Surface engineering of Li3V2(PO4)3-based cathode materials with enhanced performance for lithium-ion batteries working in a wide temperature range
Abstract
Operating at extreme temperatures is the biggest challenge for lithium-ion batteries (LIBs) in practical applications, as both the capacity and cycling stability of LIBs are largely decreased due to the sluggish reaction kinetics of the cathodes. Therefore, developing suitable cathode materials is the key point to tackling this challenge. Lithium vanadium phosphate [Li3V2(PO4)3, LVP] is a promising cathode with good features of a high working voltage, high intrinsic ionic diffusion coefficiency, and stable olivine structure in a wide temperature range, although it is perplexed by the low electronic conductivity. To tackle this issue, a series of nitrogen-doped carbon network (NC) coated LVP composites were synthesized using a hydrothermal-assisted sol-gel method. Among them, the
Keywords
INTRODUCTION
Lithium-ion batteries (LIBs) have been widely applied in portable electronics and electric vehicles due to their high energy densities and long lifespan[1,2]. They also play significant roles in some critical application scenarios, such as space explorations, drones, and military equipment that are required to operate in a wide temperature range[3]. However, operating at extreme temperatures (-20 and 60 °C) is one of the biggest challenges for LIBs due to the relatively poor electrochemical performances of electrode materials. The current commercial cathodes are restricted by either the low ionic diffusion coefficiency (e.g., LiFePO4 and LiMnO2) at low temperatures or the unstable structures (e.g., LiCoO2, LiNixCoyMnzO2, and LiNixCoyAlzO2,
Operating at extreme temperatures is regarded as a big challenge to most of the reported cathode materials of LIBs due to their common drawbacks of sluggish ion diffusion kinetics, large voltage hysteresis and high polarization at sub-zero temperatures, and structural instability or oxygen-release at high temperatures. Recent progress in promising cathode materials has witnessed remarkable achievements of cathode materials to operate in a wide temperature range, including LiNiPO4, LiMnPO4, and Li3V2(PO4)3 (LVP)[8-11]. Among them, the modified LVP, with the advantages of high ionic mobility, high theoretical capacity, high average working voltage (4.0 V), and good cycle stability, is regarded as one of the most promising cathode materials for LIBs to work in the wide-temperature-range environment. The specific capacity of LVP is
Unfortunately, the poor intrinsic electronic conductivity (2.4 × 10-7 S cm-1 at room temperature) and complicated lithium deintercalation process of LVP showed unsatisfied electrochemical performance, which probably results from the formation of different distorted phases during the charging process, the low intrinsic conductive V2(PO4)3 phase, and the wide intrinsic bandgap. To tackle those issues, many strategies have been applied, including carbon coating, surface modification, ion doping, reducing particle sizes, and electrolyte optimization. Among them, a variety of carbonous materials have been utilized to encapsulate LVP materials or form a surface coating layer on LVP particles. Those carbon-based structures or carbonous materials include carbon shells[18,19], carbon networks[20], carbon nanofibers[21], graphene[22], and other highly conductive carbon-based materials[23]. Surface modification has been widely used to improve electron transfer and enhance the cycling stability of LVP[24,25]. In addition, the heteroatomic doping can affect the electrochemical potentials and tune the intrinsic electronic conductivity of LVP by partial substitutions at Li or vanadium (V) sites[26-28], phosphate group (PO4)[29], or even anion and cation co-doping[30], leading to the reduction of electronic bandgaps, the formation of vacancy, and the decrease of energy barriers.
Herein, to enhance the electronic conductivity and structural stability of LVP cathode materials working in a wide voltage range (3.0-4.8 V) and a wide temperature range (-20 to 60 °C), a series of nitrogen-doped carbon network (NC) encapsulated LVP composites (LVP@NC-x, x = 0.5, 0.8, 1, 2, and 3) were synthesized, in which the LVP@NC-0.8 composite exhibited the highest capacity and best cycling stability in a wide temperature range (-20~60 °C) with a high charging cutoff voltage of 4.8 V. Furthermore, the detailed structure evolution behaviors of the LVP@NC-0.8 electrode were investigated via in-situ X-ray diffraction (XRD). Density functional theory (DFT) calculation results also indicated the advantage of the nitrogen-doped carbon coating strategy. Moreover, full cells with LVP@NC-0.8 cathodes and graphite anodes displayed a high working voltage of 3.5 V, good cycling stability, and high-rate capability, illustrating excellent electrochemical properties. This work verifies a new possibility of developing LVP-based cathode materials for all-climate and high-voltage applications.
RESULTS AND DISCUSSION
Materials synthesis and characterization
A series of carbon-coated LVP composites (LVP@NC-x, x = 0.5, 0.8, 1, 2, and 3) were prepared by using the hydrothermal-assisted sol-gel method, as shown in Figure 1A. With the aid of trimethyloctadecylammonium bromide (STAB), LVP nanoparticles are completely wrapped by thin nitrogen-doped carbon layers, forming interconnected secondary microparticles. All the XRD profiles of LVP@NC-x composites are well corresponded with the monoclinic structure phase (JCPDS No. 01-072-7074) with a space group of P21/n and a typical lattice constant (a = 8.606 Å, b = 8.592 Å, c = 12.037 Å, and
Figure 1. The basic properties of LVP@NC-x (x = 0.5, 0.8, 1, 2, and 3) composites: (A) Schematic illustration of the synthesis steps. (B) XRD patterns. (C) Rietveld refinement of LVP@NC-0.8 composite. SEM images of (D) precursor and (E and F) LVP@NC-0.8 composite in the low and high magnifications. (G and H) TEM images, (I) the corresponding statistical particle size distribution, where
The scanning electron microscope (SEM) images have presented slight morphology differences among the precursors with different STAB content, as shown in Figure 1D and Supplementary Figure 3A and B. Apparently, with the increase of STAB content, the precursors gradually change from small nanoparticles to layered thin nanosheets. After annealing, the final morphologies of LVP@NC-x composites are shown in Figure 1E, Supplementary Figure 3C and D. The diameter of the LVP@NC-0.8 composite after calcination reduced from ~20 to ~13 μm, as shown in Figure 1D and F. Supplementary Figure 3D shows a significant structure collapse of LVP@NC-1 after annealing. In contrast, with reduced STAB content, larger clusters with secondary microparticles were obtained, as shown in Supplementary Figure 3C. The amounts of C and N of LVP@NC-x (x = 0.5, 0.8, and 1) composites were below 5% measured by the elemental analysis
To further characterize the property of LVP@NC-x (x = 0.5, 0.8, 1) samples, Fourier transform infrared spectra (FTIR) were applied to measure the surface functional groups. As shown in Figure 2A, there is no obvious difference in FTIR spectra among LVP@NC-0.5, LVP@NC-0.8, and LVP@NC-1 samples. The characteristic peaks between 1,000-750 cm-1 are associated with the vibrations of the VO6 group, and the characteristic peaks between 1,600-1,300, 1,350-1,000, and 750-500 cm-1 are ascribed to internal vibrations, intramolecular stretching, intramolecular bending of the (PO4)3- anion, respectively[29,31-33]. The Raman spectra of LVP@NC-x (x = 0.5, 0.8, 1) samples are shown in Figure 2B. There are two obvious carbon characteristic bands at the vicinity of 1,350 and 1,602 cm-1, which correspond to the D band originating from disordered or amorphous carbon and the G band indexing from ordered or graphitized carbon, respectively. The ID/IG ratio of LVP@NC-0.8 (0.86) is lower than that of LVP@NC-0.5 (0.88) and
Figure 2. (A) FTIR spectra and (B) Raman spectra of LVP@NC-x (x = 0.5, 0.8, 1) composites. (C) Nitrogen adsorption-desorption isotherms of LVP@NC-0.8 composite, the inset is the corresponding plot of pore size distribution. (D) XPS survey spectra and high-resolution XPS spectra of (E) V 2p, (F) P 2p, (G) O 1s, (H) C 1s, and (I) N 1s of LVP@NC-0.8 composite.
The surface area and pore size distribution of all samples were examined by the Nitrogen adsorption-desorption isotherms in Figure 2C and Supplementary Figure 4A and B. The isotherm curves of the
X-ray photoelectron spectroscopy (XPS) was employed to explore the chemical states of the LVP@NC-0.8 composite. The characteristic peaks of the full spectrum of XPS in Figure 2D are located at 629.6 eV (V 2s), 530.5 eV (O 1s), 517.3 eV (V 2P), 400.6 eV (N 1s), 284.4 eV (C 1s), 190.8 eV (P 2s), and 133.0 eV (P 2P), respectively. In addition, as shown in the high-resolution spectrum of Figure 2E, two peaks are attributed to the typical spin-orbit coupling of V 2p3/2 and V 2p1/2. After deconvolution, the peaks at 524.2 and 516.8 eV are from V4+, and the peaks at 523.0 and 515.6 eV originate from V3+, which proves that V has multiple oxidation states in the LVP@NC-0.8 composite[29]. As shown in Figure 2F, the peaks at 134.4, 133.4, and 132.7 eV in the XPS spectrum of P2p originate from P-O-P bond, VOPO4, and PO43- anions, respectively, indicating that C and N elements in LVP@NC-0.8 composite only exist on the surface of LVP nanoparticles, and no related chemical bond is forming within LVP crystal[35]. In the high-resolution spectrum of O 1s [Figure 2G], two fitting peaks at 533.5 and 532.0 eV correspond to C-O and C=O, respectively, while the relatively strong fitting peaks at 530.6 eV are related to V-O and P-O[35]. In addition, the peak in the C1s XPS spectrum [Figure 2H] is divided into 288.1, 286.0, and 284.4 eV, which originate from C=O, C=N, and C-C, respectively. The strong peak intensity of the C-C bond mainly reflects the graphitized carbon in the LVP@NC-0.8 composite. The C=N bond indicates that nitrogen has been successfully doped into the carbon network[36]. The N 1s spectrum [Figure 2I] can be deconvoluted into three peaks at 401.5, 400.5, and 398.7 eV, corresponding to N-O, C=N, and C-N bonds, respectively, which is in good agreement with the previous report[37].
Electrochemical performance evaluation
To optimize the carbon content, half cells were fabricated using different LVP@NC-x (x = 0.5, 0.8, 1, 2, 3) composites as cathodes for electrochemical performance evaluation with a voltage window of 3-4.8 V [Figure 3A]. With the increase of carbon content in the cathodes, the LVP@NC-0.8 cathode shows the highest discharge capacity (174 mAh g-1) among all samples. The electrochemical behaviors were further examined by the cyclic voltammetry (CV) measurements. In the first cathodic scan [Figure 3B and Supplementary Figure 5], three narrow oxidation peaks and one relatively wide oxidation peak of the
Figure 3. Electrochemical characterizations at both (A-G) the room temperature and (H and I) the extreme temperature (-20~60 °C) in 3.0 to 4.8 V. (A) Reversible capacity comparison of LVP@NC-x (x = 0.5, 0.8, 1, 2, 3). (B) The first three CV curves and (C) charge/discharge profiles of the LVP@NC-0.8 cathode in different cycles at 0.5 C. (D) Rate performances at the different current densities from
Herein, the y (0 < y < 1) value is confined by the remarkable fact that the last Li ion is not completely extracted during the charging process, probably hindered by the strong interactive force of the phosphate framework and lattice parameter shrinkage; thereby, a large overpotential and a rapidly raised voltage curve is obtained.
The performances of LVP@NC-x (x = 0.5, 0.8, 1, 2, and 3) composites were further evaluated at different current densities and temperatures. The reversible capacities of LVP@NC-x (x = 0.5, 0.8, 1, 2, and 3) composites are 168, 174, 138, 122, and 116 mAh g-1 at 0.5 C, respectively [Figure 3A]. Figure 3C shows the galvanostatic charge/discharge behaviors of the LVP@NC-0.8 electrode at different cycles. The related Coulombic efficiency gradually increased from 88.9% at the 5th cycle to 98.4% at the 50th cycle and stabilized at ~99% in the subsequent cycle. Figure 3D displays the rate performances of LVP@NC-0.5, LVP@NC-0.8, and LVP@NC-1 electrodes at different current densities. The LVP@NC-0.8 cathode displays reversible capacities of 174.0, 165.9, 157.9, 148.5, 142.2, and 122.5 mAh g-1 at 0.5, 1, 2, 5, 10, and 20 C, respectively. Moreover, when the current rate is reduced to 0.5 C, a high reversible capacity of 164 mAh g-1 is recovered, demonstrating a superior tolerance to high current densities, which is much better than that of LVP@NC-0.5 and LVP@NC-1 cathodes. As shown in Figure 3E, the LVP@NC-x (x = 0.5, 0.8, and 1) cathodes maintained the discharge capacities of 131, 143, and 118 mAh g-1 after 100 cycles with the capacity retentions of 79.0%, 85.1%, and 83.1%, respectively. It is worth mentioning that LVP@NC-0.5 and LVP@NC-1 cathodes show major capacity attenuations, while LVP@NC-0.8 has achieved superior cycling stability, which is mainly attributed to its appropriate NC and well-encapsulation effect that effectively alleviates the side reaction between an electrode and electrolyte[37,40]. To further measure the long-term cycling stability, LVP@NC-x electrodes were tested at a high rate of 10 C [Figure 3F]. Among them, the LVP@NC-0.8 electrode retained a remarkable rechargeable capacity of 129.2 mAh g-1 after 1000 cycles with an astonishing capacity retention of ~87%. Figure 3G shows the Nyquist plots of LVP@NC-x (x = 0.5, 0.8, and 1) composites with an equivalent circuit simulating electrochemical impedance spectroscopy (EIS) data. All the EIS patterns consist of a semicircle in the high-medium frequency region and an oblique line in the low-frequency region. Among them, the semicircle is attributed to the resistances of the solid electrolyte interface (Rs) formed on the electrode, the charge transfer resistance (Rct), and the constant phase element (CPE), while an oblique line in the low-frequency region is related to the Warburg impedance (Wo), arising from the diffusion resistance of Li ions within the bulk. The fitted values of LVP@NC-x (x = 0.5, 0.8, 1) are all listed in Supplementary Table 3. Rs values of all composites were similar and relatively small compared to Rct values. Moreover, the results show that LVP@NC-0.8 has the minimum Rct value, which indicates that an appropriate amount of NC can effectively improve the electronic conductivity, facilitating the deintercalation processes of Li ions, giving a positive effect on the electrochemical performance[41].
To investigate the diffusion behavior of Li ions in LVP@NC-0.8, galvanostatic intermittent titration technique (GITT) measurements were performed to determine the diffusion coefficient of Li ions (DLi+) in the two-phase transition and the solid-solution reaction region during the charge/discharge cycle[42]. The purple curve in Supplementary Figure 6 shows the GITT curve during the 11th cycle as a function of time in 3.0-4.8 V, and the diffusion coefficient of Li ions calculated by the GITT curve is shown as the blue dots. The DLi+ values measured during the charging process and the insertion of the third Li ion process that undergo a two-phase transition are apparent values, and the DLi+ values measured during the insertion of the first two Li ions that undergo solid-solution reaction are true values[36]. The DLi+ values are in the range of 1.69 × 10-15-1.97 × 10-12 cm2 s-1 during the charge processes. While the DLi+ values during the solid-solution reaction (4.29-3.76 V) are in the range of 2.97 × 10-13-1.25 × 10-12 cm2 s-1, and the DLi+ values range from
To explore the applicability of LVP@NC-0.8 cathodes under extreme temperature conditions, it was further tested in a wide voltage range of 3.0-4.8 V and a wide temperature range of -20~60 °C. As shown in Figure 3H, with the decrease in the operating temperatures, the electrode shows a more solid-solution electrochemical behavior until the complete solid-solution reaction occurs at -10 °C. With the increase in the operating temperatures, the deintercalation of the last Li ion and intercalation of all Li ions are completely transformed into a solid-solution reaction, accompanying part of the two-phase reactions. The electrode delivered discharged capacities of 119, 124, 131, 174, 162, and 156 mAh g-1 at -20, -10, 0, 25, 40, and 60 °C at 0.5 C, respectively. The corresponding dQ/dV profiles are shown in Supplementary Figure 7. With the decrease of the temperature, the potential differences of the peaks gradually increase. Meanwhile, the intensities of redox peaks decrease. The cycling performance of the LVP@NC-0.8 electrode at different temperatures was displayed in Figure 3I. Apparently, it showed improved cycle stability at 25, 40, and 60 °C than that at -20, -10, and 0 °C. It is worth noting that the LVP@NC-0.8 electrode can still be stably operated at 60 °C. After the Li ions are released from the lattices, both the slow ionic diffusion at low temperatures and the severe structure distortion at high temperatures make the re-insertion of Li ions more difficult, resulting in a high electrode pulverization and voltage hysteresis. As shown in Supplementary Table 4, the performance comparison of the cathode materials demonstrates the as-prepared LVP@NC-0.8 cathode shows superiority when applied at extreme temperatures[43-46].
Reaction mechanism investigation
To analyze the structural evolution and capacity fading mechanisms of the LVP@NC-0.8 electrode at different temperatures, the in-situ XRD technique was conducted. As shown in Figure 4A, during the early charging stage, all major peaks shift discontinuously into the delithiated phase, indicating the formation of new phases by a two-phase reaction mechanism. The diffraction peaks then discontinuously shift to the higher angle, and the intensities of the peaks are subsequently weakened. During the formation of the
Figure 4. Phase evolutions of LVP@NC-0.8 electrode measured at -12, 25, and 60 °C in 3-4.8 V. (A) In-situ XRD waterfall plots in the initial cycle. (B) In-situ XRD mountain plots and the corresponding charge/discharge curves in the first three cycles.
Moreover, the reason for the capacity loss at extreme temperatures (-12 and 60 °C) was further examined via the in-situ XRD technique. The peak intensity of new phases (blue and yellow dotted circles) is relatively weaker at -12 and 60 °C than that at 25 °C (green dotted circle). In addition, the diffraction peaks of the
To further analyze the cyclic stability of the LVP@NC-0.8 electrode, the structure evolutions of the first three charge/discharge processes at -12, 25, and 60 °C were investigated. As shown in Figure 4B, the in-situ XRD mountain plots at different temperatures have demonstrated that the diffraction peaks in the last two cycles of the typical crystal planes show a similar peak variation trend as that in the first cycle at different temperatures. In contrast, taking the (020) plane as an example, the peak intensity decreases successively from the first cycle to the third cycle at 25 and 60 °C, while the peak intensity remains unchanged in the first three cycles at -12 °C. The electrode polarizations are aggravated at 25 and 60 °C. The sluggish movement of Li ions inhibits the serious structure distortion at -12 °C, which is conducive to the stability of the subsequent cycle. The results show that the LVP@NC-0.8 electrode has good cycling stability in the wide temperature range even at a high charging cutoff voltage of 4.8 V. Therefore, the development of LVP-based cathode materials is a promising choice for LIBs applied to operate in extreme conditions and at high operating voltage for high energy density purposes.
Theoretical calculations
To study the effect of carbon-coating on the modified LVP, the molecules of graphene and LVP crystal structure were built to represent the carbon layer and LVP crystal. The spin-polarized total densities of states (TDOS) were further calculated [Figure 5A and B]. As shown in Figure 5B, compared with the bare LVP [Figure 5A], the bandgap energy is significantly reduced from the initial 1.959 eV (LVP) to 0.20 eV (LVP@NC), demonstrating the electrons in the carbon-coated LVP are more easily excited from the valence band to the conduction band than that in bare LVP. The result verifies that the carbon-coating strategy effectively improves the intrinsic conductivity of LVP. Figure 5C-H and Supplementary Figure 8 further compare the possible migration paths of Li ions and the required energy during the migration process in bare LVP and LVP@NC, displaying the typical diffusion paths (horizontal-vertical in the top view) in the three scenarios [Figure 5C-E and Supplementary Figure 8]. Compared to the energy barriers inside bare LVP (3.74 eV), inside LVP subject (2.73 eV), and between LVP and NC (0.89 eV) [Figure 5F and G], the energy barrier of LVP@NC (0.47 eV considering Li ions are moving on the surface of NC) is the lowest [Figure 5H]. The simulated results illustrate that the Li ions are firstly transferring along the carbon shell and then moving between the interfaces, finally transporting into the LVP lattice, further displaying that the migration of Li ions in LVP@NC is considerably simpler than that in bare LVP.
Figure 5. TDOS of (A) LVP and (B) LVP@NC. (C-E) Schematics of the migration pathways and (F-H) the corresponding diffusion energy barriers of lithium-ion (C and F) inside LVP subject, (D and G) between LVP and NC, and (E and H) outside of NC of LVP@NC. In addition, the yellow, brown, purple, red, sky-blue, and gray spheres in cells represent Li, V, P, O, N, and C atoms, respectively.
Full cell performance evaluation
To verify the practical applicability of the LVP@NC-0.8 electrode, the full cell of LVP@NC-0.8||graphite was assembled. The schematic configuration of the full cell is shown in Figure 6A. The electrochemical behaviors of the full cell cycled at 0.5 C are presented in Figure 6B. The Coulombic efficiencies gradually increased from ~88% in the 2nd cycle to ~96% in the 10th cycle and finally stabilized at ~96%. The cycling stability is shown in Figure 6C, displaying a reversible capacity of 118.5 mAh g-1 with an astonishing capacity retention of ~70.1% for 100 cycles at 0.5 C. The full cells have also presented superior rate capability with reversible capacities of 169.8, 144.2, 130.3, 118.3, 100.6, and 75.5 mAh g-1 at 0.5, 1, 2, 5, 10, and 20 C, respectively [Figure 6D]. When the current rate returns to 0.5 C, it still exhibits a reversible capacity of ~139 mAh g-1, proving that the LVP@NC-0.8 can be applied in full cells with high capacity, good rate capability, and superior cycling stability.
CONCLUSIONS
In summary, with the assistance of cationic surfactant (STAB), a series of LVP embedded in NC materials were successfully achieved through a hydrothermal-assisted sol-gel method. Among them, the
DECLARATIONS
Acknowledgments
Chen S would like to acknowledge the Research Center of Shanghai University for offering access to material characterizations, and all the computations were performed on the high-performance computing platform of Shanghai University with official permissions.
Authors’ contributions
Methodology, experiment, data analysis, interpretation, initial draft writing, and manuscript revision:
Data acquisition, visualization, and investigation: Wang L, Peng Q
Manuscript revision: Dong H, Yang C, Xiao Y, Wang Y (Yong Wang)
Supervision, manuscript revision, and funding acquisition: Chou S, Sun B, Chen S
Availability of data and materials
The data that support the findings of this study are available from the corresponding author upon reasonable request.
Financial support and sponsorship
This work was financially supported by the National Natural Science Foundation of China (21975154, 22179078) and Zhejiang Provincial Natural Science Foundation of China (LY24E020002).
Conflicts of interest
All authors declared that there are no conflicts of interest.
Ethical approval and consent to participate
Not applicable.
Consent for publication
Not applicable.
Copyright
© The Author(s) 2024.
Supplementary Materials
REFERENCES
1. Feng J, Shi C, Dong H, et al. Design of ZnSe-CoSe heterostructure decorated in hollow N-doped carbon nanocage with generous adsorption and catalysis sites for the reversibly fast kinetics of polysulfide conversion. J Energy Chem 2023;86:135-45.
2. Cui X, Chen J, Sun Z, et al. A general route for encapsulating monodispersed transition metal phosphides into carbon multi-chambers toward high-efficient lithium-ion storage with underlying mechanism exploration. Adv Funct Mater 2023;33:2212100.
3. Liu Y, Russo PA, Montoro LA, Pinna N. Recent developments in Nb-based oxides with crystallographic shear structures as anode materials for high-rate lithium-ion energy storage. Battery Energy 2023;2:20220037.
4. Chen Z, Dai C, Wu G, et al. High performance Li3V2(PO4)3/C composite cathode material for lithium ion batteries studied in pilot scale test. Electrochim Acta 2010;55:8595-9.
5. Liu Y, Yang B, Dong X, Wang Y, Xia Y. A simple prelithiation strategy to build a high-rate and long-life lithium-ion battery with improved low-temperature performance. Angew Chem Int Ed 2017;56:16606-10.
6. Luo Y, Xu X, Zhang Y, et al. Hierarchical carbon decorated Li3V2(PO4)3 as a bicontinuous cathode with high-rate capability and broad temperature adaptability. Adv Energy Mater 2014;4:1400107.
7. Ni Q, Zheng L, Bai Y, et al. An extremely fast charging Li3V2(PO4)3 cathode at a 4.8 V cutoff voltage for Li-ion batteries. ACS Energy Lett 2020;5:1763-70.
8. Tong J, Su A, Ma T, et al. Boosting low temperature performance of lithium ion batteries at -40 °С using a binary surface coated
9. Cai G, Yang Y, Guo R, et al. Synthesis and low temperature electrochemical properties of CeO2 and C co-modified Li3V2(PO4)3 cathode materials for lithium-ion batteries. Electrochim Acta 2015;174:1131-40.
10. Qin R, Wei Y, Zhai T, Li H. LISICON structured Li3V2(PO4)3 with high rate and ultralong life for low-temperature lithium-ion batteries. J Mater Chem A 2018;6:9737-46.
11. Rashad M, Zhang H, Li X, Zhang H. Fast kinetics of Mg2+/Li+ hybrid ions in a polyanion Li3V2(PO4)3 cathode in a wide temperature range. J Mater Chem A 2019;7:9968-76.
12. Tan HT, Rui X, Sun W, Yan Q, Lim TM. Vanadium-based nanostructure materials for secondary lithium battery applications. Nanoscale 2015;7:14595-607.
13. Liang M, Li L, Cui X, et al. Ru- and Cl-Codoped Li3V2(PO4)3 with enhanced performance for lithium-ion batteries in a wide temperature range. Small 2022;18:e2202151.
14. Zhuang B, Wu Z, Chu W, et al. High-performance lithium-ion supercapatteries constructed using Li3V2(PO4)3/C mesoporous nanosheets. ChemistrySelect 2019;4:9822-8.
15. Wu J, Xu M, Tang C, Li G, He H, Li CM. F-doping effects on carbon-coated Li3V2(PO4)3 as a cathode for high performance lithium rechargeable batteries: combined experimental and DFT studies. Phys Chem Chem Phys 2018;20:15192-202.
16. Liu X, Feng X, Xu X, Wang F, Wang Y. Sol-assisted spray-drying synthesis of porous Li3V2(PO4)3/C microspheres as high-activity cathode materials for lithium-ion batteries. J Sol-Gel Sci Technol 2018;86:343-50.
17. Xu J, Chou SL, Zhou C, Gu QF, Liu HK, Dou SX. Three-dimensional-network Li3V2(PO4)3/C composite as high rate lithium ion battery cathode material and its compatibility with ionic liquid electrolytes. J Power Sources 2014;246:124-31.
18. Chen T, Zhou J, Fang G, et al. Rational design and synthesis of Li3V2(PO4)3/C nanocomposites as high-performance cathodes for lithium-ion batteries. ACS Sustain Chem Eng 2018;6:7250-6.
19. Liao Y, Li C, Lou X, et al. Carbon-coated Li3V2(PO4)3 derived from metal-organic framework as cathode for lithium-ion batteries with high stability. Electrochim Acta 2018;271:608-16.
20. Huang H, Yin SC, Kerr T, Taylor N, Nazar LF. Nanostructured composites: a high capacity, fast rate Li3V2(PO4)3/carbon cathode for rechargeable lithium batteries. Adv Mater 2002;14:1525-8.
21. Shin J, Yang J, Sergey C, Song MS, Kang YM. Carbon nanofibers heavy laden with Li3V2(PO4)3 particles featuring superb kinetics for high-power lithium ion battery. Adv Sci 2017;4:1700128.
22. Li Y, Xie D, Zhang Yd, et al. Synthesis and electrochemical performance of xLiV3O8·yLi3V2(PO4)3/rGO composite cathode materials for lithium ion batteries. J Mater Chem A 2015;3:14731-40.
23. Kim J, Yoo Jk, Jung YS, Kang K. Li3V2(PO4)3/conducting polymer as a high power 4 V-class lithium battery electrode. Adv Energy Mater 2013;3:1004-7.
24. Oh RG, Hong JE, Yang WG, Ryu KS. Effects of Al2O3 and AlF3 coating on the electrochemical performance of Li3V2(PO4)3/C cathode material in lithium ion batteries. Solid State Ion 2015;283:131-6.
25. Wang B, Sun D, Guo R, et al. Amorphous MnO2-modified Li3V2(PO4)3/C as high-performance cathode for LIBs: the double effects of surface coating. J Mater Sci 2018;53:2709-24.
26. Fu Q, Liu S, Sarapulova A, et al. Electrochemical and structural investigation of calcium substituted monoclinic Li3V2(PO4)3 anode materials for Li-ion batteries. Adv Energy Mater 2019;9:1901864.
27. Fan Q, Zhang Y, Xu Q, et al. Coral-shaped porous LiFePO4/graphene hybrids for high rate and all-climate battery applications. Energy Stor Mater 2019;21:457-63.
28. Li H, Peng L, Wu D, Wu J, Zhu Y, Hu X. Ultrahigh-capacity and fire-resistant LiFePO4-based composite cathodes for advanced lithium-ion batteries. Adv Energy Mater 2019;9:1802930.
29. Zeng XX, Chen H, Guo G, et al. Raising the capacity of lithium vanadium phosphate via anion and cation co-substitution. Sci China Chem 2020;63:203-7.
30. Chen Y, Zhao Y, An X, Liu J, Dong Y, Chen L. Preparation and electrochemical performance studies on Cr-doped Li3V2(PO4)3 as cathode materials for lithium-ion batteries. Electrochim Acta 2009;54:5844-50.
31. Chen Y, Zhang D, Bian X, et al. Characterizations of the electrode/electrolyte interfacial properties of carbon coated Li3V2(PO4)3 cathode material in LiPF6 based electrolyte. Electrochim Acta 2012;79:95-101.
32. Terny S, Vega-Castillo J, de la Rubia M, de Frutos J, Frechero M. Sol-gel synthesis and electrical characterization of doped-carbon decorated mixed conductor ceramics. Mater Sci Eng B 2019;241:66-74.
33. Yan J, Yuan W, Tang ZY, Xie H, Mao WF, Ma L. Synthesis and electrochemical performance of Li3V2(PO4)3-xClx/C cathode materials for lithium-ion batteries. J Power Sources 2012;209:251-6.
34. Qiao YQ, Tu JP, Wang XL, Gu CD. The low and high temperature electrochemical performances of Li3V2(PO4)3/C cathode material for Li-ion batteries. J Power Sources 2012;199:287-92.
35. Wang C, Guo Z, Shen W, Xu Q, Liu H, Wang Y. B-doped carbon coating improves the electrochemical performance of electrode materials for Li-ion batteries. Adv Funct Mater 2014;24:5511-21.
36. Rajagopalan R, Zhang L, Dou SX, Liu H. Lyophilized 3D lithium vanadium phosphate/reduced graphene oxide electrodes for super stable lithium ion batteries. Adv Energy Mater 2016;6:1501760.
37. Wang C, Shen W, Liu H. Nitrogen-doped carbon coated Li3V2(PO4)3 derived from a facile in situ fabrication strategy with ultrahigh-rate stable performance for lithium-ion storage. New J Chem 2014;38:430-6.
38. Zhang LL, Li Z, Yang XL, et al. Binder-free Li3V2(PO4)3/C membrane electrode supported on 3D nitrogen-doped carbon fibers for high-performance lithium-ion batteries. Nano Energy 2017;34:111-9.
39. Oh W, Park H, Jin BS, Thangavel R, Yoon WS. Understanding the structural phase transitions in lithium vanadium phosphate cathodes for lithium-ion batteries. J Mater Chem A 2020;8:10331-6.
40. Zhang C, Li H, Ping N, Pang G, Xu G, Zhang X. Facile synthesis of nitrogen-doped carbon derived from polydopamine-coated
41. Su J, Wu XL, Lee JS, Kim J, Guo YG. A carbon-coated Li3V2(PO4)3 cathode material with an enhanced high-rate capability and long lifespan for lithium-ion batteries. J Mater Chem A 2013;1:2508-14.
42. Böckenfeld N, Balducci A. The influence of carbon content on the lithium diffusion and electrochemical properties of lithium vanadium phosphate. J Appl Electrochem 2014;44:467-74.
43. Li G, Zhang Z, Wang R, Huang Z, Zuo Z, Zhou H. Effect of trace Al surface doping on the structure, surface chemistry and low temperature performance of LiNi0.5Co0.2Mn0.3O2 cathode. Electrochim Acta 2016;212:399-407.
44. Li J, Zhu Y, Wang L, Cao C. Lithium titanate epitaxial coating on spinel lithium manganese oxide surface for improving the performance of lithium storage capability. ACS Appl Mater Interfaces 2014;6:18742-50.
45. Hagh NM, Amatucci GG. Effect of cation and anion doping on microstructure and electrochemical properties of the LiMn1.5Ni0.5O4-δ spinel. J Power Sources 2014;256:457-69.
Cite This Article
Export citation file: BibTeX | EndNote | RIS
OAE Style
Liang M, Wang Y, Dong H, Wang L, Peng Q, Yang C, Xiao Y, Wang Y, Chou S, Sun B, Chen S. Surface engineering of Li3V2(PO4)3-based cathode materials with enhanced performance for lithium-ion batteries working in a wide temperature range. Microstructures 2024;4:2024034. http://dx.doi.org/10.20517/microstructures.2023.90
AMA Style
Liang M, Wang Y, Dong H, Wang L, Peng Q, Yang C, Xiao Y, Wang Y, Chou S, Sun B, Chen S. Surface engineering of Li3V2(PO4)3-based cathode materials with enhanced performance for lithium-ion batteries working in a wide temperature range. Microstructures. 2024; 4(3): 2024034. http://dx.doi.org/10.20517/microstructures.2023.90
Chicago/Turabian Style
Minxia Liang, Yiting Wang, Hanghang Dong, Lei Wang, Qianqian Peng, Chao Yang, Yao Xiao, Yong Wang, Shulei Chou, Bing Sun, Shuangqiang Chen. 2024. "Surface engineering of Li3V2(PO4)3-based cathode materials with enhanced performance for lithium-ion batteries working in a wide temperature range" Microstructures. 4, no.3: 2024034. http://dx.doi.org/10.20517/microstructures.2023.90
ACS Style
Liang, M.; Wang Y.; Dong H.; Wang L.; Peng Q.; Yang C.; Xiao Y.; Wang Y.; Chou S.; Sun B.; Chen S. Surface engineering of Li3V2(PO4)3-based cathode materials with enhanced performance for lithium-ion batteries working in a wide temperature range. Microstructures. 2024, 4, 2024034. http://dx.doi.org/10.20517/microstructures.2023.90
About This Article
Special Issue
Copyright
Data & Comments
Data
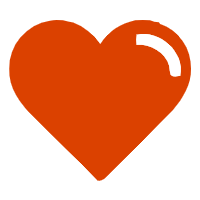
Comments
Comments must be written in English. Spam, offensive content, impersonation, and private information will not be permitted. If any comment is reported and identified as inappropriate content by OAE staff, the comment will be removed without notice. If you have any queries or need any help, please contact us at support@oaepublish.com.