Extracellular vesicles of Lactiplantibacillus plantarum PCM 2675 and Lacticaseibacillus rhamnosus PCM 489: an introductory characteristic
Abstract
Aim: Extracellular vesicles (EVs) are involved in intercellular and interkingdom communication in the complex communities that constitute the niche-specific microbiome of the colonized host. Therefore, studying the structure and content of EVs produced by resident bacteria is crucial to understanding their functionality and impact on the host and other microorganisms.
Methods: Bacterial EVs were isolated by differential centrifugation, their size and concentration were measured by transmission electron microscopy and nanoparticle tracking analysis, and the cargo proteins were identified by liquid chromatography coupled to tandem mass spectrometry. The cytotoxicity of bacterial EVs was tested using the human epithelial cell line A549 and an in vivo model of Galleria mellonella larvae.
Results: The isolation and preliminary characteristics of EVs from two strains of lactic acid bacteria - Lactiplantibacillus plantarum PCM 2675 and Lacticaseibacillus rhamnosus PCM 489 - were presented, confirming the production of vesicular structures with sizes in the range of 50-170 nm for L. plantarum and 80-250 nm for
Conclusions: A better understanding of the composition and functionality of bacterial EVs may contribute to their future effective use in supporting human health.
Keywords
INTRODUCTION
Lactic acid bacteria Lactiplantibacillus plantarum and Lacticaseibacillus rhamnosus, being found naturally in fermented foods and residing in different niches of the host organism, exhibit a number of beneficial functions for human health and could therefore be considered probiotics[1]. As recent studies have shown, these species contribute to the maintenance of oral and gut health by interacting with other microorganisms in microbiome, inducing changes in the specific inhabited niche, competing with pathogens for surfaces and nutrients, releasing substances with antimicrobial properties, and modulating of the host immune system[2,3].
Considering the alteration of the balance in different niches of the host organism, several studies have been conducted recently on the impact of L. plantarum and L. rhamnosus on other residing microorganisms. In the case of microorganisms inhabiting the first part of the digestive system, that is, the oral cavity, the expression of virulence-related genes and the formation of biofilms by bacteria Streptococcus mutans and fungi Candida albicans - key pathogens responsible for different disorders in the mouth, including dental caries and oral thrush - are affected by L. plantarum strain 14917[4,5]. In addition, plantaricin, an antimicrobial peptide produced by this strain, inhibited the growth of these pathogenic microorganisms[6]. In other studies, L. plantarum VHProbi® V38 demonstrated antimicrobial activity against not only
Additionally, studies on the mechanisms of the regulation of intestinal microbiota have shown that
Currently, postbiotics specified as inanimate probiotic microorganisms, their components or products including bacteriocins, lactic acid, hydrogen peroxide, and others attract significant attention due to their health-promoting potential for use, while reducing the risk associated with the overgrowth of viable microbial cells, development of infections in immunocompromised individuals, or horizontal gene transfer from probiotics to other bacteria contributing to the spread of antibiotic resistance[17-20]. As demonstrated recently in numerous studies, postbiotics might be involved in inhibiting pathogenic bacteria and in immunomodulatory activities, resulting in beneficial effects for host and regulation of the inflammatory processes[21]. Postbiotics from L. rhamnosus GG were capable of inhibiting S. mutans biofilm, metabolic activity, and expression of gene gtfB[22], while postbiotics from L. plantarum PD18 prevented biofilm formation by S. mutans and P. gingivalis[23]. L. plantarum postbiotics also suppressed the pathogenicity of Salmonella enterica Typhimurium by reducing the expression of virulence-related genes, pili- and flagellum-encoding genes, and biofilm formation-related genes[24]. L. plantarum and L. rhamnosus postbiotics inhibited the viability and metabolic activity of fungi Candida parapsilosis, also affecting interactions of fungal cells with vaginal epithelium[25]. Therefore, not only live bacterial cells, but also their products and derivatives may be beneficial in the maintenance of healthy balance in inhabited niches, including the oral cavity, further sections of the gastrointestinal tract, or the vagina[9,20,25]. This group of particles includes bacterial extracellular vesicles (EVs) - nanometer-sized structures enclosed with a lipid bilayer and containing a wide variety of biologically active molecules. To date, a wide range of functions of probiotic EVs have been demonstrated to support host health through various mechanisms. The specific roles of EVs produced by probiotics in interspecies and interkingdom communication, and their impact on various physiological and pathological processes have been summarized and detailed in recent comprehensive reviews[17,26,27]. Numerous pieces of evidence have demonstrated that these structures can modify the environment colonized by probiotics through the action of transferred different molecules (e.g., antibacterial peptides, enzymes, organic acids) so as to inhibit the growth of pathogenic microorganisms and reduce the possibility of their overgrowth and colonization of the particular host niche[17]. Moreover, probiotic EVs may support the functioning of the intestinal epithelial barrier by strengthening its stability and influencing intercellular junctions (EVs are internalized by cells via clathrin-dependent endocytosis), and they modulate the activity of the innate and adaptive immunity by regulating nuclear factor kappa B (NFκB) levels and affecting the secretion of proinflammatory and anti-inflammatory cytokines[17,27]. The proposed mechanism of the influence of probiotic EVs on immune system cells may involve the internalization of vesicles by dendritic cells, mediated via interactions with TLRs TLR2 and TLR4, followed by the activation of intracellular signaling and activation of mitogen-activated protein kinases (MAPKs) pathway, and, in consequence, differentiation of naïve T-helper cells (Th0) into regulatory T cells (Treg) that produce interleukins IL-4, IL-10, and IL-22 with an anti-inflammatory mode of action; however, in other reports, the increase in proinflammatory cytokines tumor necrosis factor-alpha (TNF-α), IL-6, and IL-8 has also been described in the presence of probiotic EVs under varying host cell states and microenvironmental conditions[26,27]. Therefore, the currently available data, which still require extensive expansion, indicate that the mechanisms of action of probiotic EVs are complex and context-dependent. These mechanisms are influenced by the host immune system status, microbiota balance, and the presence of pathogenic microorganisms or their EVs, and are also determined by the specific species or probiotic strain involved. Consequently, these factors together may either suppress or enhance the organism’s defense response, aiming to fight adverse conditions and improve overall health.
EVs are pivotal for intercellular communication and their potential use as postbiotics is currently attracting research attention due to the greater safety of postbiotics in individuals with impaired immunity, compared to the use of live bacterial cells[26,28]. This requires an understanding of the conditions of effective production of EVs and an assessment of their non-toxicity after contact with host. Thus, the primary aims of this short communication are (i) to isolate EVs produced by two tested bacterial strains from cultures on solid media; (ii) to characterize the morphology and sizes of EVs and conduct an initial evaluation of their proteinaceous cargo; and (iii) to perform preliminary verification of potential adverse effects on the host in selected
METHODS
Bacterial strains and growth conditions
Lactiplantibacillus plantarum PCM 2675 and Lacticaseibacillus rhamnosus PCM 489 (ATCC 9595) were purchased from Polish Collection of Microorganisms (Wroclaw, Poland) and cultivated in 20 mL of liquid De Man Rogosa and Sharp medium (MRS; Thermo Fisher Scientific, Waltham, MA, USA) in the microaerophilic atmosphere for 24 h at 37 °C, without shaking. The number of cells in liquid culture was estimated by measuring the optical density at 600 nm (OD600).
EVs isolation
For isolation of EVs, 4.5 × 109 bacterial cells were transferred to MRS agar plate [MRS broth with 1.5% (w/v) agar] and cultured for 24 h at 37 °C in an atmosphere of 5% CO2. Then, bacterial cells were carefully collected from the surface of the solid medium and transferred with sterile loop to Eppendorf tube containing 1 mL Dulbecco’s phosphate-buffered saline (DPBS), pH 7.50 ± 0.30 (Biowest, Nuaillé, France), and incubated for 10 min at room temperature. The number of viable bacterial cells producing EVs was assessed by transferring 10 µL from a series of ten-fold diluted bacterial cell suspensions, to a sterile MRS agar plate, followed by the incubation for 24 h at 37 °C and colony forming unit (CFU) counting. The next step in EVs separation from bacterial cells was the centrifugation for 10 min, 5,000 × g at 4 °C to sediment bacterial cells; after that, supernatants with EVs were transferred to the new Eppendorf tubes and further centrifuged twice for 15 min, 5,000 × g at 4 °C to remove any cells remnants. After discarding the sediment, collected supernatants were filtered using Ultrafree-CL Centrifugal Filter Unit (Merck, Darmstadt, Germany) with a pore size of 0.22 μm and transferred to sterilized polycarbonate thick wall centrifuge tubes (13 mM × 64 mM) with 13-mM-diameter Delrin tube adapters, ultracentrifuged at 4 °C for 1 h with a relative centrifugal field of 144,000 × g (k factor 112) using a fixed-angle type 60 Ti Rotor in an Optima LE-80 K Ultracentrifuge (Beckman Coulter, Brea, CA, USA). Obtained EVs pellets were suspended in 400 μL of sterile DPBS and stored at -80 °C. After each isolation, 10 µL of the obtained sample containing EVs was transferred to a sterile MRS agar medium to confirm the absence of contamination with bacterial cells after incubation for 24 h at 37 °C. The nomenclature of the obtained vesicles is as follows - LpEVs for EVs produced by L. plantarum and LrEVs for EVs produced by L. rhamnosus.
Protein and lipid concentration measurements
Protein concentrations in the EVs-containing samples were assessed using o-phthalaldehyde (OPA; Sigma-Aldrich, St. Louis, MO, USA)[29]. Briefly, 10 µL of sample and 300 µL of OPA reagent were applied to the wells of a black 96-well microplate (Greiner Bio-One, Kremsmünster, Austria) in six biological replicates, and after 5 min of incubation in the dark, the fluorescence intensity measurements were carried out at an excitation wavelength of 340 nm and emission of 450 nm using a Synergy H1 microplate reader (BioTek Instruments, Winooski, VT, USA). The phospholipid concentration in the EVs-containing samples was measured in three biological replicates using 20 µL of obtained sample of EVs of each bacterial species using the Phospholipid Assay Kit (Sigma-Aldrich) in accordance with the manufacturer’s instructions.
Transmission electron microscopy imaging and nanoparticle tracking analysis measurements
Visualization of EVs was performed with transmission electron microscopy (TEM) with the use of JEOL JEM-2100 HT microscope (JEOL, Tokyo, Japan). Formvar-coated, 300 mesh copper grids were prepared for each vesicle sample by applying 7 µL of EVs suspension in DPBS buffer and allowing it to be adsorbed, and then negative staining using 2% uranyl acetate was performed (Chemapol, Prague, Czech Republic). The TEM images were acquired using a 4 k × 4 k camera (TVIPS) equipped with the EMMENU software version 4.0.9.87. Information on the size and concentration of the bacterial EVs was obtained using nanoparticle tracking analysis (NTA). Prepared samples were measured in a flow mode using NanoSight NS300 system, Blue488 laser, and NTA software Version 3.4 (Malvern Instruments, Malvern, UK). Each sample diluted in 0.22 µM-filtered DPBS, pH 7.50 ± 0.30 (Lonza, Basel, Switzerland) was recorded at 24 °C three times for
Proteomic identification of vesicular proteins
Identification of proteins contained in bacterial EVs was performed with liquid chromatography-tandem mass spectrometry (LC-MS/MS) and UltiMate 3000 RSLCnano System coupled with Q-Exactive mass spectrometer (Thermo Fisher Scientific, Waltham, MA, USA) with DPV-550 Digital PicoView nanospray source (New Objective, Woburn, MA, USA) as described in details previously[29-31] with some minor modifications. Briefly, a sample containing EVs with a total amount of vesicular proteins equal to 20 µg was prepared in 100 μL of 100 mM Tris-HCl buffer, pH 7.60 ± 0.10, with 1% (w/v) sodium dodecyl sulfate. Then, the prepared mixture was sonicated in four cycles of 30 s each, using UP50H Compact Lab Homogenizer with 50 W, 30 kHz, amplitude 80%, cycle 0.5 (Hielscher Ultrasonics, Teltow, Germany), incubated for 5 min at 95 °C, and centrifuged for 12 min at 12,000 × g. After that, proteins were precipitated overnight with trichloroacetic acid at -20 °C, centrifuged at 10,000 × g for 15 min at 10 °C, and washed twice with ice-cold acetone. The obtained precipitate was dissolved in 100 μL of 10 mM N-2-hydroxyethylpiperazine-N’-2-ethanesulfonic acid (HEPES) buffer, pH 8.50 ± 0.20 and digested with 30 µL of 0.027 µg/µL Trypsin/Lys-C Mix (Promega, Mannheim, Germany). The obtained and separated peptides were identified after processing of obtained RAW files by the Proteome Discoverer platform (v.1.4.1.14, Thermo Fisher Scientific) and searched using a locally installed MASCOT search engine (v.2.5.1, Matrix Science, London, UK) and corresponding protein databases with taxonomy restrictions SwissProtTrEMBL_Lactobacillus_plantarum (25349 sequences) or SwissProtTrEMBL_Lactobacillus_ rhamnosus (15335 sequences) and NCBInr database (26490256 sequences) with taxonomy restriction Other Firmicutes (3598679 sequences). The search parameters were applied as follows: fixed modification - cysteine carbamidomethylation; variable modifications - methionine oxidation; precursor mass tolerance - 10 ppm; fragment mass tolerance
Measurement of hydrolytic activity of EVs
The proteolytic activity of EVs was measured according to the procedure described previously[31] using EnzChek™ Protease Assay Kit (Thermo Fisher Scientific) following the manufacturer’s instructions. Bacterial EVs (5 × 109) were incubated in 100 µL of DPBS, pH 7.50 ± 0.30 in the wells of a black, flat-bottom 96-well microplate (Greiner Bio-One) with 1 μg of BODIPY FL casein at 37 °C in the dark for 24 h. The control was BODIPY FL casein in DPBS only, without EVs. Two biological replicates with three technical replicates each were performed. The fluorescence intensity was measured with excitation and emission wavelengths of 485 and 528 nm, respectively, with a Synergy H1 microplate reader.
A549 cell culture
The A549 human lung adenocarcinoma cell line (CLL-185™) was cultured in F-12K medium (Kaighn’s modification of Ham’s F-12 medium) (Corning, New York, NY, USA) supplemented with 10% fetal bovine serum (FBS) (Gibco, Thermo Fisher Scientific), 100 U/mL penicillin, and 100 mg/mL streptomycin (Biowest) at 37 °C in the atmosphere of 5% CO2 and 95% humidity.
XTT and Lactate Dehydrogenase release assays
Human cells from the cell line A549 (2 × 104 cells) were incubated in the wells of 96-well flat base, polystyrene microplate with standard surface (Sarstedt, Nümbrecht, Germany) with 5 × 108 EVs in 100 µL of F-12K medium for 24 h, then supernatants were removed, and cells were washed twice with 200 μL DPBS. To determine cell metabolic activity, the XTT (sodium 3′-[1-(phenylaminocarbonyl)-3,4-tetrazolium]-bis (4-methoxy6-nitro) benzene sulfonic acid hydrate) reduction test was performed, and 100 μL of RPMI 1640 medium without phenol red (Biowest) and 50 μL of XTT reagent [XTT at a final concentration of 1 mg/mL (Thermo Fisher Scientific) and PMS (N-methyl dibenzopyrazine methyl sulfate) at a final concentration of
Galleria mellonella survival
The in vivo safety of bacterial EVs was evaluated using Galleria mellonella moth larvae. For each group, ten randomly selected representative individuals in their last instar were chosen. Using 10 µL Hamilton syringe (Merck), 10 µL of samples contained 108 or 109 bacterial EVs in sterile DPBS buffer, pH 7.50 ± 0.30 (Biowest) were administered in the left last proleg of each larva. Survival at 37 °C was monitored for the next 7 days. Four or three biological replicates were performed for LpEVs or LrEVs, respectively.
Statistical analysis
To analyze the statistical significance, an unpaired t-test or one-way ANOVA with Dunnett’s multiple comparisons test (for data shown as bar graphs) and Log-rank (Mantel-Cox) test (for survival rate analysis) were performed with GraphPad Prism software version 10.0.3 (GraphPad Software, La Jolla, CA, USA). Results on bar graphs are presented as mean ± standard deviation. Statistical significance levels versus control were indicated by **** for P < 0.0001, and ns when not significant.
RESULTS
Bacterial EVs were isolated from cultures on solid media using a previously described method[29]. Briefly, bacterial strains L. plantarum PCM 2675 and L. rhamnosus PCM 489 (ATCC 9595) were first cultivated at 37 °C under microaerophilic conditions in MRS liquid medium, and after 24 h, each culture was diluted a hundred times, resulting in OD600 of 0.95 for L. plantarum and 0.39 for L. rhamnosus. Subsequently, 54 and 130 µL of each diluted culture were transferred to MRS agar plates for further growth under the same conditions. To minimize the accumulation of the sedimented and concentrated liquid medium components in the obtained EVs-containing samples, only bacterial cells were collected from the solid medium. In addition, after each isolation, it was confirmed that the obtained EV samples did not contain any viable bacterial cells. The procedure for isolating bacterial EVs from cultures on solid media is schematically presented in Figure 1.
Figure 1. Step-by-step procedure for isolation of bacterial EVs from cells grown on MRS solid media. The figure was partly generated using Servier Medical Art, provided by Servier, licensed under a Creative Commons Attribution 4.0 unported license. EVs: Extracellular vesicles; MRS: De Man Rogosa and Sharp.
NTA-based size distribution measurement of isolated particles indicated a mode value of 99.9 ± 3.1 nm for EVs produced by L. plantarum [Figure 2A], and 95.7 ± 5.3 nm for L. rhamnosus [Figure 3A]. The number of vesicles produced by the detected number of living bacterial cells collected from one MRS agar plate has been assessed based on particle concentration measurement with NTA and on CFU counting. Accomplished estimations indicated that 2.86 × 1012 viable L. plantarum cells enabled the isolation of about 2.58 × 1011 EVs, and for 1.08 × 1012 viable L. rhamnosus cells, about 3.66 × 1010 particles were isolated. Using TEM, the presence and morphology of spherical structures in obtained samples were confirmed for L. plantarum [Figure 2B] and L. rhamnosus [Figure 3B]. Notably, some heterogeneity in EVs populations was observed, particularly for L. rhamnosus. Furthermore, the presence of structures composed of lipids and proteins was also confirmed by the measurement of their average concentration in the obtained EVs-containing samples. The average phospholipid concentration was 17 ± 1.6 µM for LpEVs and 8.8 ± 0.5 µM for LrEVs, while the average protein concentration was 0.93 ± 0.15 mg/mL and 1.06 ± 0.16 mg/mL for LpEVs and LrEVs, respectively.
Figure 2. Characteristics of EVs produced by L. plantarum PCM 2675. (A) NTA-based particle size distribution analysis; a representative histogram of the average size distribution from three measurements of a single sample (black line) is presented. The presented blue numbers indicate the maxima of peaks, and the red areas show the SD between measurements. The size parameters of EVs are included. Factors D10, D50, and D90 denote that 10%, 50%, and 90% of the EV population had a diameter of less than or equal to the presented value; (B) TEM images of LpEVs. White arrows point to the individual particles. Scale bars included in individual panels. EVs: Extracellular vesicles; NTA: nanoparticle tracking analysis; TEM: transmission electron microscopy; SD: standard deviation.
Figure 3. Characteristics of EVs from L. rhamnosus PCM 489. (A) NTA-based particle size distribution analysis; a representative histogram of the average size distribution from three measurements of a single sample (black line) is presented. The presented blue numbers indicate the maxima of peaks, and the red areas show the SD between measurements. The size parameters of EVs are included. Factors D10, D50, and D90 denote that 10%, 50%, and 90% of the EV population had a diameter of less than or equal to the presented value; (B) TEM images of LrEVs. White arrows point to the individual particles. Scale bars included in individual panels. EVs: Extracellular vesicles; NTA: nanoparticle tracking analysis; TEM: transmission electron microscopy; SD: standard deviation.
Subsequently, proteomic identification of proteins found in bacterial vesicles was performed in duplicate with the shotgun proteomic approach and LC-MS/MS. Identifying these proteins and indicating their functions might not only elucidate the mechanisms by which probiotic EVs interact with other cells, but also imply potential biogenesis pathways, and enable the projection of further application of EVs across various fields. The analyses showed some discrepancy in the number of identified proteins in both samples, despite the same protein concentration. For LpEVs and LrEVs, the total amount of protein subjected to analysis was the same and the obtained mass spectra had similar intensities, so the differences in the number of identified proteins most likely result from the available content of the databases for both tested species. For L. plantarum, all identified proteins are listed in Supplementary Table 1, while for L. rhamnosus in Supplementary Table 2, and for further comparisons, proteins identified in both replicates were selected. The analysis of the location and functions of identified vesicular proteins [Figure 4] was performed by assigning specific annotations to individual molecules based on data included in the UniProt protein database (https://www.uniprot.org/)[33] and InterPro protein families database (https://www.ebi.ac.uk/inter pro/)[34].
Figure 4. Characteristics of proteins identified in LpEVs and LrEVs. (A) Proteins grouped by their location; (B) Proteins grouped by their function.
In the case of EVs of both species tested, primarily membrane (30% for LpEVs and 32% for LrEVs) and cytoplasmic (36% for LpEVs and 32% for LrEVs) proteins were detected among proteins with known locations; additionally, molecules with an assigned extracellular location were also identified (11% for LpEVs and 4% for LrEVs) [Figure 4A]. That would confirm the origin of EVs as extracellular structures originating from the cell, surrounded by a membrane, and transporting some of the intracellular proteins[35]. Classification of proteins based on their function assignment showed that vesicular proteins identified for L. plantarum are involved mostly in protein biosynthesis and transport (13%), transcription and translation (16%), cell wall maintenance (11%), carbohydrate metabolism (11%), while for L. rhamnosus in the cell wall biosynthesis and remodeling (12%), carbohydrate metabolism (16%) and other metabolic pathways (20%) [Figure 4B]. Proteins involved in the transport of distinct types of molecules (1% for LpEVs or 8% for LrEVs) and in the interactions with ligands at the cell surface (6% for LpEVs or 16% for LrEVs) were also identified for both species. Taking into consideration proteins responsible for transport, for LpEVs, adenosine triphosphate-binding cassette (ABC)-type oligopeptide transport system, amino acid ABC transporters, bacteriocin transport accessory protein, manganese transport protein MntH have been identified, and for LrEVs, ABC transporter substrate-binding protein, oligopeptide ABC transporter substrate-binding protein, calcium ABC transporter adenosine triphosphate (ATPase), phosphotransferase system (PTS sugar transporter), and ABC transporter permease have been found in EVs. Among the proteins involved in metabolism, lactate dehydrogenase, glyceraldehyde-3-phosphate dehydrogenase, enolase, and glucose-6-phosphate isomerase have been identified in EVs of both tested species, and fructose-1,6-bisphosphate aldolase, phosphoglycerate kinase and pyruvate kinase in LpEVs, and tagatose 1,6-diphosphate aldolase in LrEVs.
The proteomic identification of vesicular proteins indicated the presence of various enzymes within bacterial EVs, prompting further investigation into the proteolytic activity of these EVs. Among the identified enzymes in LpEVs were extracellular zinc metalloproteinase Zmp2, peptidase Do, dipeptidase PepV, serine-type D-Ala-D-Ala carboxypeptidase, and in LrEVs, carboxypeptidase, aminopeptidase, endopeptidase La, S8 family serine peptidase, serine-type D-Ala-D-Ala carboxypeptidase, and ATP-dependent zinc metalloprotease FtsH [Supplementary Tables 1 and 2]. For both tested species, the noticeable ability to hydrolyze peptide bonds has been demonstrated with the use of casein as a proteinaceous substrate [Figure 5A].
Figure 5. The effect of bacterial EVs on the host. (A) EVs-related proteinase activity measured with BODIPY FL casein as a substrate. The control sample was DPBS with substrate, without EVs. Statistical significance levels versus control are marked as **** for P < 0.0001, and ns when not significant; (B) The survival curve of Galleria mellonella larvae after the injection of EVs produced by L. plantarum (LpEV) and L. rhamnosus (LrEV). Injection with DPBS served as a control; (C) The metabolic activity and (D) LDH release by A549 epithelial cells following treatment with 5 × 108 EVs produced by L. plantarum (LpEV) and L. rhamnosus (LrEV). Untreated epithelial cells and lysed cells served as negative and positive controls, respectively. Statistical significance levels versus control: ns when not significant. Evs: Extracellular vesicles; DPBS: Dulbecco’s phosphate-buffered saline.
Since, in addition to examining the composition of EVs, it is also critical to verify their impact on the host and test whether they are non-toxic to host cells, an in vitro model using human epithelial cells and an
Moreover, the effect of EVs released by both bacterial species on human epithelial cell line A549 was tested with the XTT assay and with the measurement of LDH release [Figure 5]. The metabolic activity of A549 cells after incubation with LpEVs and LrEVs demonstrated a slight decrease, but without statistical significance [Figure 5C]. Furthermore, the measurement of LDH release, correlating with the level of the cell damage, indicated the comparable level of released LDH after treatment of cells with bacterial EVs compared to the control cells, as the minor changes were statistically not significant [Figure 5D].
DISCUSSION
The knowledge of EVs production by Gram-positive bacteria is still incomplete and the mechanisms of their biogenesis and release require intensive investigation, as they differ from those reported for Gram-negative bacteria, also due to the presence of a thick layer of cell wall peptidoglycan on the bacterial cell surface[36]. Thus far, it has been indicated that EVs released by Gram-positive bacteria may have various biogenesis pathways, often accompanied by bacterial cell wall degradation, and based on membrane blebbing and explosive cell lysis[37]. This may also result in significant heterogeneity of these structures, considering not only their sizes, but also composition and properties. In general, the isolation of bacterial EVs also presents challenges that necessitate optimization for both efficiency and contamination-free preparations. Purification of EVs from liquid cultures may pose a risk of co-isolation of the medium components concentrated together with vesicles[38]. Therefore, in the case of other microorganisms, an improved method of EV isolation has recently been introduced, which involves EVs isolation from fungal cells cultured on solid media. This approach minimized the risk of co-concentration of the medium components, since only microbial cells were collected from the surface of plates containing solid media, and then suspended in a phosphate-buffered saline. In addition, the noteworthy advantages of this method, compared to isolation from a liquid medium, include significantly reduced procedural time and decreased labor intensity. Thus, the procedure of isolating EVs from solid media cultures has been considered fast, reproducible, and reliable[39]. Therefore, in this work, we proposed the use of this method for the isolation of EVs produced by two species of Gram-positive bacteria that are classified as probiotic microorganisms, and we presented their preliminary characteristics to provide an introduction to further advanced research on their functionality as postbiotics. The pleiotropic effects of postbiotics from lactic acid bacteria currently attract significant interest. Their wide application range includes nutritional support, modulation of the host immune response, anticancer activity, and antimicrobial action against different pathogenic microorganisms[17,26,27,40-44]. EVs produced by Lactobacillaceae, considered as bioactive compounds, fit into the criteria established for postbiotics, and their beneficial effects, often comparable to those of viable bacteria, have been repeatedly demonstrated[45,46]. Therefore, a more detailed knowledge about EVs may contribute to a better understanding of their application possibilities, and some interesting studies with physicochemical characteristics and proteomic analysis of EVs produced by different lactobacilli have been recently published[47-52].
In this work, EVs from two strains from this family - Lactiplantibacillus plantarum PCM 2675 and Lacticaseibacillus rhamnosus PCM 489 - were isolated and characterized. Their sizes correspond to those specified for other strains, although the reported ranges are quite variable. EVs produced by L. plantarum strain WCFS1 were in the range of 31-200 nm[47], for strain KCTC 11401BP 20-100 nm[53], and for strain BGAN8 20-140 nm[48] with the average size of about 50 nm as a monomer and about 800 nm as a multimeric structure[49]. For EVs derived from L. plantarum Q7, their sizes ranged from 70 nm to 500 nm with a mean size of 185.5 ± 65.4 nm[54], and the mean size of EVs isolated from L. plantarum APsulloc 331261 was
Proteomic analysis demonstrated that several proteins characterized in L. plantarum PCM 2675 EVs are membrane-located, similarly as described in the other proteomic studies of EVs derived from L. plantarum strains WCFS1, BGAN8, JCM8341, and BCRC 10069[47-49,59]. Herein, numerous proteins involved in carbohydrate metabolic pathways, cell division, transport, cell wall homeostasis and communication were found within the protein cargo of LpEVs. For EVs from L. rhamnosus PCM 489, identified proteins included cell metabolism enzymes, membrane proteins related to transport, and enzymes involved in cell wall remodeling and catabolism. In the identification of vesicular proteins for the L. plantarum strain BGAN8, numerous membrane proteins and transport-related proteins were also found as EVs cargo[47,48]. Some of the identified vesicular proteins, including glyceraldehyde-3-phosphate dehydrogenase (GAPDH), identified both in LpEVs and LrEVs, might be involved in the interactions with host proteins mucin and fibronectin[60]. GAPDH was also identified in EVs produced by L. plantarum in other studies[48]. Moreover, in LpEVs, additional mucus-binding proteins were identified herein, as well as D- and L-lactate dehydrogenases, enzymes crucial for bacterial physiology and interactions with host and other microorganisms that constitute microbiome[61,62]. Additionally, ABC transporters and bacteriocin transport accessory proteins that were identified in this work in bacterial EVs, are involved in the secretion of antimicrobial peptides - bacteriocins[63], and the cysteine peptidases from the C39 family are responsible for hydrolysis of the leader peptides from the precursors of various bacteriocins[64]. In the proteomic studies of EVs from other strains and species of Lactobacillaceae, molecules such as lipoproteins, lactate dehydrogenase, mucus binding proteins, ABC transporters, peptidases and lysozyme were also identified[48,49,50,59], which indicates important similarities in the protein content of EVs characterized for different representatives of lactobacilli. In addition, the presence of hydrolases among vesicular proteinaceous cargo and the hydrolytic activity presented by bacterial EVs might be related to the mechanism of their release from the bacterial cell, which is not yet sufficiently well understood for Gram-positive bacteria, but possibly can involve the degradation of cell wall peptidoglycan by enzymes, including lysozyme and peptidases, carried within or at the surface of membrane vesicles[49,65]. Several types of proteases have been identified so far within vesicles of other species of Lactobacillaceae, including zinc metalloproteases and serine proteases[48,49,59]. Importantly, this study demonstrates the genuine proteolytic activity of bacterial EVs, supported by the proteomic identification of several potential proteases within EVs cargo. As a wide range of proteases produced by lactic acid bacteria demonstrate specificity toward the milk protein casein[66], this protein was also used herein to demonstrate that the proteases in EVs produced by the two bacterial strains tested are also hydrolytically active. The proteolytic activity of lactic acid bacteria is favorably related to their application in industry, i.e., in food fermentation, as their probiotic properties significantly increase the health benefits for consumers of such products[67].
The involvement of EVs released by probiotic bacteria in the different types of interactions with host cells has been repeatedly demonstrated; thus, the verification of their non-toxicity for the host is of particular importance[26]. In our study, the cytotoxic effect of LpEVs and LrEVs was tested using the A549 epithelial cell line isolated from lung tissue of lung cancer patient[68] and an in vivo invertebrate model of G. mellonella larvae. Epithelial cells are often the first line of contact with probiotics or postbiotics after their administration. The decision to use this specific cell line was based on reports indicating that oral or respiratory administration of probiotics, including L. plantarum and L. rhamnosus, or postbiotics derived therefrom, can be beneficial for host during different respiratory diseases, including bronchopulmonary dysplasia, chronic obstructive pulmonary disease, pneumonia, cystic fibrosis, infection with influenza virus, and lung cancer[69,70]. The changes observed in these studies were not statistically significant, but some very slight effects were detectable. In other studies, there were no significant changes in the metabolic activity of Caco-2 cells after 24-h incubation with EVs from L. plantarum WCFS1[47]. When the toxicity of EVs from
In addition to in vitro tests, it is also important to analyze the impact of EVs on the host in the in vivo approach[43]. In such a model, the involvement of many different cell types and host systems in response to a stimulus is considered. Previously, we tested the mortality of G. mellonella larvae after injection of EVs derived from probiotic bacteria S. salivarius K12 and yeast S. boulardii CNCM I-745, and there was no statistically significant difference in larval survival between the EVs and the DPBS injection[29]. In the current study for EVs from L. plantarum and L. rhamnosus tested strains, the effect on larvae viability was also not statistically significant; however, a minor reduction in the survival rate was observed, which correlated with the increased amount of injected EVs.
The characterization of EVs from L. plantarum PCM 2675 and L. rhamnosus PCM 489 showed that these structures are similar to EVs previously described for other strains within the same species. Specifically, they are comparable in size, proteomic content, and effect on host cells. Therefore, the EVs isolation method employed in this work produced structures with features similar to those obtained in other studies, but with a shorter procedure time. In addition, the presented results provided evidence for the potential applications of LpEVs and LrEVs in different contexts where probiotic properties could benefit host health. However, careful observation of the effects of probiotic vesicular structures on the host during functional studies is certainly required, especially regarding the dependence of the response on the EVs concentration and method of administration.
A potential future application of probiotic-derived EVs may be their use as drug nanocarriers directed to a specific therapeutic target[78,79]. A significant advantage of EVs released by bacteria is their rapid production capacity, which surpasses that of mammalian systems. Moreover, probiotic EVs are well-tolerated by the host organism, as they originate from microorganisms that are part of the physiological microbiota[45]. A particularly promising strategy is the engineering of bacterial vesicles, based either on the genetic modification of selected strains to increase the production of EVs or to specifically change their properties and content, or direct modification of already produced bacterial structures[80]. Their application in treating various disorders, including chronic wounds, central nervous system diseases, cancer, and osteoporosis, is currently intensively studied[80-82]. An interesting example of such an approach was the construction of recombinant probiotic strain Escherichia coli Nissle 1917 with overexpression of chemokine receptor CXCR4 and growth factor BMP-2, which were subsequently presented on the surface of produced EVs as fusion proteins with bacterial surface protein ClyA[83,84]. Thus, engineered vesicles have been later employed in the approach to the treatment of osteoporosis in an ovariectomized mouse model, yielding promising results[84]. Research attempts are also in progress to enable various vesicular preparations to be administered not only intravenously, but also superficially, orally or through aerosols, which would significantly facilitate the therapeutic process and reduce the inconvenience for patients[85,86]. Considering the health benefits of probiotics and the versatile applications of modified EVs, enhancing the targeting and effectiveness of probiotic-derived EVs, as well as combining them with other materials, could significantly contribute to the design and development of innovative therapeutic strategies.
DECLARATIONS
Acknowledgments
We acknowledge the Proteomics and Mass Spectrometry Core Facility of the Malopolska Centre of Biotechnology for protein identification.
Authors’ contributions
Methodology: Kowalik K, Kulig K, Barczyk-Woznicka O, Karnas E, Karkowska-Kuleta J
Investigation: Kowalik K, Kulig K, Barczyk-Woznicka O, Karnas E
Resources: Rapala-Kozik M, Pyza E, Zuba-Surma E
Writing - original draft preparation: Kulig K, Kowalik K
Writing - review and editing: Karkowska-Kuleta J, Pyza E, Zuba-Surma E, Rapala-Kozik M
Funding acquisition: Karkowska-Kuleta J
Availability of data and materials
The datasets generated during the current study are available in the Cracow Open Research Data Repository, https://doi.org/10.57903/UJ/K93MBY. The mass spectrometry proteomics data have been deposited to the ProteomeXchange Consortium via the PRIDE partner repository[32] with the dataset identifiers PXD048874 (L. plantarum) and PXD048876 (L. rhamnosus).
Financial support and sponsorship
This work was financially supported by the National Science Centre, Poland (no. 2021/43/D/NZ6/01464 to J.K.K).
Conflicts of interest
All authors declared that there are no conflicts of interest.
Ethical approval and consent to participate
Not applicable.
Consent for publication
Not applicable.
Copyright
© The Author(s) 2024.
Supplementary Materials
REFERENCES
1. Önning G, Palm R, Linninge C, Larsson N. New Lactiplantibacillus plantarum and Lacticaseibacillus rhamnosus strains: well tolerated and improve infant microbiota. Pediatr Res. 2022;91:1849-57.
2. Avila M, Ojcius DM, Yilmaz O. The oral microbiota: living with a permanent guest. DNA Cell Biol. 2009;28:405-11.
3. Saïz P, Taveira N, Alves R. Probiotics in oral health and disease: a systematic review. Applied Sciences. 2021;11:8070.
4. Zeng Y, Fadaak A, Alomeir N, et al. Lactobacillus plantarum Disrupts S mutans-C. albicans cross-kingdom biofilms. Front Cell Infect Microbiol. 2022;12:872012.
5. Bao J, Huang X, Zeng Y, et al. Dose-dependent inhibitory effect of probiotic Lactobacillus plantarum on Streptococcus mutans-Candida albicans cross-kingdom microorganisms. Pathogens. 2023;12:848.
6. Zeng Y, Fadaak A, Alomeir N, et al. Effect of probiotic Lactobacillus plantarum on Streptococcus mutans and Candida albicans clinical isolates from children with early childhood caries. Int J Mol Sci. 2023;24:2991.
7. Zhang J, Duan Z. Identification of a new probiotic strain, Lactiplantibacillus plantarum VHProbi® V38, and its use as an oral health agent. Front Microbiol. 2022;13:1000309.
8. Zhang J, Li K, Bu X, Cheng S, Duan Z. Characterization of the anti-pathogenic, genomic and phenotypic properties of a Lacticaseibacillus rhamnosus VHProbi M14 isolate. PLoS One. 2023;18:e0285480.
9. Giordani B, Parolin C, Vitali B. Lactobacilli as anti-biofilm strategy in oral infectious diseases: a mini-review. Front Med Technol. 2021;3:769172.
10. Wang X, Tang J, Zhang S, Zhang N. Effects of Lactiplantibacillus plantarum 19-2 on immunomodulatory function and gut microbiota in mice. Front Microbiol. 2022;13:926756.
11. Yu YY, Wu LY, Sun X, Gu Q, Zhou QQ. Effect of Lactobacillus plantarum ZFM4 in Helicobacter pylori-infected C57BL/6 mice: prevention is better than cure. Front Cell Infect Microbiol. 2023;13:1320819.
12. Song Y, Sun M, Mu G, Tuo Y. Exopolysaccharide produced by Lactiplantibacillus plantarum Y12 exhibits inhibitory effect on the Shigella flexneri genes expression related to biofilm formation. Int J Biol Macromol. 2023;253:127048.
13. Mathipa-Mdakane MG, Thantsha MS. Lacticaseibacillus rhamnosus: a suitable candidate for the construction of novel bioengineered probiotic strains for targeted pathogen control. Foods. 2022;11:785.
14. Echegaray N, Yilmaz B, Sharma H, et al. A novel approach to Lactiplantibacillus plantarum: from probiotic properties to the omics insights. Microbiol Res. 2023;268:127289.
15. Garcia-Gonzalez N, Battista N, Prete R, Corsetti A. Health-promoting role of Lactiplantibacillus plantarum isolated from fermented foods. Microorganisms. 2021;9:349.
16. Segers ME, Lebeer S. Towards a better understanding of Lactobacillus rhamnosus GG--host interactions. Microb Cell Fact. 2014;13 Suppl 1:S7.
17. Sanwlani R, Bramich K, Mathivanan S. Role of probiotic extracellular vesicles in inter-kingdom communication and current technical limitations in advancing their therapeutic utility. Extracell Vesicles Circ Nucleic Acids. 2024;5:609-26.
20. Petrariu OA, Barbu IC, Niculescu AG, et al. Role of probiotics in managing various human diseases, from oral pathology to cancer and gastrointestinal diseases. Front Microbiol. 2023;14:1296447.
21. Magryś A, Pawlik M. Postbiotic fractions of probiotics Lactobacillus plantarum 299v and Lactobacillus rhamnosus GG show immune-modulating effects. Cells. 2023;12:2538.
22. Banakar M, Pourhajibagher M, Etemad-Moghadam S, et al. Antimicrobial effects of postbiotic mediators derived from Lactobacillus rhamnosus GG and Lactobacillus reuteri on Streptococcus mutans. Front Biosci. 2023;28:88.
23. Butrungrod W, Chaiyasut C, Makhamrueang N, Peerajan S, Chaiyana W, Sirilun S. Postbiotic metabolite of Lactiplantibacillus plantarum PD18 against periodontal pathogens and their virulence markers in biofilm formation. Pharmaceutics. 2023;15:1419.
24. Hu A, Huang W, Shu X, et al. Lactiplantibacillus plantarum postbiotics suppress salmonella infection via modulating bacterial pathogenicity, autophagy and inflammasome in mice. Animals. 2023;13:3215.
25. Spaggiari L, Sala A, Ardizzoni A, et al. Lactobacillus acidophilus, L. plantarum, L. rhamnosus, and L. reuteri cell-free supernatants inhibit Candida parapsilosis pathogenic potential upon infection of vaginal epithelial Cells monolayer and in a transwell coculture system in vitro. Microbiol Spectr. 2022;10:e0269621.
26. Molina-Tijeras JA, Gálvez J, Rodríguez-Cabezas ME. The immunomodulatory properties of extracellular vesicles derived from probiotics: a novel approach for the management of gastrointestinal diseases. Nutrients. 2019;11:1038.
27. Rubio AP, D'Antoni CL, Piuri M, Pérez OE. Probiotics, their extracellular vesicles and infectious diseases. Front Microbiol. 2022;13:864720.
28. Kullar R, Goldstein EJC, Johnson S, McFarland LV. Lactobacillus bacteremia and probiotics: a review. Microorganisms. 2023;11:896.
29. Kulig K, Kowalik K, Surowiec M, et al. Isolation and characteristics of extracellular vesicles produced by probiotics: yeast Saccharomyces boulardii CNCM I-745 and bacterium Streptococcus salivarius K12. Probiotics Antimicrob Proteins. 2024;16:936-48.
30. Surman M, Hoja-Łukowicz D, Szwed S, et al. An insight into the proteome of uveal melanoma-derived ectosomes reveals the presence of potentially useful biomarkers. Int J Mol Sci. 2019;20:3789.
31. Karkowska-Kuleta J, Kulig K, Bras G, et al. Candida albicans biofilm-derived extracellular vesicles are involved in the tolerance to caspofungin, biofilm detachment, and fungal proteolytic activity. J Fungi. 2023;9:1078.
32. Perez-Riverol Y, Bai J, Bandla C, et al. The PRIDE database resources in 2022: a hub for mass spectrometry-based proteomics evidences. Nucleic Acids Res. 2022;50:D543-52.
33. UniProt Consortium. UniProt: the universal protein knowledgebase in 2023. Nucleic Acids Res. 2023;51:D523-31.
34. Paysan-Lafosse T, Blum M, Chuguransky S, et al. InterPro in 2022. Nucleic Acids Res. 2023;51:D418-27.
35. Gill S, Catchpole R, Forterre P. Extracellular membrane vesicles in the three domains of life and beyond. FEMS Microbiol Rev. 2019;43:273-303.
36. Briaud P, Carroll RK. Extracellular vesicle biogenesis and functions in gram-positive bacteria. Infect Immun. 2020:88.
37. Jeong D, Kim MJ, Park Y, et al. Visualizing extracellular vesicle biogenesis in gram-positive bacteria using super-resolution microscopy. BMC Biol. 2022;20:270.
38. Le LHM, Steele JR, Ying L, Schittenhelm RB, Ferrero RL. A new isolation method for bacterial extracellular vesicles providing greater purity and improved proteomic detection of vesicle proteins. J Extracell Biol. 2023;2:e84.
39. Reis FCG, Borges BS, Jozefowicz LJ, et al. A novel protocol for the isolation of fungal extracellular vesicles reveals the participation of a putative scramblase in polysaccharide export and capsule construction in cryptococcus gattii. mSphere. 2019;4:e00080-19.
40. Moradi M, Kousheh SA, Almasi H, et al. Postbiotics produced by lactic acid bacteria: the next frontier in food safety. Compr Rev Food Sci Food Saf. 2020;19:3390-415.
41. Cuevas-González PF, Liceaga AM, Aguilar-Toalá JE. Postbiotics and paraprobiotics: from concepts to applications. Food Res Int. 2020;136:109502.
42. Kudra A, Kaźmierczak-Siedlecka K, Sobocki BK, et al. Postbiotics in oncology: science or science fiction? Front Microbiol. 2023;14:1182547.
43. Rawling M, Schiavone M, Mugnier A, et al. Modulation of zebrafish (Danio rerio) intestinal mucosal barrier function fed different postbiotics and a probiotic from Lactobacilli. Microorganisms. 2023;11:2900.
44. Krzyżek P, Marinacci B, Vitale I, Grande R. Extracellular vesicles of probiotics: shedding light on the biological activity and future applications. Pharmaceutics. 2023;15:522.
45. González-Lozano E, García-García J, Gálvez J, et al. Novel horizons in postbiotics: Lactobacillaceae extracellular vesicles and their applications in health and disease. Nutrients. 2022;14:5296.
46. Croatti V, Parolin C, Giordani B, Foschi C, Fedi S, Vitali B. Lactobacilli extracellular vesicles: potential postbiotics to support the vaginal microbiota homeostasis. Microb Cell Fact. 2022;21:237.
47. Li M, Lee K, Hsu M, Nau G, Mylonakis E, Ramratnam B. Lactobacillus-derived extracellular vesicles enhance host immune responses against vancomycin-resistant enterococci. BMC Microbiol. 2017;17:66.
48. Bajic SS, Cañas MA, Tolinacki M, et al. Proteomic profile of extracellular vesicles released by Lactiplantibacillus plantarum BGAN8 and their internalization by non-polarized HT29 cell line. Sci Rep. 2020;10:21829.
49. Kurata A, Kiyohara S, Imai T, et al. Characterization of extracellular vesicles from Lactiplantibacillus plantarum. Sci Rep. 2022;12:13330.
50. Dean SN, Leary DH, Sullivan CJ, Oh E, Walper SA. Isolation and characterization of Lactobacillus-derived membrane vesicles. Sci Rep. 2019;9:877.
51. Dean SN, Rimmer MA, Turner KB, et al. Lactobacillus acidophilus membrane vesicles as a vehicle of bacteriocin delivery. Front Microbiol. 2020;11:710.
52. Caruana JC, Dean SN, Walper SA. Isolation and characterization of membrane vesicles from Lactobacillus species. Bio Protoc. 2021;11:e4145.
53. Kim MH, Choi SJ, Choi HI, et al. Lactobacillus plantarum-derived extracellular vesicles protect atopic dermatitis induced by Staphylococcus aureus-derived extracellular vesicles. Allergy Asthma Immunol Res. 2018;10:516-32.
54. Hao H, Zhang X, Tong L, et al. Effect of extracellular vesicles derived from Lactobacillus plantarum Q7 on gut microbiota and ulcerative colitis in mice. Front Immunol. 2021;12:777147.
55. Kim W, Lee EJ, Bae IH, et al. Lactobacillus plantarum-derived extracellular vesicles induce anti-inflammatory M2 macrophage polarization in vitro. J Extracell Vesicles. 2020;9:1793514.
56. Behzadi E, Mahmoodzadeh Hosseini H, Imani Fooladi AA. The inhibitory impacts of Lactobacillus rhamnosus GG-derived extracellular vesicles on the growth of hepatic cancer cells. Microb Pathog. 2017;110:1-6.
57. Tong L, Zhang X, Hao H, et al. Lactobacillus rhamnosus GG derived extracellular vesicles modulate gut microbiota and attenuate inflammatory in DSS-induced colitis mice. Nutrients. 2021;13:3319.
58. Champagne-Jorgensen K, Mian MF, McVey Neufeld KA, Stanisz AM, Bienenstock J. Membrane vesicles of Lacticaseibacillus rhamnosus JB-1 contain immunomodulatory lipoteichoic acid and are endocytosed by intestinal epithelial cells. Sci Rep. 2021;11:13756.
59. Lee BH, Chen YZ, Shen TL, Pan TM, Hsu WH. Proteomic characterization of extracellular vesicles derived from lactic acid bacteria. Food Chem. 2023;427:136685.
60. Sánchez B, Schmitter JM, Urdaci MC. Identification of novel proteins secreted by Lactobacillus plantarum that bind to mucin and fibronectin. J Mol Microbiol Biotechnol. 2009;17:158-62.
62. Llibre A, Grudzinska FS, O'Shea MK, et al. Lactate cross-talk in host-pathogen interactions. Biochem J. 2021;478:3157-78.
63. Eijsink VG, Axelsson L, Diep DB, Håvarstein LS, Holo H, Nes IF. Production of class II bacteriocins by lactic acid bacteria; an example of biological warfare and communication. Antonie Van Leeuwenhoek. 2002;81:639-54.
64. Wu KH, Tai PC. Cys32 and His105 are the critical residues for the calcium-dependent cysteine proteolytic activity of CvaB, an ATP-binding cassette transporter. J Biol Chem. 2004;279:901-9.
65. Luo R, Chang Y, Liang H, et al. Interactions between extracellular vesicles and microbiome in human diseases: new therapeutic opportunities. Imeta. 2023;2:e86.
66. Christensen LF, Høie MH, Bang-Berthelsen CH, Marcatili P, Hansen EB. Comparative structure analysis of the multi-domain, cell envelope proteases of lactic acid bacteria. Microorganisms. 2023;11:2256.
67. Kieliszek M, Pobiega K, Piwowarek K, Kot AM. Characteristics of the proteolytic enzymes produced by lactic acid bacteria. Molecules. 2021;26:1858.
68. Giard DJ, Aaronson SA, Todaro GJ, et al. In vitro cultivation of human tumors: establishment of cell lines derived from a series of solid tumors. J Natl Cancer Inst. 1973;51:1417-23.
69. Du T, Lei A, Zhang N, Zhu C. The beneficial role of probiotic Lactobacillus in respiratory diseases. Front Immunol. 2022;13:908010.
70. Yuksel N, Gelmez B, Yildiz-Pekoz A. Lung microbiota: its relationship to respiratory system diseases and approaches for lung-targeted probiotic bacteria delivery. Mol Pharm. 2023;20:3320-37.
71. Fan J, Zhang Y, Zuo M, et al. Novel mechanism by which extracellular vesicles derived from Lactobacillus murinus alleviates deoxynivalenol-induced intestinal barrier disruption. Environ Int. 2024;185:108525.
72. Fakharian F, Sadeghi A, Pouresmaeili F, Soleimani N, Yadegar A. Anti-inflammatory effects of extracellular vesicles and cell-free supernatant derived from Lactobacillus crispatus strain RIGLD-1 on Helicobacter pylori-induced inflammatory response in gastric epithelial cells in vitro. Folia Microbiol (Praha). 2024;69:927-39.
73. Keyhani G, Mahmoodzadeh Hosseini H, Salimi A. Effect of extracellular vesicles of Lactobacillus rhamnosus GG on the expression of CEA gene and protein released by colorectal cancer cells. Iran J Microbiol. 2022;14:90-6.
74. Lee KS, Kim Y, Lee JH, et al. Human probiotic Lactobacillus paracasei-derived extracellular vesicles improve tumor necrosis factor-α-induced inflammatory phenotypes in human skin. Cells. 2023;12:2789.
75. Mierzejewska J, Kowalska P, Marlicka K, et al. Exploring extracellular vesicles of probiotic yeast as carriers of biologically active molecules transferred to human intestinal cells. Int J Mol Sci. 2023;24:11340.
76. Kim JH, Jeun EJ, Hong CP, et al. Extracellular vesicle-derived protein from Bifidobacterium longum alleviates food allergy through mast cell suppression. J Allergy Clin Immunol. 2016;137:507-16.e8.
77. Nasiri G, Azimirad M, Goudarzi H, et al. The inhibitory effects of live and UV-killed Akkermansia muciniphila and its derivatives on cytotoxicity and inflammatory response induced by Clostridioides difficile RT001 in vitro. Int Microbiol. 2024;27:393-409.
78. Liu H, Geng Z, Su J. Engineered mammalian and bacterial extracellular vesicles as promising nanocarriers for targeted therapy. Extracell Vesicles Circ Nucleic Acids. 2022;3:63-86.
79. Liu H, Li M, Zhang T, Liu X, Zhang H, Geng Z, Su, J. Engineered bacterial extracellular vesicles for osteoporosis therapy. Chem Eng Journ. 2022;450:138309.
80. Ji N, Wang F, Wang M, Zhang W, Liu H, Su J. Engineered bacterial extracellular vesicles for central nervous system diseases. J Control Release. 2023;364:46-60.
81. Gujrati V, Kim S, Kim SH, et al. Bioengineered bacterial outer membrane vesicles as cell-specific drug-delivery vehicles for cancer therapy. ACS Nano. 2014;8:1525-37.
82. Li Z, Liu J, Song J, et al. Multifunctional hydrogel-based engineered extracellular vesicles delivery for complicated wound healing. Theranostics. 2024;14:4198-217.
83. Liu H, Zhang H, Wang S, et al. Bone-targeted bioengineered bacterial extracellular vesicles delivering siRNA to ameliorate osteoporosis. Compos Part B Eng. 2023;255:110610.
84. Liu H, Song P, Zhang H, et al. Synthetic biology-based bacterial extracellular vesicles displaying BMP-2 and CXCR4 to ameliorate osteoporosis. J Extracell Vesicles. 2024;13:e12429.
85. Mondal J, Pillarisetti S, Junnuthula V, et al. Extracellular vesicles and exosome-like nanovesicles as pioneering oral drug delivery systems. Front Bioeng Biotechnol. 2023;11:1307878.
Cite This Article
How to Cite
Download Citation
Export Citation File:
Type of Import
Tips on Downloading Citation
Citation Manager File Format
Type of Import
Direct Import: When the Direct Import option is selected (the default state), a dialogue box will give you the option to Save or Open the downloaded citation data. Choosing Open will either launch your citation manager or give you a choice of applications with which to use the metadata. The Save option saves the file locally for later use.
Indirect Import: When the Indirect Import option is selected, the metadata is displayed and may be copied and pasted as needed.
About This Article
Copyright
Data & Comments
Data
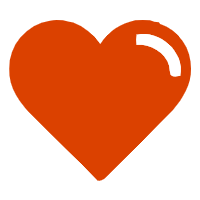
Comments
Comments must be written in English. Spam, offensive content, impersonation, and private information will not be permitted. If any comment is reported and identified as inappropriate content by OAE staff, the comment will be removed without notice. If you have any queries or need any help, please contact us at [email protected].