carrier and prepared AC/PEG composites with a high loading capacity of up to 70% and uniform distribution through a vacuum adsorption method, as shown in Figure 7A. Subsequently, the AC/PEG was mixed with wood powder and HDPE, and the wood-plastic composite with phase change heat storage function was made by hot pressing process. The composite not only demonstrated excellent heat storage properties, but also boosted mechanical properties, with a 167% increase in elongation at break and an increase in material rigidity[182]. In Figure 7B, three different porous bamboo-derived materials were used as a PW skeleton to construct PCCs with a hierarchical structure. The structure provides enough space to load PW and prevents leakage through intermolecular force such as capillary force, which promotes shape stability and heat energy storage efficiency. The highest thermal conductivity of bamboo-derived PCMs is about 2.17 times higher than that of pure PW, reaching 0.522 W·m-¹·K-¹[183]. Besides, due to their higher latent heat, stable chemical properties and low supercooling, lauric acid (LA) and SA are commonly used in the preparation of PCMs for the construction sector to achieve passive temperature regulation of buildings and reduce energy consumption of air conditioning and heating systems. Optimizing the weight ratio of LA and SA can make PCCs reach the lowest eutectic temperature of 31.2 °C, which is close to the typical indoor comfortable temperature range, and can effectively absorb and release heat in the building envelope, which has practical value in construction applications[163]. Hydrated salts show great application prospects in the field of building heat storage because of their high heat storage capacity, economical value and non-flammability. However, hydrated salts also have some drawbacks, mainly including large supercooling phenomenon, shape maintenance difficulties, and the tendency to phase separation. Therefore, Wang et al. prepared bagasse-derived carbon with a highly oriented and stacked lamellar structure by using biological waste as a resource, which can mitigate or eliminate its own defects as a support carrier for hydrated salts and simplify its storage process. As confirmed in Figure 7C, compared with a pure sodium sulfate decahydrate (SSD), the addition of BBC improves the thermal conductivity of PCCs, up to 1.79 W·m-¹·K-¹. The application and experimental diagram can be seen in Figure 7D[55]. Zheng et al. successfully introduced nano-Ag particles into the PC/PEG PCCs through a high-voltage electrostatic field; the mechanism was illustrated in Figure 7E, a process that enhanced photothermal conversion efficiency. After being soaked in the simulated concrete pore solution for seven days, the photothermal conversion efficiency reached 110.6%, demonstrating a thermal storage density of 147 J g-1, and showing resistance to common aggressive ions such as Cl- and SO42-[23].
Figure 7. (A) Fabrication mechanism of AC/PEG and A-WPCs[182]; (B) The synthesis of bamboo-derived PCCs[183]; (C) Thermal conductivities of pure SSD and other PCCs; (D) Application and experimental diagram for the solar-thermal energy conversion[55]; (E) Schematic diagram of photothermal conversion mechanism of nano-Ag particles[23]. AC: activated carbon; PEG: polyethylene glycol; SSD: sodium sulfate decahydrate; PCC: phase change composite.
Integrating PCMs into the building sector is expected to significantly enhance energy efficiency by absorbing and releasing heat when the building experiences temperature fluctuations. The use of this material in buildings can effectively store thermal energy during the heating and cooling cycle, subsequently releasing this energy when needed, thereby reducing dependence on traditional energy sources and lowering energy consumption. Although PCMs show great potential for improving building energy efficiency and reducing environmental impact, several challenges remain in their practical application. The low thermal conductivity of PCMs restricts their heat transfer efficiency and may adversely affect their mechanical properties when integrated with construction materials such as concrete. Furthermore, PCMs necessitate advanced packaging technologies to mitigate leakage and volatilization, thereby increasing the complexity and cost associated with these materials. Combining it with BDCCs can gradually improve these problems, thereby enhancing the long-term stability and durability of PCMs, and has broad application prospects in heat storage, heat regulation and energy saving.
Thermal management
With the rapid development of electronic technology, the increasing power consumption of electronic devices has made heat dissipation a critical issue. In the field of electric vehicles, LIBs are widely used due to their high energy density and long cycle life. However, thermal runaway in LIBs, which involves rapid temperature rise due to irreversible chemical reactions, can lead to dangerous situations such as battery leakage, fire, or even explosion[184,185]. This highlights the importance of effective thermal management. PCCs can regulate temperature by absorbing and releasing latent heat, preventing overheating and enhancing thermal stability. Functional BDCCs for LIBs are prepared through mechanisms such as porous structure, heteroatom doping, surface modification, composite construction, and activation treatment, as shown in Figure 8. These mechanisms improve electrochemical properties, ensuring the safety and reliability of LIBs and demonstrating broad application prospects.
Figure 8. Fabrication mechanism for BDCCs/LIBs. BDCC: biomass-derived carbon composite; LIB: lithium-ion batterie.
Batteries produce heat in the operation process, especially in the fast charging and discharging or high-power output. Efficient thermal energy storage materials can help battery thermal management systems regulate temperature and prevent overheating, thereby extending battery life and improving safety. Wu et al. discovered that the diatom frustules calcined at 400 °C (400CDF) were a suitable PEG supporting skeleton, and the PEG/400CDF composite prepared has a PEG adsorption capacity of 72.7%, a relative enthalpy efficiency of 97.7%, and still shows thermal and chemical stability after 200 thermal cycles, as shown in Figure 9A. It is potentially valuable for the design and development of high-performance shape-stable PCMs for thermal management applications[186]. The relationship between materials and thermal management applications is very strong, especially in terms of energy efficiency and sustainability, helping to maintain the battery temperature within a safe and efficient range. Muthya Goud et al. used myristol alcohol, whose melting point range (33-37 °C) was suitable for the ideal operating range (15-40 °C) of LIBs, as PCMs, and then physically mixed with biochar to form composites of different proportions. Figure 9B presents that when the biochar is not less than 24 wt%, PCCs show shape stability, indicating that they can maintain their shape during phase transition and are not prone to leakage[187]. Doping nanomaterials with excellent thermal conductivity in PCMs is a common and effective method to improve their thermal conductivity. However, nanomaterials are not only more costly, but also may significantly reduce the latent heat capacity of PCMs. Therefore, Xiong et al. extracted biochar microparticles from food waste garlic stems, which possess a 3D porous structure and 2D flaky morphology in Figure 9C. These characteristics contribute to the formation of additional heat transfer pathways within PW, thereby enhancing thermal conductivity while maintaining a low reduction in latent heat capacity[188]. In another work, the bio-based Mxene-mixed aerogel/PW PCCs constructed by Huang et al. have excellent response rate; the photo/electrothermal conversion efficiency can reach 97.45% and 98.89%, respectively, and the load rate is 95.16%. Moreover, the relative enthalpy efficiency of the sample after different cycles remained above 98%, as can be seen in Figure 9D. The PCCs with a high enthalpy value (231.38 J g-1) make them ideal for thermal management applications such as industrial waste heat recovery and thermal management of electronic equipment[189]. As described in Figure 9E, Lee et al. successfully combined the excellent properties of bio-based thermoplastic polymers with the heat storage capacity of PCMs, to prepare a heat-conducting composite with high thermal conductivity through injection molding technology using a mixed packing system of oxidized carbon fiber (CF-OH) and aluminum nitride. The material achieves a high through-plane thermal conductivity of 4.25 W·m-¹·K-¹ and a latent heat of 170.7 J g-1, a 189% improvement over pure erythritol. This result allows the material to conduct heat efficiently in addition to having a high latent heat, which combines energy saving and heat dissipation characteristics to promote effective thermal management[190]. Luo et al. used CP, a natural biodegradable biomass material, as the supporting skeleton of n-docosane (ND), and CP with different mesh numbers has different microstructures. CP80 and CP160 can better encapsulate ND inside the battery due to their complete cellular structure and high encapsulation rate; CP160 has excellent air tightness, 107.3% relative enthalpy efficiency and up to 99.43% quality retention after leakage test, as shown in Figure 9F[56]. Currently, LIBs will generate a lot of heat during operation; if this heat cannot be effectively managed and controlled, it will lead to reduced battery performance, shortened life and result in the risk of thermal runaway. Thermal management systems are important in LIBs, especially in electric and hybrid vehicles, to prevent hot spots from forming inside the battery and ensure that the battery operates within the temperature range for safe and optimal performance. Xu et al. used diatomite as the supporting matrix and added expanded graphite to reduce the leakage of PEG. The highest thermal conductivity of the sample with high thermal conductivity was 1.203 W·m-¹·K-¹, which was 4.02 times higher than that of pure PEG. These PPCs are applied to the battery module and are charged and discharged at 1C and 2C discharge rates, as shown in Figure 9G and H; the optimal sample battery module can maintain the temperature below 60 °C at 2C discharge rates, demonstrating good thermal management capabilities[48].
Figure 9. (A) The PEG/400CDF composite after 100/200 thermal cycles[186]; (B) Shape stability study of PCMs and biochar PCCs[187]; (C) 3D porous structure and 2D flaky morphology of biochar microparticles[188]; (D) The relative enthalpy efficiency of PCCs for 0, 100, 200, 300, 400, and 500 cycles[189]; (E) Schematic illustration of the fabrication process of PCCs[190]; (F) SEM image of CP, the calculation results of encapsulation efficiency, relative enthalpy efficiency and encapsulation effectiveness of different ND/CP samples, and leakage retention histograms of shape-stable PCMs[56]; (G) Temperature curves during ten charge/discharge cycle tests at 2C discharge rate and the schematic of the heat dissipation of battery modules; (H) The schematic of the battery modules and battery thermal management system platform[48]. PEG: polyethylene glycol; PCM: phase change material; PCC: phase change composite; 3D: three-dimensional; 2D: two-dimensional; ND: n-docosane; CP: cork powder.
In the field of thermal management of electronic devices, PCMs have attracted attention due to their unique heat storage and release characteristics, enabling a more sustainable and economical thermal management strategy. Resource-rich and environmentally friendly BDCCs usually maintain an interpermeable network structure, which is conducive to heat transfer and diffusion, thereby improving the thermal conductivity of PCCs. Combined with BDCCs, these materials can further improve the performance of PCMs and solve some problems existing in traditional PCMs, such as leakage, low thermal conductivity, weak light absorption, etc. This can not only improve the efficiency of thermal management, but also increase the sustainability of thermal management systems. Currently, the development of novel materials with multiple functions, designed to eliminate EMI and enable multi-source-driven thermal management of electronic devices, has become a general consensus in the field.
EMI
The distinction is that in EMI, magnetic-thermal conversion is usually not directly involved. The core goal of EMI is to reduce or avoid EMI by using conductive or magnetic conductive materials to block, absorb or scatter electromagnetic waves. Such materials include conductive materials (such as metal foil and metal mesh), magnetic materials, foam materials, aerogel, carbon-based materials, organic materials, intelligent EMI shielding materials, etc.[51,116,191,192], which can reduce or avoid EMI by reflecting or absorbing electromagnetic waves, effectively isolating electromagnetic waves, and providing protection for electronic devices. As shown in Figure 10, intelligent EMI shielding materials can respond to a particular external stimulus (such as pressure, temperature, chemical reagents, shape memory and phase transition, etc.), and realize the dynamic adjustment of EMI shielding effectiveness (SE). Materially, as a key component of intelligent EMI shielding materials, PCMs can adjust their electromagnetic characteristics by changing the physical state at different temperatures, and realize dynamic control of EMI shielding effect by using thermal energy absorption or release during phase transition[191,193].
Figure 10. Classification of smart EMI shielding materials[193]. EMI: electromagnetic interference.
Since PCMs will undergo phase transformation when the temperature changes, that is, from one state to another (such as from solid to liquid or gas), this process involves the absorption or release of latent heat, resulting in changes in parameters such as thermal conductivity, density and volume of the composite. These changes not only change the thermal properties of the material, but also affect its electromagnetic properties, because the propagation speed and absorption rate of electromagnetic waves in different phase states are different. Therefore, as exposited in Figure 11A, He et al. adopted a dual-encapsulation strategy, first impregnating PW into biological PC made from a loofah sponge and then coating a polyurethane layer containing Fe3O4 nanoparticles, with a synergistic effect resulting in a composite with a photothermal conversion efficiency of up to 76% and a strong EMI shielding capability of 32 dB. This dual-encapsulation method improves the sealing and leak-proof properties of the composite, while also enhancing the mechanical strength and thermal stability, with a relative enthalpy efficiency of about 84.9%, showing very low mass loss after 50 thermal cycles[194]. Shen et al. also introduced Fe3O4 nanoparticles into the composite, which improved the magnetic loss capacity. Lignin-based carbon aerogel PCMs exhibited a satisfactory EMI SE of 53.83 dB and a relative enthalpy efficiency of 95.4% at the addition of 7wt% Fe3O4 and a concentration of 7.5 mg mL-1 of graphene oxide. The structure of the horizontal plane and vertical plane of the composite are observed in Figure 11B, showing a clear and fine 3D conductive network and an oriented porous structure, which contributes to the efficient transmission of current, and improves the SE of the material against electromagnetic waves by increasing the multiple reflection and scattering of electromagnetic waves in the pores of the material[51]. Figure 11C illustrates that Cao et al. developed an innovation of biomass/MXene derivative conductive hybrid scaffold, where vacuum impregnation with PW encapsulation resulted in an EMI SE value under X-band of as high as 45.0 dB, with a corresponding melting enthalpy value of 215.7 J g-1 (relative enthalpy efficiency as 99.9%) of PCMs[191]. The design and preparation of this composite provides a new solution for efficient EMI and thermal management, especially in application scenarios requiring miniaturization, integration and high power electronics[191]. Among them, MXene is an important class of 2D materials, mainly composed of transition metal carbides, nitrides or carbon nitrides, and is only a few atomic layers thick. Because there are hydroxyl or terminal oxygen atoms on the surface of MXene, it has the metal conductive characteristics of transition metal carbides. MXene has demonstrated significant advantages in the field of EMI shielding, mainly due to its excellent electrical conductivity, tunable surface chemistry, light weight and thermal stability, which allow MXene to effectively reflect and absorb electromagnetic waves, thereby reducing interference. Additionally, due to its high mechanical flexibility and ease of processing, MXene can be made into a variety of shapes and composites to suit different shielding needs[195]. In Figure 11D, Chen et al. developed a multifunctional shape-stable PCMs based on MXene/phytic acid mixed deposition on non-carbonized wood with a thermal conductivity 4.6 times that of PEG, a solar to thermal to electrical conversion efficiency of up to 98.58% and a maximum EMI SE of 44.45 dB in the X-band. Thermal durability and stability in at least 200 heating and cooling cycles. The stacking of MXene nanosheets on the surface of wood cell walls provides moderate electrical conductivity and effective impedance matching, reducing significant reflection and enhancing absorption. Even more impressive is that the combination of MXene and phytic acid significantly reduces the flammability of the composite, giving it a self-quenching behavior that enables multifunctional applications of PCCs[192]. Oppositely, Zhu et al. used MXene aerogel as the substrate and introduced an appropriate amount of metal ions (K+) to induce the self-assembly of MXene nanosheets to form ordered arranged structures, the mechanism of which is described in Figure 11E, which not only enhanced the internal conductive network, but also improved the reflection and absorption ability of electromagnetic waves. PW was immersed in MXene/K+ aerogel by vacuum impregnation method to prepare multi-scene response PCCs, which provided a new opportunity for the development of the next generation of multifunctional PCCs[196]. In high-performance electronics and advanced energy management systems, metal foams are also used to prepare PCCs with efficient thermal management and excellent EMI shielding capabilities. Metal foam offers significant advantages in the application of EMI PCMs, including enhanced electromagnetic shielding, improved thermal management capabilities, increased thermal stability, prevention of PCM leakage, improved structural strength, and multifunctional integration features, which make metal foam an important material for boosting the performance and protection of electronic devices. As can be seen in Figure 11F, Yan et al. encapsulated PEG in the prepared Ni foam/lignin/reduced graphene oxide dual network scaffold by vacuum impregnation method. The optimal sample of the PCCs had an EMI SE of 69.9 dB (in the 8.2-12.4 GHz band), and the photothermal and electrothermal conversion capacity was high, with a relative enthalpy efficiency of 98.72%. The Ni foam in the PCC system has high conductivity, and the porous structure provides a large number of interfaces, so that the electromagnetic wave can be reflected and absorbed several times inside the material, which further boosts EMI SE. This multiple reflection and absorption mechanism not only effectively weakens the intensity of electromagnetic waves, but also converts electromagnetic energy into heat energy, thereby reducing EMI[46]. On this basis, Yan et al. used a similar preparation method to develop shape-stable PCCs with dual-network carriers. Thanks to the crosslinking of conductive fibers with PVA to form a 3D network, and then combined with Ni foam, EMI SE can reach an astonishing 110.37 dB in the X-band frequency range, as shown in Figure 11G[45]. It has far-reaching significance for multi-source-driven energy storage and electromagnetic shielding applications.
Figure 11. (A) Schematic illustration of the preparation process of dual-encapsulated multifunctional[194]. (B) SEM images in horizontal plane and in vertical plane for Lignin-based carbon aerogel PCMs[51]. (C) Schematic diagram of the preparation procedure of PCMs[191]. (D) Mechanism of hybrid-modified wood-encapsulated PCMs enabling effective solar to electricity conversion[192]. (E) Assembly structure with different K+ content[196]. (F) The EMI total shielding effectiveness (SET)[46]. (G)The EMI SET[45]. PCM: phase change material; EMI: electromagnetic interference.
Advances in next-generation communication technologies have generated elevated data transfer rates and more compact electronic components, which pose significant challenges to the stability and security of devices, thereby exacerbating EMI issues and thermal management challenges. PCMs can effectively absorb excess heat during fluctuations in ambient temperature, thus maintaining thermal stability within electronic devices and mitigating performance degradation caused by heat accumulation. Furthermore, integrating PCMs with EMI can lead to the development of a new class of multifunctional materials that not only manage and regulate heat efficiently but also provide protection against EMI, safeguarding electronic devices from adverse effects induced by external electromagnetic waves. In the domain of electromagnetic shielding, multi-source-driven PCMs exhibit significant potential. These materials, by integrating conductive networks with the porous structures of BDCCs, not only boost the absorption and reflection of electromagnetic waves but also offer additional pathways through their porous architecture to disperse and attenuate these waves effectively. BDCCs, which synergistically combine the functionalities of electromagnetic shielding and phase change energy storage, present a robust solution for thermal management and electromagnetic compatibility in electronic devices. Their implications are crucial for enhancing equipment reliability and prolonging operational lifespan.
CONCLUSION AND OUTLOOK
Biomass, as a naturally occurring material in the ecosystem, makes biochar present significant advantages by virtue of its innate resourcefulness, sustainability, elemental richness, diversified morphological structure, and cost advantages. It also reveals great potential for multi-source drive and multifunctional collaboration. Biomass is a renewable resource whose main sources include plants, animals, microorganisms and so forth. Among them, plants are the most common raw materials. In materials science, carbon materials can be categorized into 0D (e.g., fullerenes), 1D (e.g., CNTs), 2D (e.g., graphene) and 3D (e.g., porous AC) forms based on their structural dimensions. Pyrolysis, HTC, activation and template methods are commonly utilized by researchers to convert biomass into BDCCs with special microstructures and remarkable properties. These methods enable precise control of the porosity, SSA and chemical composition of the material to obtain the desired electrical, thermal and mechanical properties. Additionally, to further elevate the functionality of BDCCs, researchers also modified them by means of doping with polymers, metals or oxides. These modifications can introduce new chemically active sites to enhance the thermal conductivity or adsorption capacity of PCMs. Concurrently, by finely regulating the reaction conditions such as carbonization temperature and time, the amount of activator and the type of template, composites with specific structures and functions can be synthesized to fulfill the requirements of different application scenarios. The versatility of these composites provides a broad prospect for the high-value utilization of biomass resources. Currently, BDCCs have emerged as promising candidates for the development of high-performance PCM applications, especially in solar energy utilization, building materials, thermal management and EMI. Overall, this review describes composites with BDCCs as precursors or components and their corresponding functionalized applications, focusing on the types of materials, preparation and research progress in partial applications about energy storage and utilization of PCMs.
Figure 12. Future outlook of BDCCs: opportunities, challenges and directions. BDCC: biomass-derived carbon composite.
However, the transformation process of BDCCs from research to practical application does face a series of challenges, which involve the synthesis of materials, performance optimization, large-scale production and many other aspects. The details are as follows. (1) Typically, non-biomass carbon composites have more homogeneous physicochemical properties and sources; however, the wide range and heterogeneity of biomass feedstock sources and the cost and energy consumption of the preparation method limit large-scale application in PCMs; (2) The most critical and immediate problem is that the preparation process of BDCCs requires fine control to ensure the specific microstructure and properties of the desired composite, thus preventing leakage of the phase change matrix through the strong capillary force of the porous structure; (3) The activation process necessitates the utilization of potent corrosive reagents and the release of noxious gases, which can result in environmental contamination and deleterious effects on the ecosystem. To address these challenges effectively and promote the large-scale application of BDCCs in PCMs, several strategies need to be implemented. (1) A standardized and efficient biomass collection and management system must be established to provide a stable source of high-quality biochar for manufacturing PCMs. For instance, develop quality standards for biomass raw materials, including key parameters such as moisture content, ash content, calorific value, etc., and then establish a classification and grading system for biomass feedstocks to meet the production needs of various BDCCs; (2) The exploration of universal carbonization methods is the key to eliminating differences between similar biomass, which may involve the study of the pyrolysis behavior and chemical changes of different biomass feedstocks during the carbonization process to determine the optimal carbonization conditions that can help improve the production efficiency and quality consistency of BDCCs; (3) Adopt green activators instead of traditional corrosive reagents and use enzyme- or microbe-assisted activation to pre-treat biomass, thereby optimizing the preparation process to control reaction conditions. Alternatively, develop greener emerging technologies to avoid environmental problems. As illustrated in Figure 12, the development of BDCCs is characterized by a range of opportunities, challenges and directions. With multiple strengths in the PCM energy storage, biochar can further promote both the energy storage efficiency and application versatility of PCMs by optimizing comprehensive performance of BDCCs, thereby positively influencing the entire energy consumption structure. As the continuous development and expansion of the corresponding applications of BDCCs, exploring and addressing the above challenges still remains a critical direction for future researchers.
DECLARATIONS
Acknowledgments
This work was supported by the National Natural Science Foundation of China (Grant Nos. U20A20340, 52003111 and 21975054), Guangzhou Hongmian Project (HMJH-2020-0012), Faculty of Health Sciences, University of Macau, the Multi-Year Research Grant (MYRG) of University of Macau and the University of Macau Development Foundation (UMDF) (MYRG2022-00011-FHS and MYRG-GRG2023-00013-FHS-UMDF), the Science and Technology Development Fund, Macau SAR (0103/2021/A, 0002/2021/AKP, 0133/2022/A3, 0009/2022/AKP, and 0006/2023/ITP1), Dr. Stanley Ho Medical Development Foundation (SHMDF-OIRFS/2022/002), and Ministry of Education Frontiers Science Centre for Precision Oncology, University of Macau (SP2023-00001-FSCPO), National Key R&D Program of China (2020YFB0408100), Anhui Provincial Key Research and Development Project (2023z04020021), the Program for Guangdong Introducing Innovative and Entrepreneurial Team (2016ZT06C412), and Foshan Science and Technology Innovation Team Project (1920001000108).
Authors’ contributions
Investigation: Liu, X. H.
Conceptualization: Liu, X. H.; Huang, J. T.
Visualization: Liu, X. H.
Writing original draft: Liu, X. H.
Writing-review & editing: Liu, X. H.; Huang, J. T.
Validation: Lin, J. H.
Formal analysis Lin, J. H.; Huang, J. T.
Methodology Lin, J. H.; Zhao, Z. Z.
Funding acquisition: Song, J. J.; Min, Y. G.; Li, B.
Supervision: Li, B.; Huang, J. T.
Availability of data and materials
Not applicable.
Financial support and sponsorship
None.
Conflicts of interest
Jianjun Song is affiliated with Guangzhou Liangyue Materials Technology Co., Ltd., while the other authors have declared that they have no conflicts of interest.
Ethical approval and consent to participate
Not applicable.
Consent for publication
Not applicable.
Copyright
© The Author(s) 2025.
REFERENCES
1. Qiu, W.; Hao, Q.; Annamareddy, S. H. K.; Xu, B. B.; Guo, Z.; Jiang, Q. Electric Vehicle revolution and implications: ion battery and energy. Eng. Sci. 2022, 20, 100-9.
2. Hussain, S. A.; Razi, F.; Hewage, K.; Sadiq, R. The perspective of energy poverty and 1st energy crisis of green transition. Energy 2023, 275, 127487.
3. Riahi, A.; Shafii, M. B. Experimental evaluation of a vapor compression cycle integrated with a phase change material storage tank for peak load shaving. Eng. Sci. 2023, 23, 870.
4. Zhakiyev, N.; Akhmetov, Y.; Omirgaliyev, R.; et al. Comprehensive scenario analyses for coal exit and renewable energy development planning of Kazakhstan using PyPSA-KZ. Eng. Sci. 2024, 29, 1085.
5. Olabi, A.; Abdelkareem, M. A. Renewable energy and climate change. Renew. Sust. Energ. Rev. 2022, 158, 112111.
6. Lin, B.; Li, Z. Towards world's low carbon development: The role of clean energy. Appl. Energy. 2022, 307, 118160.
7. Iyke, B. N. Climate change, energy security risk, and clean energy investment. Energy. Econ. 2024, 129, 107225.
8. Hussain, A.; Arif, S. M.; Aslam, M. Emerging renewable and sustainable energy technologies: State of the art. Renew. and. Sust. Energy. Rev. 2017, 71, 12-28.
9. Jiang, H.; Wu, X.; Zhang, H.; et al. Toward effective electrocatalytic C-N coupling for the synthesis of organic nitrogenous compounds using CO 2 and biomass as carbon sources. SusMat 2023, 3, 781-820.
10. Rebellon, H. E.; Henao, O. F. P.; Gutierrez-velasquez, E. I.; Amell, A. A.; Colorado, H. A. Eng Sci 2024;29:1164.
11. Srivastava, M.; Surana, K.; Singh, P. K.; Singh, R. C. Nickel oxide embedded with polymer electrolyte as efficient hole transport material for perovskite solar cell. Eng. Sci. 2021, 17, 216-23.
12. Li, C. H.; Feng, M. J.; Guo, F.; et al. The evolution of tin-based perovskites solar cells. Eng. Sci. 2022, 19, 1-4.
13. Zhou, Y. F.; Feng, W. F.; Xu, Y. B.; et al. Development of silicon-based anode for lithium-ion batteries and its application in solid-state electrolytes. Eng. Sci. 2023, 28, 1060.
14. Boshoman, S. B.; Fatoba, O. S.; Jen, T. C. Transition metal oxides as electrocatalytic material in fuel cells: a review. Eng. Sci. 2023, 25, 948.
15. Zhong, Y. M.; Liu, D. C.; Yang, Q. Y.; et al. Boosting microwave absorption performance of bio-gel derived Co/C nanocomposites. Eng. Sci. 2023. DOI: 10.30919/es988.
16. Chandra RB, Shivamurthy B, Bore Gowda SB, Sathish kumar M. Flexible linear low-density polyethylene laminated aluminum and nickel foil composite tapes for electromagnetic interference shielding. Eng. Sci. 2022. DOI: 10.30919/es8d777.
17. Deng, B.; He, R.; Zhang, J.; et al. Interfacial modulation of a self-sacrificial synthesized SnO2@Sn core-shell heterostructure anode toward high-capacity reversible Li+ storage. Inorg. Chem. 2023, 62, 15736-46.
18. Wang, J.; Zhang, J.; Zhang, Y. Z.; et al. Atom-level tandem catalysis in lithium metal batteries. Adv. Mater. 2024, 36, e2402792.
19. Tong, X.; Li, N. Q.; Zeng, M.; Wang, Q. W. Organic phase change materials confined in carbo-based materials for thermal properties enhancement: recent advancement and challenges. Renew. Sust. Energy. Rev. 2019, 108, 398-422.
20. Chai, Z. Z.; Fang, M. H.; Min, X. Composite phase-change materials for photo-thermal conversion and energy storage: a review. Nano. Energy. 2024, 124, 109437.
21. Zhou, Z. X. Y.; Huang, Y. Q.; Shen, Q.; Li, Y. Y.; Cheng, X. M. Composite phase change materials with carbon-mesh/CuS/ZnO interface biocarbon skeleton for solar energy storage, solar photocatalysis and electromagnetic shielding. J. Energy. Storage. 2024, 90, 111937.
22. Rahimi, E.; Babapoor, A.; Moradi, G.; Kalantary, S.; Monazzam, E. M. Personal cooling garments and phase change materials: a review. Renew. Sust. Energy. Rev. 2024, 190, 114063.
23. Zheng, C. W.; Huang, Z. Y.; Wang, D. Y.; et al. Synthesis and properties of biomass derived carbon/PEG composite as photothermal conversion effective phase change material for functional concrete. Cement. Concrete. Comp. 2024, 149, 105495.
24. Su, J. T.; Lin, J. H.; Cao, Y.; et al. Experimental investigation and numerical simulation on microwave thermal conversion storage properties of multi-level conductive porous phase change materials and its multifunctional applications. Appl. Therm. Eng. 2024, 253, 123774.
25. Chen, X.; Cheng, P.; Tang, Z. D.; Xu, X. L.; Gao, H. Y.; Wang, G. Carbon-based composite phase change materials for thermal energy storage, transfer, and conversion. Adv. Sci. (Weinh). 2021, 8, 2001274.
26. Sathishkumar, A.; Sundaram, P.; Cheralathan, M.; Kumar, P. G. Effect of nano-enhanced phase change materials on performance of cool thermal energy storage system: a review. J. Energy. Storage. 2024, 78, 110079.
27. Yu, C. B.; Li, G. Q. Electrical energy harvesting by connected form stable phase change material composites. Energy. Convers. Manage. 2024, 299, 117851.
28. Naik, N.; Shivamurthy, B.; Thimmappa, B. H. S.; Gupta, A.; Guo, J. Z.; Seok, I. A review on processing methods and characterization techniques of green composites. Eng. Sci. 2022, 20, 80-99.
29. Rengga, W. D. P.; Imani, N. A. C.; Wijayati, N.; Tohiran, T.; Cahyati, W. H.; Saputra, D. Adsorption and release of ibuprofen from silica-carbon composites based on rice husks (Oryza Sativa) and banana peels (Musa Acuminata). Eng. Sci. 2023, 23, 873.
30. Zhou, M.; Wang, J. W.; Zhao, Y.; Wang, G. H.; Gu, W. H.; Ji, G. B. Hierarchically porous wood-derived carbon scaffold embedded phase change materials for integrated thermal energy management, electromagnetic interference shielding and multifunctional application. Carbon 2021, 183, 515-24.
31. Tiwari, S. K.; Bystrzejewski, M.; De, A. A.; Huczko, A.; Wang, N. N. Methods for the conversion of biomass waste into value-added carbon nanomaterials: Recent progress and applications. Prog. Energy. Combust. Sci. 2022, 92, 101023.
32. Thota, S. P.; Bag, P. P.; Vadlani, P. V.; Belliraj, S. K. Plant biomass derived multidimensional nanostructured materials: a green alternative for energy storage. Eng. Sci. 2022, 18, 31-58.
33. He, H. Z.; Zhang, R. Q.; Zhang, P. C.; et al. Functional carbon from nature: biomass-derived carbon materials and the recent progress of their applications. Adv. Sci. (Weinh). 2023, 10, e2205557.
34. Zhou, H.; Zhang, R.; Yue, C. Y.; et al. Enhanced charge transfer over sustainable biochar decorated Bi2WO6 composite photocatalyst for highly efficient water decontamination. Chin. J. Catal. 2024, 59, 169-84.
35. Culebras, M.; Collins, G. A.; Beaucamp, A.; Geaney, H.; Collins, M. N. Lignin/Si hybrid carbon nanofibers towards highly efficient sustainable Li-ion anode materials. Eng. Sci. 2022, 17, 195-203.
36. Meng, L. T.; Hou, C. P.; Hou, J.; et al. Preparation and performance of in situ carbon-coated silicon monoxide@C@carbon microspheres composite anode material for lithium-ion batteries. Eng. Sci. 2022, 20, 134-43.
37. Xing, B. L.; Shi, F.; Jin, Z. Z.; et al. A facile ice-templating-induced puzzle coupled with carbonization strategy for kilogram-level production of porous carbon nanosheets as high‐capacity anode for lithium‐ion batteries. Carbon. Energy. 2024, 6, e633.
38. Sun, Z.; Qi, H. G.; Chen, M. H.; et al. Progress in cellulose/carbon nanotube composite flexible electrodes for supercapacitors. Eng. Sci. 2021, 18, 59-74.
39. Liu, B.; Ye, Y.; Yang, M.; et al. All-in-one biomass-based flexible supercapacitors with high rate performance and high energy density. Adv. Funct. Mater. 2024, 34, 2310534.
40. Fu, C.; Wang, J. J.; Shen, Q. F.; Cui, Z. H.; Ding, S. Determining the optimal biomass-gasification-based fuel cell trigeneration system in exergy-based cost and carbon footprint method considering energy level. Energy. Convers. Manage. 2024, 299, 117802.
41. Qiu, L. L.; Xu, M.; Tian, W. Y.; et al. Biomass derived self-doped carbon nanosheets enable robust hole transport layers with ion buffer for perovskite solar cells. ChemSusChem 2025, 18, e202400510.
42. Lin, J.; Feng, X.; Huang, J.; et al. Flexible AIE/PCM composite fiber with biosensing of alcohol, fluorescent anti-counterfeiting and body thermal management functions. Biosens. Bioelectron. 2025, 267, 116799.
43. Ma, Y. Q.; Shen, J. F.; Li, T.; Sheng, X. X.; Chen, Y. A “net-ball” structure fiber membrane with electro-/photo-thermal heating and phase change synchronous temperature regulation capacity via electrospinning. Sol. Energy. Mater. Sol. Cells. 2024, 276, 113078.
44. Xu, W. H.; Su, J. T.; Lin, J. H.; Huang, J. T.; Weng, M. M.; Min, Y. G. Enhancing the light-thermal absorption and conversion capacity of diatom-based biomass/polyethylene glycol composites phase change material by introducing MXene. J. Energy. Storage. 2023, 72, 108253.
45. Yan, R. H.; Huang, Z.; Zhang, L.; Chen, Y.; Sheng, X. X. Cellulose-reinforced foam-based phase change composites for multi-source driven energy storage and EMI shielding. Compos. Commun. 2024, 51, 102047.
46. Yan, R. H.; Huang, Z.; Chen, Y.; Zhang, L.; Sheng, X. X. Phase change composite based on lignin carbon aerogel/nickel foam dual-network for multisource energy harvesting and superb EMI shielding. Int. J. Biol. Macromol. 2024, 277, 134233.
47. Hu, X. P.; Wu, H.; Liu, S.; et al. Fabrication of organic shape-stabilized phase change material and its energy storage applications. Eng. Sci. 2021, 17 , 1-27.
48. Xu, W. H.; Yang, W.; Su, J.; et al. Diatom-based biomass composites phase change materials with high thermal conductivity for battery thermal management. J. Energy. Storage. 2024, 96, 112737.
49. Liu, B. W.; Lv, G. C.; Liu, T. M.; et al. Research progress of biomass materials in the application of organic phase change energy storage materials. J. Mater. Chem. A. 2024, 12, 8663-82.
50. Wu, G. Z.; Bing, N. C.; Li, Y. F.; Xie, H. Q.; Yu, W. Three-dimensional directional cellulose-based carbon aerogels composite phase change materials with enhanced broadband absorption for light-thermal-electric conversion. Energy. Convers. Manage. 2022, 256, 115361.
51. Shen, R. B.; Weng, M. M.; Zhang, L.; Huang, J. T.; Sheng, X. X. Biomass-based carbon aerogel/Fe3O4@PEG phase change composites with satisfactory electromagnetic interference shielding and multi-source driven thermal management in thermal energy storage. Compos. Part. A-Appl. S. 2022, 163, 107248.
52. Weng, M. M.; Liu, S. D.; Su, J. T.; et al. Hydrophobic and antimicrobial polyimide based composite phase change materials with thermal energy storage capacity, applied as multifunctional construction material. Eng. Sci. 2022, 19, 301-11.
53. Weng, M. M.; Lin, J. H.; Yang, Y. J.; et al. MXene-based phase change materials for multi-source driven energy storage, conversion and applications. Sol. Energy. Mater. Sol. Cells. 2024, 272, 112915.
54. He, X. L.; Cui, C. Q.; Chen, Y.; Zhang, L.; Sheng, X. X.; Xie, D. L. MXene and polymer collision: sparking the future of high‐performance multifunctional coatings. Adv. Funct. Mater. 2024, 34, 2409675.
55. Wang, T.; Cui, W.; Li, X. X.; Ma, T.; Wang, Q. W. Economical and shape-stabilized hydrated salt/bagasse biomass-derived carbon phase change composite for thermal energy storage. J. Energy. Storage. 2024, 85, 111083.
56. Luo, Y.; Tao, J.; Shan, Y. Z.; et al. A shape-stabilized phase change composite from biomass cork powder as a matrix for thermal energy storage and photothermal conversion. J. Energy. Storage. 2024, 77, 109914.
57. Xiong, C. Y.; Zheng, C. M.; Jiang, X.; et al. Recent progress of green biomass based composite materials applied in supercapacitors, sensors, and electrocatalysis. J. Energy. Storage. 2023, 72, 108633.
58. Hegde, S. S.; Bhat, B. R. Biomass waste-derived porous graphitic carbon for high-performance supercapacitors. J. Energy. Storage. 2024, 76, 109818.
59. Hao, R.; Yang, Y.; Wang, H.; et al. Direct chitin conversion to N-doped amorphous carbon nanofibers for high-performing full sodium-ion batteries. Nano. Energy. 2018, 45, 220-8.
60. Chaudhary, P.; Bansal, S.; Sharma, B. B.; Saini, S.; Joshi, A. Waste biomass-derived activated carbons for various energy storage device applications: a review. J. Energy. Storage. 2024, 78, 109996.
61. Atchudan, R.; Jebakumar, I. E. T. N.; Perumal, S.; et al. Facile synthesis of nitrogen-doped porous carbon materials using waste biomass for energy storage applications. Chemosphere 2022, 289, 133225.
62. Qiu, D. P.; Kang, C. H.; Li, M.; et al. Biomass-derived mesopore-dominant hierarchical porous carbon enabling ultra-efficient lithium-ion storage. Carbon 2020, 162, 595-603.
63. Radenković, M.; Petrović, J.; Pap, S.; et al. Waste biomass derived highly-porous carbon material for toxic metal removal: optimisation, mechanisms and environmental implications. Chemosphere 2024, 347, 140684.
64. Li, H. M.; Ma, Y. F.; Wang, Y.; et al. Nitrogen enriched high specific surface area biomass porous carbon: A promising electrode material for supercapacitors. Renew. Energy. 2024, 224, 120144.
66. Mohan, A.; Jaison, A.; Lee, H. U.; et al. Facile synthesis of N-doped biomass derived porous carbon from opuntia humifusa using simple solid state activation method for reversible capture of volatile iodine. J. Anal. Appl. Pyrolysis. 2024, 179, 106473.
68. Yu, C. L.; Dan, J. H.; Liu, Z. W.; et al. A facile, green strategy to synthesize N/P self-doped, biomass-derived, hierarchical porous carbon from water hyacinth for efficient VOCs adsorption. Fuel 2024, 358, 130136.
69. Lobato-peralta, D. R.; Arreola-ramos, C. E.; Ayala-cortés, A.; et al. Optimizing capacitance performance: solar pyrolysis of lignocellulosic biomass for homogeneous porosity in carbon production. J. Cleaner. Prod. 2024, 448, 141622.
70. Yang, Z.; Liu, X.; Ma, X.; et al. Efficient Preparation of biomass-based ultra-thin 2D porous carbon materials by in situ template-activation and its application in sodium ion capacitors. Adv. Funct. Mater. 2024, 34, 2310717.
71. Chen, Y. Y.; Liao, Y.; Qing, Y.; et al. Recent advances in plant-derived porous carbon for lithium-sulfur batteries. J. Energy. Storage. 2024, 99, 113186.
72. Chen, Z. K.; Jiang, X. L.; Boyjoo, Y.; et al. Nanoporous carbon materials derived from biomass precursors: sustainable materials for energy conversion and storage. Electrochem. Energy. Rev. 2024, 7, 223.
73. Xu, M. M.; Fu, S. Q.; Wen, Y. K.; et al. Self-templating synthesis of biomass-based porous carbon nanotubes for energy storage and catalytic degradation applications. Green. Energy. Environ. 2024, 9, 584-95.
74. Zago, S.; Scarpetta-pizo, L. C.; Zagal, J. H.; Specchia, S. PGM-Free biomass-derived electrocatalysts for oxygen reduction in energy conversion devices: promising materials. Electrochem. Energy. Rev. 2024, 7, 197.
75. Niu, J.; Shao, R.; Liu, M. Y.; et al. Porous carbons derived from collagen-enriched biomass: tailored design, synthesis, and application in electrochemical energy storage and conversion. Adv. Funct. Mater. 2019, 29, 1905095.
76. Priya, D. S.; Kennedy, L. J.; Anand, G. T. Emerging trends in biomass-derived porous carbon materials for energy storage application: a critical review. Mater. Today. Sustainability. 2023, 21, 100320.
77. Tarimo, D. J.; Oyedotun, K. O.; Sylla, N. F.; Mirghni, A. A.; Ndiaye, N. M.; Manyala, N. Waste chicken bone-derived porous carbon materials as high-performance electrode for supercapacitor applications. J. Energy. Storage. 2022, 51, 104378.
78. Yang, H. W.; Lin, H. H.; Yang, C. L.; et al. Structural regulation of carbon materials through hydrothermal mixing of biomass and its application in supercapacitors. J. Energy. Storage. 2024, 83, 110688.
79. Yuan, F.; Wu, D. L.; Guo, J.; Liu, Q.; Wang, T. Fermentation assisted preparation of O and N riched porous carbon for high performance flexible supercapacitors. Appl. Surf. Sci. 2023, 616, 156525.
80. Egun, I. L.; Liu, Z. X.; Zheng, Y. Y.; et al. Turning waste tyres into carbon electrodes for batteries: exploring conversion methods, material traits, and performance factors. Carbon. Energy. 2024, 6, e571.
81. Shen, G. Y.; Li, B. C.; Xu, Y. Y.; et al. Waste biomass garlic stem-derived porous carbon materials as high-capacity and long-cycling anode for lithium/sodium-ion batteries. J. Colloid. Interface. Sci. 2024, 653, 1588-99.
82. Durán-jiménez, G.; Rodriguez, J.; Stevens, L.; Kostas, E. T.; Dodds, C. Microwave pyrolysis of waste biomass and synthesis of micro-mesoporous activated carbons: The role of textural properties for CO2 and textile dye adsorption. Chem. Eng. J. 2024, 488, 150926.
83. Samage, A.; Halakarni, M.; Yoon, H.; Sanna, K. N. Sustainable conversion of agricultural biomass waste into electrode materials with enhanced energy density for aqueous zinc-ion hybrid capacitors. Carbon 2024, 219, 118774.
84. Zhang, X. H.; Han, R. Y.; Liu, Y. Z.; et al. Porous and graphitic structure optimization of biomass-based carbon materials from 0D to 3D for supercapacitors: a review. Chem. Eng. J. 2023, 460, 141607.
85. Feng, Y.; Jiang, J. J.; Xu, Y. X.; et al. Biomass derived diverse carbon nanostructure for electrocatalysis, energy conversion and storage. Carbon 2023, 211, 118105.
86. Gan, J.; Chen, L. Z.; Chen, Z. J.; et al. Lignocellulosic biomass-based carbon dots: synthesis processes, properties, and applications. Small 2023, 19, e2304066.
87. Selvaraj, A. R.; Muthusamy, A.; Inho-cho; Kim, H.; Senthil, K.; Prabakar, K. Ultrahigh surface area biomass derived 3D hierarchical porous carbon nanosheet electrodes for high energy density supercapacitors. Carbon 2021, 174, 463-74.
88. Gao, J.; Wang, Z. Q.; Wang, Z. F.; et al. Biomass-based controllable morphology of carbon microspheres with multi-layer hollow structure for superior performance in supercapacitors. J. Colloid. Interface. Sci. 2024, 658, 90-9.
89. Bahadur, R.; Singh, G.; Li, Z.; et al. Hybrid nanoarchitectonics of ordered mesoporous C60-BCN with high surface area for supercapacitors and lithium-ion batteries. Carbon 2024, 216, 118568.
90. An, Y.; Ren, Z. J.; Kong, Y.; Tian, Y. C.; Jiang, B.; Shaik, F. Fluorine-based multi-halogen atom doped vinasse carbon quantum dots on vertical graphene: a bifunctional catalytic electrode for water splitting. Int. J. Hydrogen. Energy. 2024, 58, 633-45.
91. Lu, J.; Veksha, A.; Lisak, G. Conversion of municipal sewage sludge into biogenic multi-walled carbon nanotubes and hydrogen using X-Mo/MgO (X = Co, Fe, Ni) catalysts through pyrolysis-chemical vapor deposition process. Chem. Eng. J. 2024, 496, 153794.
92. Hasan M, Sayantha Aniv S, Mominul Islam M. Carbon nanosheets-based supercapacitor materials: recent advances and prospects. Chem. Rec. 2024, 24, e202300153.
93. Pandey, M.; Deshmukh, K.; Raman, A.; Asok, A.; Appukuttan, S.; Suman, G. Prospects of MXene and graphene for energy storage and conversion. Renew. Suste. Energy. Rev. 2024, 189, 114030.
94. Fang, Y.; Liu, Y. X.; Qi, L.; Xue, Y. R.; Li, Y. L. 2D graphdiyne: an emerging carbon material. Chem. Soc. Rev. 2022, 51, 2681-709.
95. Yang, H.; Yin, J.; Yang, J. T.; Tang, S. B.; Zhang, W. L.; Yang, G. X. From macro to micro: biomass-derived advanced carbon microtube assembly for sodium-ion batteries. Nano. Energy. 2024, 125, 109591.
96. Wu, Q. P.; Chen, K. Y.; Shadike, Z.; Li, C. L. Relay-type catalysis by a dual-metal single-atom system in a waste biomass derivative host for high-rate and durable Li-S batteries. ACS. Nano. 2024, 18, 13468-83.
97. Ma, Q. H.; Wang, Z. P.; Zhang, L. Y.; et al. Marrying Fe nanoclusters with 3D carbon nanofiber aerogels: triggering fast and robust faradic capacitive deionization. Sep. Purif. Technol. 2025, 353, 128503.
98. Liang, C.; Xia, H. Y.; Yin, L. H.; et al. Carbon foam directly synthesized from industrial lignin powder as featured material for high efficiency solar evaporation. Chem. Eng. J. 2024, 481, 148375.
99. Hao, X. X.; Li, D.; Peng, X. W.; Lan, W.; Liu, C. F. In situ construction of biomass derived 3D carbon framework for efficient electromagnetic interference shielding and Joule heating performance. Chem. Eng. J. 2024, 479, 147681.
100. Ding, L.; Sun, L.; Yu, J. K.; et al. 0D bio-based carbon dots and 2D MXene hybridization toward fabricating flame-retardant, conductive and sensing cellulose fabrics. Chem. Eng. J. 2024, 488, 150776.
101. Chen, Q.; Tan, X. F.; Liu, Y. G.; et al. Biomass-derived porous graphitic carbon materials for energy and environmental applications. J. Mater. Chem. A. 2020, 8, 5773-811.
102. Tang, X. F.; Liu, D.; Wang, Y. J.; et al. Research advances in biomass-derived nanostructured carbons and their composite materials for electrochemical energy technologies. Prog. Mater. Sci. 2021, 118, 100770.
103. Wang, Y. L.; Zhang, M. C.; Shen, X. Y.; et al. Biomass-derived carbon materials: controllable preparation and versatile applications. Small 2021, 17, e2008079.
104. Rawat, S.; Mishra, R. K.; Bhaskar, T. Biomass derived functional carbon materials for supercapacitor applications. Chemosphere 2022, 286, 131961.
105. Mujtaba, M.; Fernandes, F. L.; Fazeli, M.; et al. Lignocellulosic biomass from agricultural waste to the circular economy: a review with focus on biofuels, biocomposites and bioplastics. J. Cleaner. Prod. 2023, 402, 136815.
106. Kim, J. H.; Lee, T.; Tsang, Y. F.; Moon, D. H.; Lee, J.; Kwon, E. E. Functional use of carbon dioxide for the sustainable valorization of orange peel in the pyrolysis process. Sci. Total. Environ. 2024, 941, 173701.
107. Lee, S.; Lee, T.; Cha, H.; et al. Enhancement of syngas through integrating carbon dioxide in the catalytic pyrolysis of plantation waste. Energy. Convers. Manag. 2024, 311, 118554.
108. Varma, A. K.; Mondal, P. Pyrolysis of sugarcane bagasse in semi batch reactor: effects of process parameters on product yields and characterization of products. Industrial. Crops. and. Products. 2017, 95, 704-17.
109. Dai, L.; Wang, Y.; Liu, Y.; et al. Integrated process of lignocellulosic biomass torrefaction and pyrolysis for upgrading bio-oil production: A state-of-the-art review. Renewable. and. Sustainable. Energy. Reviews. 2019, 107, 20-36.
110. Zhang, Y. N.; Liang, Y. Y.; Li, S. Y.; et al. A review of biomass pyrolysis gas: forming mechanisms, influencing parameters, and product application upgrades. Fuel 2023, 347, 128461.
111. Dhyani, V.; Bhaskar, T. A comprehensive review on the pyrolysis of lignocellulosic biomass. Renew. Energy. 2018, 129, 695-716.
112. Li, J. S.; Xu, K. L.; Yao, X. W.; Liu, J. Investigation of biomass slow pyrolysis mechanisms based on the generation trends in pyrolysis products. Process. Saf. Environ. 2024, 183, 327-38.
113. Liu, J. M.; Xue, X. F.; Qin, Z. X.; Yang, Y. N.; Liang, J. Aromatic bio-oil production from corn stover by FAU + MFI dual-catalyst catalytic fast pyrolysis: a study of two catalyst parameters. Fuel 2024, 367, 131499.
114. Patel, H.; Mohanty, A.; Misra, M. Post-combustion CO2 capture using biomass based activated porous carbon: Latest advances in synthesis protocol and economics. Renew. Sust. Energy. Rev. 2024, 199, 114484.
115. Chen, H. R.; Xu, H.; Zhu, H. N.; et al. A review on bioslurry fuels derived from bio-oil and biochar: preparation, fuel properties and application. Fuel 2024, 355, 129283.
116. Lin, J. H.; Huang, J. T.; Guo, Z. H.; et al. Hydrophobic multilayered PEG@PAN/MXene/PVDF@SiO2 composite film with excellent thermal management and electromagnetic interference shielding for electronic devices. Small 2024, 20, e2402938.
117. Tan, X. F.; Liu, S. B.; Liu, Y. G.; et al. Biochar as potential sustainable precursors for activated carbon production: multiple applications in environmental protection and energy storage. Bioresour. Technol. 2017, 227, 359-72.
118. Heidarinejad, Z.; Dehghani, M. H.; Heidari, M.; Javedan, G.; Ali, I.; Sillanpää, M. Methods for preparation and activation of activated carbon: a review. Environ. Chem. Lett. 2020, 18, 393-415.
119. Luo, L.; Lan, Y. L.; Zhang, Q. Q.; et al. A review on biomass-derived activated carbon as electrode materials for energy storage supercapacitors. J. Energy. Storage. 2022, 55, 105839.
120. Ullah, S.; Shah, S. S. A.; Altaf, M.; et al. Activated carbon derived from biomass for wastewater treatment: synthesis, application and future challenges. J. Anal. Appl. Pyrolysis. 2024, 179, 106480.
121. Pallarés, J.; González-cencerrado, A.; Arauzo, I. Production and characterization of activated carbon from barley straw by physical activation with carbon dioxide and steam. Biomass. Bioenerg. 2018, 115, 64-73.
122. Li, X. H.; Huang, Z. H.; Shao, S. S.; Cai, Y. X. Machine learning prediction of physical properties and nitrogen content of porous carbon from agricultural wastes: effects of activation and doping process. Fuel 2024, 356, 129623.
123. Gowthami, D.; Sharma, R.; Tyagi, V.; Rathore, P. K. S.; Sarı, A. Development of a novel form-stable phase change material based on alkali activated date seed biochar to harvest solar thermal energy. J. Energy. Storage. 2024, 83, 110699.
124. Jin, C. B.; Nai, J. W.; Sheng, O. W.; et al. Biomass-based materials for green lithium secondary batteries. Energy. Environ. Sci. 2021, 14, 1326-79.
125. Yang, F. Y.; Cao, S. A.; Tang, Y. J.; Yin, K. L.; Gao, Y. J.; Pang, H. HCl-activated porous nitrogen-doped carbon nanopolyhedras with abundant hierarchical pores for ultrafast desalination. J. Colloid. Interface. Sci. 2022, 628, 236-46.
126. Hao, J. Y.; Wang, B. X.; Xu, H.; et al. Interfacial regulation of biomass-derived carbon towards high-performance supercapacitor. J. Energy. Storage. 2024, 86, 111301.
127. Zhao, Y. J.; Liu, X. T.; Li, W. H.; et al. One-step synthesis of garlic peel derived biochar by concentrated sulfuric acid: enhanced adsorption capacities for Enrofloxacin and interfacial interaction mechanisms. Chemosphere 2022, 290, 133263.
128. Ren, W. D.; Li, C.; Fan, M. J.; Wang, Y.; Li, Q. Y.; Hu, X. Production of poplar branch-derived activated carbon with acidic deep eutectic solvent pretreatment coupled with chemical activation. Fuel 2025, 381, 133417.
129. Deng, L. H.; Zhao, Y. J.; Sun, S. Z.; Feng, D. D.; Zhang, W. D. Preparation of corn straw-based carbon by “carbonization-KOH activation” two-step method: gas-solid product characteristics, activation mechanism and hydrogen storage potential. Fuel 2024, 358, 130134.
130. Romero-hernandez, J. J.; Paredes-laverde, M.; Silva-agredo, J.; Mercado, D. F.; Ávila-torres, Y.; Torres-palma, R. A. Pharmaceutical adsorption on NaOH-treated rice husk-based activated carbons: kinetics, thermodynamics, and mechanisms. J. Cleaner. Prod. 2024, 434, 139935.
131. Wu, W. J.; Wu, C. L.; Zhang, G. J.; Liu, J.; Li, Y. L.; Li, G. Q. Synthesis and characterization of magnetic K2CO3-activated carbon produced from bamboo shoot for the adsorption of Rhodamine b and CO2 capture. Fuel 2023, 332, 126107.
132. Kong, L. H.; Li, C.; Sun, R. X.; et al. Thermal pretreatment of willow branches impacts yield and pore development of activated carbon in subsequent activation with ZnCl2 via modifying cellulose structure. Chin. J. Chem. Eng. 2024, 69, 227-37.
133. Zhong, R. Q.; Zhang, H. X.; Zhang, Y. L.; Yue, P.; Wu, X. L. KMnO4-assisted synthesis of hierarchical porous carbon with ultrahigh capacitance for supercapacitor. J. Energy. Storage. 2022, 51, 104346.
134. Vignesh, K.; Ganeshbabu, M.; Puneeth, N. P. N.; et al. Oxygen-rich functionalized porous carbon by KMnO4 activation on pods of prosopis juliflora for symmetric supercapacitors. J. Energy. Storage. 2023, 72, 108216.
135. Tian, X. D.; Chen, Z. C.; Hou, J.; Li, Z. Q. Electrochemical properties of porous carbon derived from coal gasification fine ash via low-temperature alkaline fusion and KOH activation. J. Energy. Storage. 2024, 75, 109557.
136. Zhai, Z. Z.; Wang, S. S.; Xu, Y. L.; et al. Starch-based carbon aerogels prepared by an innovative KOH activation method for supercapacitors. Int. J. Biol. Macromol. 2024, 257, 128587.
137. Qw, Zhang Hj, Li Yl, Zhang Xg, Pan D. Multifunctional response of biomass carbon/sodium sulfate decahydrate composite phase change materials. J. Energy. Storage. 2024, 83, 110621.
138. Chen, W.; Gong, M.; Li, K. X.; et al. Insight into KOH activation mechanism during biomass pyrolysis: Chemical reactions between O-containing groups and KOH. Appl. Energy. 2020, 278, 115730.
139. Singh, G.; Maria, R. A.; Geng, X.; Vinu, A. Recognizing the potential of K-salts, apart from KOH, for generating porous carbons using chemical activation. Chem. Eng. J. 2023, 451, 139045.
140. Xu, Z. X.; Ma, X. Q.; Zhou, J.; et al. The influence of key reactions during hydrothermal carbonization of sewage sludge on aqueous phase properties: a review. J. Anal. Appl. Pyrolysis. 2022, 167, 105678.
141. Yu, S. J.; He, J. K.; Zhang, Z. E.; et al. Towards negative emissions: hydrothermal carbonization of biomass for sustainable carbon materials. Adv. Mater. 2024, 36, e2307412.
142. González-arias, J.; Sánchez, M. E.; Cara-jiménez, J.; Baena-moreno, F. M.; Zhang, Z. Hydrothermal carbonization of biomass and waste: a review. Environ. Chem. Lett. 2022, 20, 211-21.
143. Wang, T. F.; Zhai, Y. B.; Zhu, Y.; Li, C. T.; Zeng, G. M. A review of the hydrothermal carbonization of biomass waste for hydrochar formation: process conditions, fundamentals, and physicochemical properties. Renew. Sust. Energy. Rev. 2018, 90, 223-47.
144. Lilian, M. J.; Bissessur, R.; Kang, K.; He, Q. S.; Hu, Y. Study of KOH-activated hydrochar for CO2 adsorption. J. Ind. Eng. Chem. 2025, 143, 240-51.
145. Zhang, P.; Chen, Y. X.; Song, X. T.; Zhang, H. R.; Cui, J. L.; Wang, B. F. Preparation of hierarchical porous carbon from corncob hydrochar by KCl enhancing K2CO3 activation for electrode material of supercapacitor. Chem. Eng. J. 2025, 503, 157703.
146. Zhang, W.; Cheng, R. R.; Bi, H. H.; Lu, Y. H.; Ma, L. B.; He, X. J. A review of porous carbons produced by template methods for supercapacitor applications. New. Carbon. Mater. 2021, 36, 69-81.
147. Tian, W. J.; Zhang, H. Y.; Duan, X. G.; Sun, H. Q.; Shao, G. S.; Wang, S. B. Porous carbons: structure-oriented design and versatile applications. Adv. Funct. Mater. 2020, 30, 1909265.
148. Wang, C. S.; Yan, B.; Zheng, J. J.; et al. Recent progress in template-assisted synthesis of porous carbons for supercapacitors. Adv. Powder. Mater. 2022, 1, 100018.
149. Tong, Z.; Yan, X.; Wang, Y.; Li, K.; Ma, W. Lightweight Si3N4@SiO2 ceramic foam for thermal insulation and electromagnetic wave transparency. Nano. Res. 2024, 17, 4298-306.
150. Kamiyama, A.; Kubota, K.; Igarashi, D.; et al. MgO-template synthesis of extremely high capacity hard carbon for Na-ion battery. Angew. Chem. Int. Ed. Engl. 2021, 60, 5114-20.
151. Li, H. X.; Shi, W. J.; Liu, L. Y.; et al. Coupling effect of vacancy defects and multi-adsorption sites in porous carbon cathode for high-performance aqueous zinc-ion hybrid capacitors. Chem. Eng. J. 2024, 487, 150630.
152. Jiang, M. C.; Sun, N.; Li, T. Y.; et al. Revealing the charge storage mechanism in porous carbon to achieve efficient K ion storage. Small 2024, 20, e2401478.
153. Duan, G. G.; Xiao, J. L.; Tian, Z. W.; et al. Nano-CaCO3 templated porous carbon enable high-rate and ultralong cycle performance supercapacitor. J. Energy. Storage. 2024, 78, 109934.
154. Igarashi, D.; Tanaka, Y.; Kubota, K.; et al. New template synthesis of anomalously large capacity hard carbon for Na- and K-ion batteries. Adv. Energy. Mater. 2023, 13, 2302647.
155. Shi, J. S.; Cui, H. M.; Xu, J. G.; Yan, N. G.; Liu, Y. W. Design and fabrication of hierarchically porous carbon frameworks with Fe2O3 cubes as hard template for CO2 adsorption. Chem. Eng. J. 2020, 389, 124459.
156. Doustkhah, E.; Hassandoost, R.; Khataee, A.; Luque, R.; Assadi, M. H. N. Hard-templated metal-organic frameworks for advanced applications. Chem. Soc. Rev. 2021, 50, 2927-53.
157. Wang, S. Y.; Luo, L. Y.; Wu, A. P.; et al. Recent advances in tailoring zeolitic imidazolate frameworks (ZIFs) and their derived materials based on hard template strategy for multifunctional applications. Coord. Chem. Rev. 2024, 498, 215464.
158. Pavlenko, V.; Khosravi, H. S.; Żółtowska, S.; et al. A comprehensive review of template-assisted porous carbons: Modern preparation methods and advanced applications. Mater. Sci. Eng,. R. 2022, 149, 100682.
159. Farghali, M.; Osman, A. I.; Mohamed, I. M. A.; et al. Strategies to save energy in the context of the energy crisis: a review. Environ. Chem. Lett. 2023, 1-37.
160. Wei, X.; Shi, X.; Li, Y.; et al. Analysis of the European energy crisis and its implications for the development of strategic energy storage in China. J. Energy. Storage. 2024, 82, 110522.
161. Zhang, K. K.; Hu, C.; Huang, H. H.; Li, B.; Huang, C. X.; Wang, S. F. Achieving efficient energy utilization by PCM in the food supply chain: encapsulation technologies, current applications, and future prospects. J. Energy. Storage. 2024, 79, 110214.
162. Huang, J.; Wu, B.; Lyu, S.; et al. Improving the thermal energy storage capability of diatom-based biomass/polyethylene glycol composites phase change materials by artificial culture methods. Sol. Energy. Mater. Sol. Cells. 2021, 219, 110797.
163. Tan, Q. L.; Liu, H. F.; Shi, Y.; Zhang, M. Y.; Yu, B. D.; Zhang, Y. Lauric acid/stearic acid/nano-particles composite phase change materials for energy storage in buildings. J. Energy. Storage. 2024, 76, 109664.
164. Zhou, J. X.; Yang, L. J.; Cao, X. Y.; et al. MXene nanosheets coated conjugated microporous polymers hollow microspheres incorporating with phase change material for continuous desalination. J. Colloid. Interface. Sci. 2024, 654, 819-29.
165. Xiao, Y. S.; Li, T.; Yang, Y. J.; et al. Exploring flame-retardant, shape-stabilized multi-functional composite phase change materials. Sol. Energy. Mater. Sol. Cells. 2025, 282, 113369.
166. Huang, J. T.; Zeng, X. F.; Yu, W. T.; Zhang, H. C.; Min, Y. G. Polyimide-based porous carbon/Ni nano-particle composites prepared by phase separation method with broadband absorption characteristics. Diamond. and. Relat. Mater. 2025, 152, 111968.
167. Cao, Y.; Zhao, Z. Z.; Zeng, X. F.; Teng, J. X.; Huang, J. T.; Min, Y. G. High-performance polyimide/polypyrrole-CNTs@PEG composites for integrated thermal management and enhanced electromagnetic wave absorption. Adv. Compos. Hybrid. Mater. 2025, 8, 1202.
168. Liu, M.; Qian, R. D.; Yang, Y.; Lu, X. T.; Huang, L.; Zou, D. Q. Modification of phase change materials for electric-thermal, photo-thermal, and magnetic-thermal conversions: a comprehensive review. Adv. Funct. Mater. 2024, 34, 2400038.
169. Wang, C. J.; Liang, W. D.; Yang, Y. Y.; et al. Biomass carbon aerogels based shape-stable phase change composites with high light-to-thermal efficiency for energy storage. Renew. Energy. 2020, 153, 182-92.
170. Cao, Y.; Li, W.; Huang, D.; et al. One-step construction of novel phase change composites supported by a biomass/MXene gel network for efficient thermal energy storage. Sol. Energy. Mater. Sol. Cells. 2022, 241, 111729.
171. Huang, J. T.; Su, J. T.; Weng, M. M.; et al. An innovative phase change composite with high thermal conductivity and sensitive light response rate for thermal energy storage. Solar. Energy. Materials. and. Solar. Cells. 2022, 245, 111872.
172. Huang, J. T.; Su, J. T.; Xu, W. H.; et al. High enthalpy efficiency lignin-polyimide porous hybrid aerogel composite phase change material with flame retardancy for superior solar-to-thermal energy conversion and storage. Sol. Energy. Mater. Sol. Cells. 2022, 248, 112036.
173. Shen, R. B.; Lian, P.; Cao, Y.; Chen, Y.; Zhang, L.; Sheng, X. X. All lignin-based sponge encapsulated phase change composites with enhanced solar-thermal conversion capability and satisfactory shape stability for thermal energy storage. J. Energy. Storage. 2022, 54, 105338.
174. Liu, X. L.; Ni, R. Z.; Tian, Y.; Yao, H. C.; Xu, Q.; Xuan, Y. M. Environment-friendly efficient thermal energy storage paradigm based on sugarcane-derived eco-ceramics phase change composites: from material to device. Renew. Energy. 2023, 217, 119155.
175. Hu, Y.; Zhang, M. U.; Quan, B. Q.; et al. Polyethylene glycol infiltrated biomass-derived porous carbon phase change composites for efficient thermal energy storage. Adv. Compos. Hybrid. Mater. 2024, 7, 880.
176. Huo, Y. J.; Yan, T.; Pan, W. G. Efficient solar thermal storage of foamy bamboo charcoal-based composite phase change materials. Sol. Energy. 2024, 268, 112269.
177. Tian, W. S.; Xiao, Y.; Qin, G. Z.; Zheng, X. Anisotropic and shape-stable sugarcane-based phase change composites in the application of solar thermal energy storage. Energy 2024, 308, 132942.
178. Ye, X. Y.; Yang, D. J.; Yu, L. L.; Jiang, P. W.; Liu, W. F.; Lou, H. M. Phase change material composites based on 3D lignin-derived porous carbon prepared by in-situ activation for efficient solar-driven energy conversion and storage. J. Colloid. Interface. Sci. 2025, 678, 704-19.
179. Rathore, P. K. S.; Gupta, N. K.; Yadav, D.; Shukla, S. K.; Kaul, S. Thermal performance of the building envelope integrated with phase change material for thermal energy storage: an updated review. Sustainable. Cities. and. Soc. 2022, 79, 103690.
180. Reddy, V. J.; Ghazali, M. F.; Kumarasamy, S. Advancements in phase change materials for energy-efficient building construction: a comprehensive review. J. Energy. Storage. 2024, 81, 110494.
181. Qi, C. S.; Zhang, F.; Mu, J.; Zhang, Y.; Yu, Z. M. Enhanced mechanical and thermal properties of hollow wood composites filled with phase-change material. J. Cleaner. Prod. 2020, 256, 120373.
182. Xu, J. N.; Sun, J. M.; Zhao, J. Q.; et al. Eco-friendly wood plastic composites with biomass-activated carbon-based form-stable phase change material for building energy conversion. Ind. Crops. Prod. 2023, 197, 116573.
183. Yue, X. F.; Zhang, R.; Jin, X. B.; Zhang, X. F.; Bao, G. G.; Qin, D. C. Bamboo-derived phase change material with hierarchical structure for thermal energy storage of building. J. Energy. Storage. 2023, 62, 106911.
184. Chen, M. Y.; Yu, Y.; Ouyang, D. X.; et al. Research progress of enhancing battery safety with phase change materials. Renew. Sust. Energy. Rev. 2024, 189, 113921.
185. Jiang, H.; Li, J. D.; Xie, Y. H.; et al. Design of efficient microstructured path by magnetic orientation boron nitride nanosheets/MnFe2O4 enabling waterborne polyurethane with high thermal conductivity and flame retardancy. J. Mater. Sci. Technol. 2025, 209, 207-18.
186. Wu, B. Y.; Lyu, S.; Han, H.; et al. Biomass-based shape-stabilized phase change materials from artificially cultured ship-shaped diatom frustules with high enthalpy for thermal energy storage. Compos. Part. B:. Eng. 2021, 205, 108500.
187. Muthya Goud, V.; Raval, F.; Ruben Sudhakar, D. A sustainable biochar-based shape stable composite phase change material for thermal management of a lithium-ion battery system and hybrid neural network modeling for heat flow prediction. J. Energy. Storage. 2022, 56, 106163.
188. Xiong, T.; Ok, Y. S.; Dissanayake, P. D.; et al. Preparation and thermal conductivity enhancement of a paraffin wax-based composite phase change material doped with garlic stem biochar microparticles. Sci. Total. Environ. 2022, 827, 154341.
189. Huang, D. Y.; Wang, Z. B.; Sheng, X. X.; Chen, Y. Bio-based MXene hybrid aerogel/paraffin composite phase change materials with superior photo and electrical responses toward solar thermal energy storage. Sol. Energy. Mater. Sol. Cells. 2023, 251, 112124.
190. Lee, W.; Lee, J.; Yang, W.; Kim, J. Fabrication of biobased advanced phase change material and multifunctional composites for efficient thermal management. ACS. Sust. Chem. Eng. 2023, 11, 1178-89.
191. Cao, Y.; Zeng, Z. H.; Huang, D. Y.; Chen, Y.; Zhang, L.; Sheng, X. X. Multifunctional phase change composites based on biomass/MXene-derived hybrid scaffolds for excellent electromagnetic interference shielding and superior solar/electro-thermal energy storage. Nano. Res. 2022, 15, 8524-35.
192. Chen, Y.; Meng, Y.; Zhang, J.; et al. Leakage Proof, flame-retardant, and electromagnetic shield wood morphology genetic composite phase change materials for solar thermal energy harvesting. Nanomicro. Lett. 2024, 16, 196.
193. Hou, X.; Feng, X. R.; Jiang, K.; Zheng, Y. C.; Liu, J. T.; Wang, M. Recent progress in smart electromagnetic interference shielding materials. J. Mater. Sci. Technol. 2024, 186, 256-71.
194. He, H. F.; Wang, Y. B.; Zhao, Z. L.; Wang, Q. Q.; Wei, Q. F.; Cai, Y. B. Dual-encapsulated multifunctional phase change composites based on biological porous carbon for efficient energy storage and conversion, thermal management, and electromagnetic interference shielding. J. Energy. Storage. 2022, 55, 105358.
195. Verma, R.; Thakur, P.; Chauhan, A.; Jasrotia, R.; Thakur, A. A review on MXene and its’ composites for electromagnetic interference (EMI) shielding applications. Carbon 2023, 208, 170-90.
Cite This Article

How to Cite
Download Citation
Export Citation File:
Type of Import
Tips on Downloading Citation
Citation Manager File Format
Type of Import
Direct Import: When the Direct Import option is selected (the default state), a dialogue box will give you the option to Save or Open the downloaded citation data. Choosing Open will either launch your citation manager or give you a choice of applications with which to use the metadata. The Save option saves the file locally for later use.
Indirect Import: When the Indirect Import option is selected, the metadata is displayed and may be copied and pasted as needed.
About This Article
Copyright
Data & Comments
Data
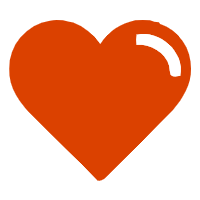
Comments
Comments must be written in English. Spam, offensive content, impersonation, and private information will not be permitted. If any comment is reported and identified as inappropriate content by OAE staff, the comment will be removed without notice. If you have any queries or need any help, please contact us at [email protected].