Cargo exchange between human and bacterial extracellular vesicles in gestational tissues: a new paradigm in communication and immune development
Abstract
Host-bacteria and bacteria-bacteria interactions can be facilitated by extracellular vesicles (EVs) secreted by both human and bacterial cells. Human and bacterial EVs (BEVs) propagate and transfer immunogenic cargos that may elicit immune responses in nearby or distant recipient cells/tissues. Hence, direct colonization of tissues by bacterial cells is not required for immunogenic stimulation. This phenomenon is important in the feto-maternal interface, where optimum tolerance between the mother and fetus is required for a successful pregnancy. Though the intrauterine cavity is widely considered sterile, BEVs from diverse sources have been identified in the placenta and amniotic cavity. These BEVs can be internalized by human cells, which may help them evade host immune surveillance. Though it appears logical, whether bacterial cells internalize human EVs or human EV cargo is yet to be determined. However, the presence of BEVs in placental tissues or amniotic cavity is believed to trigger a low-grade immune response that primes the fetal immune system for ex-utero survival, but is insufficient to disrupt the progression of pregnancy or cause immune intolerance required for adverse pregnancy events. Nevertheless, the exchange of bioactive cargos between human and BEVs, and the mechanical underpinnings and health implications of such interactions, especially during pregnancy, are still understudied. Therefore, while focusing on the feto-maternal interface, we discussed how human cells take up BEVs and whether bacterial cells take up human EVs or their cargo, the exchange of cargos between human and BEVs, host cell (feto-maternal) inflammatory responses to BEV immunogenic stimulation, and associations of these interactions with fetal immune priming and adverse reproductive outcomes such as preeclampsia and preterm birth.
Keywords
INTRODUCTION
Extracellular vesicles (EVs) are lipid bilayer, nano-sized, non-replicating (non-nucleated) subcellular particles released by all cells to facilitate cell-free intercellular communication and transport of molecules[1-3]. EVs promote cell-to-cell interaction by transferring diverse bioactive molecules and genetic information[4]. EVs represent the real-time snapshot of the parent cells’ physiologic or pathophysiologic state, and they protect their cargo (DNA, RNA, proteins, lipids, and metabolites) from degradation[5-10]. Hence, EVs serve as a valuable source of circulating indicators of the physiologic and pathologic state of a tissue that can be used as biomarkers or to develop targeted therapies[4-10]. EVs, as paracrine signalers, can deliver their cargo and elicit a response. Often, EVs interact with receptors on their target cells and trigger intracellular signaling via surface ligands[11,12]. For example, receptors expressed on EVs can engage in a ligand-receptor interaction with a cell expressing appropriate receptors, where cell signaling can be triggered. EVs can also signal through intracellular target molecules[13,14].
Like humans, prokaryotic organisms like bacteria also produce vesicles referred to as outer membrane vesicles (OMVs), primarily from Gram-negative bacteria or, in general, bacterial extracellular vesicles (BEVs)[15]. Both human and bacterial-derived EVs are widely studied, although often exclusively. Human body sites (mouth, gut, skin, respiratory, and genital tracts), where host cells co-exist with bacterial species (microbiota) in a dynamic mutualistic relationship (microbiome)[2,16-24], are sources of EVs from both humans and microbes. The host-bacteria and bacteria-bacteria interactions at such niches can be mediated by the exchange of biomolecules between both cell types, some of which are packaged and transported by EVs in a paracrine fashion[2,16,25,26]. Some researchers believe that EVs are the main communication pathway between human cells and the microbiota[27] because EVs guarantee host-microbiome interaction without direct contact and in a bidirectional fashion[24,28-30]. Importantly, this EV-mediated communication channel is utilized or exploited by both commensal and infectious species[31].
Accordingly, our group recently reported the presence of BEVs in placental tissues and postulated that the human placenta harbors BEVs secreted by endogenous commensal microbes or microbes from the individual’s environment (air, water, or food)[26]. However, the exchange of biomolecular cargos between human and bacterial EVs, and the mechanical underpinnings and health implications of such interactions, especially in pregnancy, are still understudied. Therefore, this review highlights the putative mechanisms and potential immunologic, physiologic and pathologic, biomarker, and therapeutic implications of cargo exchange between human and bacterial EVs. With a focus on the feto-maternal interface, we discussed how human cells take up BEVs and whether bacterial cells take up human EVs or their cargo, the exchange of cargos between human and BEVs, host cell (feto-maternal) inflammatory responses to BEV immunogenic stimulation, and associations of these interactions with fetal immune priming and adverse reproductive outcomes such as preeclampsia and preterm birth.
Physiological role of human EVs
Besides direct cellular contacts, multicellular organisms, including humans, employ secreted molecules packaged in EVs to mediate physiological and pathological activities[11,32,33]. Depending on their origin, size, biosynthesis, cargo contents, tissue tropism and function, human EVs are classified into exosomes
The secretion of EVs is mediated by endosomal sorting complexes required for transport (ESCRT) pathway proteins and their homologs, and ESCRT-independent pathways[42-44]. Furthermore, EVs express specific transmembrane or lipid-bound extracellular and cytosolic protein markers [Table 1][3,34,40,43,45-59]. Generally, exosomes are enriched in TSG101, ALIX, or HSP70 when the intraluminal vesicles (ILV) are formed through the ESCRT-dependent pathway. However, the tetraspanins (CD9, CD63, and CD81) are enriched when the vesicles are formed via the ESCRT-independent pathway[60-65]. Documentation of these protein markers [Table 1] is part of the minimum criteria for reporting studies involving EV isolation and characterization[3,40].
Typical human extracellular vesicle protein markers
Transmembrane or lipid-bound extracellular | Cytosolic |
Tetraspanins: CD9, CD63, CD81, CD82 | Tumor susceptibility gene 101 (TSG101) |
Major histocompatibility complex (MHC) class I (HLA-A/B/C) | Flotillin-1 and 2 (FLOT1/2) |
Epithelial cell adhesion molecule (EpCAM) | Programmed cell death 6 interacting protein (ALIX) |
Platelet endothelial cell adhesion molecule-1 (PECAM-1) | Vacuolar protein sorting-associated protein 4 (VPS4A/B) |
Intercellular adhesion molecule-1 (ICAM1) | Arrestin domain-containing protein 1 (ARRDC1) |
Heparan sulfate proteoglycans, including syndecans | Annexins (ANXA) |
Integrins (ITGA/ITGB) | Caveolins |
RHOA | |
ADP-ribosylation factor 6 (ARF6) | |
Syntenin | |
Heat shock proteins: HSC70 (HSPA8), HSP70 (HSPA1A), and HSP84 (HSP90AB1) | |
Rabs (Ras-related protein GTPases) | |
Tau [microtubule-associated proteins (MAPT), neurons] |
Humans employ EVs, especially exosomes, in several physiological processes, including cell growth, proliferation and differentiation, angiogenesis, immune responses, cellular signaling, migration, and metabolism. In pregnancy, an area where the authors of this review are primarily focused, EVs are involved in implantation[66,67], feto-placental growth[68-70], feto-maternal communication[29,30], pregnancy immune homeostasis[71-73], and most importantly, function as signalers of parturition between the fetus and the mother[35,74-77]. They are also indicated in pathological conditions, including autoimmune and neurodegenerative diseases, cancer progression, cardiovascular, respiratory, metabolic, and neurological disorders, infectious diseases, and host-bacteria interactions[13,26,36,39,42,78-82].
Cargo packaging in human (eukaryotic) EVs
EVs package their cargos through ESCRT-dependent or -independent pathways[37,38,80]. Both pathways are believed to work in synergy, which may explain how exosomes can carry unique contents different from the parental cell[38,80].
Proteins: The sorting of proteins into EVs follows specific ESCRT, tetraspanins, and lipid-dependent mechanisms[37,38,80]. The packaging of ESCRT proteins such as ALIX and TSG101, along with tetraspanins such as CD63, in EVs indicates the biogenesis as well as the selective protein sorting machinery present in EVs[37,38,44,80].
The ESCRT is composed of four multi-protein complexes (ESCRT-0, -I, -II, and –III) and their accessory proteins, particularly the AAA ATPase VPS4, VTA1, and ALIX, which successively recognize and load protein cargos into ILVs[80,83]. ESCRT facilitates the loading of ubiquitinated proteins into ILVs by recognizing the ubiquitin tags in the proteins’ lysine residue(s)[37,38,44,60,80]. For example, soluble Mycobacterium tuberculosis proteins are transported into exosomes after ubiquitination[84]. The early-acting complexes (ESCRT-0, -I, -II) contain ubiquitin-binding domains and bind to and load ubiquitinated proteins into EVs. At the same time, the late-acting ESCRT-III and VPS4 terminate EV formation and budding[44,85]. ALIX can also load proteins, for example, protease activator receptor 1 and purinergic receptor P2Y1, into developing EVs in a ubiquitin-independent manner[86,87].
In addition to ubiquitination, selective protein cargo sorting in EVs is also mediated by other post-translational modifications (PTMs), including the addition of proteins such as small ubiquitin-like modifiers (SUMO, SUMOylation), NEDD8 (NEDDylation), ISG15 (ISGylation), FAT10 (FAT10ylation)[37,38,88-90], and removal of ubiquitin (Deubiquitination)[80]. Other PTMs that can sort proteins into EVs include phosphorylation, acetylation, myristoylation, glycosylation, citrullination, oxidation, prenylation, palmitoylation, amidation, biotinylation, deamination, formylation, glycation, hydroxylation, methylation, farnesylation, glutathionylation, geranylgeranylation, mono-ADP-ribosylation, GPI-anchor, WW domain and coiled-coil domain[37,38,80,90].
These PTMs regulate the structure, subcellular localization (e.g., sorting into EVs), and function of the protein in a context-specific manner[91,92]. However, they may not be often observed in all molecules or cell types[37]. For example, besides ubiquitination, HSC71, HSP90, 14-3-3ε, C20, and pyruvate kinase type M2 (PKM2) may drive the sorting of MHC II cargo in dendritic cell (DC)-derived exosomes[93]. This shows that the sorting of MHC II into exosomes of DCs is not dependent on MHC II ubiquitination in contrast to the sorting of MHC II at MVBs destined for degradation by lysosomal hydrolases[60,94,95]. Therefore, the content of EVs does not always mirror the protein composition of the cells of origin, and this does not occur randomly[37]. However, the EV cargo changes reflect the cell's physiological or pathological state[96].
RNA: Different types of RNA species -small and long non-coding RNAs, miRNAs, mRNAs, siRNA, and structural RNAs are actively and passively packaged into EV compartments[97-99] via six different pathways: (1) RNA-binding proteins (RBP) (e.g., heterogeneous nuclear ribonucleoprotein (hnRNP) and SYNCRIP) and miRNA motifs (EXO-motifs); (2) 3’ miRNA sequence-dependent pathway; (3) miRNA-induced silencing complex (miRISC)-related pathway; (4) other RBPs-related pathways (e.g., YBX-1, Ago2, MVP, MEX3C, and La protein); (5) membrane proteins-dependent pathway (e.g., Caveolin-1, VPS4A, and nSMase2); and (6) lipid raft-related pathway (based on the mechanism of EV biogenesis)[37,38,80,100-102]. The binding and sorting of miRNAs into EVs by various RBPs and membranous proteins are summarized in the review by Groot and Lee[100].
DNA: The majority of EV-associated DNA is located on the surface of the vesicle, while the gDNA and mtDNA exist inside the vesicle, protected from digestion by DNase[103]. gDNA is believed to be packaged into EVs from micronuclei (MN), which are cytoplasmic structures derived from unstable nuclei and surrounded by nuclear membrane[104,105]. As shown in cancer cells, the unstable envelope of MN easily breaks down during cell division, releasing the gDNA and other nuclear contents. CD63 interacts with the disrupted MN and its contents to shuttle the DNA into exosomes referred to as nExo[105-107]. The ESCRT proteins can also facilitate the loading of DNA into small EVs, while ARF6 and RhoA mediate DNA loading into large EVs (MVs) from the cytosol[105,108]. Cell-free DNA (cfDNA) released by dead cells can also be packaged into EVs by sticking on the surface of released EVs[106]. However, packaging of mtDNA and other DNA types occurs through yet unknown mechanisms[105,106,109], or through pathways similar to gDNA loading[108].
Lipids: EVs are enriched in lipids, particularly the plasma membrane phosphatidylserine, sphingomyelin, and ceramide, and to a lesser extent, phosphatidylcholine, phosphatidylinositol, phosphatidylethanolamine, and phosphatidylglycerol[110,111]. Sorting of lipids and proteolipids in EVs is suggested to be associated with the yield and size of the EVs but independent of protein sorting[111] and can be mediated by lipid raft domains[38,112-114].
Cargo sorting in MVs depends on plasma membrane oligomerization[37]. Plasma membrane anchors target cytoplasmic proteins into MVs and to the budding site[115]. Like exosomes, MVs can also package proteins via the ESCRT-dependent pathway. For example, the binding of ARRDC1 and a component of ESCRT-I (TSG101) facilitates the loading of proteins into MVs and subsequent exocytosis via Gag-mediated budding[37,54,116].
In summary, packaging of EV cargo (DNA, RNA, proteins, lipids) is a selective rather than random process mediated by selective ESCRT-dependent and -independent mechanisms. Understanding the mechanistic processes is important as the pathways of EV biogenesis, cargo sorting, and packaging considerably impact the functioning of an EV, namely, the determination of its targeted destination, cargo delivery, and recipient cell’s function[37,38,80,100]. For example, genetic deletion of CD81 hinders/reduces the incorporation/presence of the Rac gene (Rho family of GTPases) within EVs[117]. DCs isolated from CD9 knockout mice also exhibit decreased EV release, and CD9 influences the Wnt (Wingless-related integration site) signaling pathway by modulating the EV packaging of β-catenin[118]. This underscores a significant role for tetraspanins in EV biogenesis[119]. More information on the role of tetraspanins in packaging EVs with specific cargo has been published[65,120,121].
Physiologic role of bacterial EVs
In response to their environment, both pathogenic and commensal bacterial species secrete EVs that are similar in size to EVs derived from eukaryotic cells [Figure 1][2,4,23,122-130]. Gram-positive and Gram-negative bacteria produce EVs that contain components of the parent cells[2,4,131]. An extensive comparison between Gram-negative and Gram-positive BEVs is published[2,4,25].
Figure 1. Comparison of human and bacterial-derived extracellular vesicles. ARF6: ADP ribosylation factor 6; ESCRT proteins (TSG101, ALIX); ICAM-1: intercellular adhesion molecule 1; LPS: lipopolysaccharide; Omp: outer membrane protein; MHC-1: major histocompatibility complex-1; Nuclei acids (DNA, RNA); Tetraspanins (CD9, CD63, CD81). Density data[130]. Created with BioRender.com.
BEVs from Gram-negative bacteria, as mentioned above, are called OMVs and are released by blebbing the bacterial outer membrane. OMVs (~20-250 nm) contain both periplasmic and cytoplasmic components [Figure 1]. Gram-negative bacteria also undergo explosive cell lysis to generate outer-inner membrane vesicles (O-IMV)[25,132]. Gram-positive bacteria form cytoplasmic membrane vesicles (CMVs, ~20-400 nm) by budding and shedding their cytoplasmic membrane, which eventually crosses the hydrolyzed peptidoglycan cell wall. CMVs carry cytosolic substances [Figure 1][25,132,133]. Additionally, both
BEVs mediate bacteria-bacteria and bacteria-host interactions, promoting healthy and pathological conditions[2,134]. BEVs facilitate quorum sensing, promoting communication and coordinating group behavior among the species[135,136]. With the aid of diverse biomolecules transported as cargo, BEVs are also involved in bacterial competition and survival, adhesion, invasion of host tissue and infection, biofilm formation, resistance to antibiotics and other environmental stressors, host immune response and evasion, and determination of cell fate (autophagy)[4,27,34,79,122,128,137-143].
Furthermore, BEVs promote the transfer of microbial genetic material (horizontal gene transfer (HGT) and the exchange of toxins and virulence factors[4,122,144,145]. BEVs contain a myriad of biomolecules, including microbial genetic components (luminal and surface-associated DNA, sRNA, mRNA, miRNA), metabolites, virulence factors (phospholipase C, alkaline phosphatase, esterase lipase, serine protease), and toxins (cholera, adenylate cyclase, cytolethal distending, VacA, PagJ, PagK1)[2,122,123,144]. They also contain proteins and glycoproteins that aid bacterial adhesion, invasion, survival, and immune evasion (OmpA,
Although with limitations that are being addressed[148], native and bio-engineered EVs derived from pathogenic bacteria are also potential sources of vaccines [2,4,42]. For instance, vaccination against tumors and intracellular viruses has been demonstrated using EVs derived from hypervesiculating Salmonella typhimurium that induce antigen-specific CD8+ T cell responses[149]. Similarly, EVs from S. aureus induce specific humoral and cellular immune responses. Mice vaccinated with S. aureus-derived EVs were protected from pneumonia and mortality induced by the administration of sublethal and lethal doses of
Cargo packaging in BEVs
This review will introduce some fundamental concepts of cargo loading in BEVs, and a detailed discussion on the mechanisms is not attempted here but can be seen in references[153-161].
Proteins: The biological functions of EVs are determined by their protein cargo[153]. Studies have examined how BEV cargo is packaged using several strains of S. aureus to generate ideas for new interventions against pathogenic bacteria and develop drug delivery systems[153]. In S. aureus, it was shown that there is a selective cargo sorting process in BEVs[153]. The packaging of proteins in EVs was driven by abundance, charge, and subcellular localization[153,162-164]. Though more investigation is required, it is believed that EV cargo also contains components such as chaperones and protein secretion systems for the selection of proteins into EVs[153].
This is reminiscent of a previous report that OMVs strictly sort their protein cargos by the special signal sequences (signal peptides) in the amino acid sequences[23,154]. The signal peptides guide proteins to their target vesicles which must possess the corresponding receptor on their membranes to recognize the signal peptides[23,155,156,158]. BEVs can also internalize foreign proteins via fusion to P49 protein expressed in Shewanella vesiculosa HM13 strain[157].
Selective cargo sorting into BEVs also includes outer membrane lipid chaperones. Outer membrane lipid chaperones enriched with LPS carrying negatively charged O antigen chains (A-LPS) may play a critical role in selective cargo packaging due to their affinity for the overall charge[159,165]. This increased the selective packaging of virulence factors such as gingipains into Porphyromonas gingivalis OMVs[159]. Proteins associated with charged LPS are packaged in OMVs, while those associated with neutral LPS (O-LPS) are localized in the outer membrane[158,159,161]. Similarly, the lipid composition of Streptococcus pyrogens membrane was responsible for the selective enrichment of specific proteins and RNA species[160].
Nucleic acids: Although the mechanism by which nucleic acids are packaged by OMVs remains unclear, DNA, mRNA, miRNA, and other non-coding RNAs may enter OMVs through a similar recognition of corresponding sequences[166,167]. EVs carry DNA both on the membrane surface and in the lumen, with most DNA located on the external surface of OMV[145]. Different forms of luminal DNA have been identified in OMVs secreted by E. coli, Neisseria gonorrhoeae, Pseudomonas aeruginosa, and Haemophilus influenzae. The luminal DNA retains its antigenicity even after treatment of the vesicle with DNase[168].
Lipids: The lipid composition of Gram-negative and Gram-positive BEV are different. The most common lipids in Gram-negative BEV are phosphoglycerolipids. Other lipids found in Gram-negative BEV include lipoproteins, LPS, glycerolipids, and phospholipids. In Gram-positive bacteria such as S. pyrogens, the BEVs are abundant in phosphatidylglycerol and lack cardiolipin[160].
Cargo exchange between human and bacterial EVs
BEVs facilitate bacteria-host and bacteria-bacteria interactions with their cargos[25,131] [Figure 1] that also deliver toxins and virulence factors into host cells[131,169].
Uptake of BEVs by human (host) cells
After adhesion/binding of BEVs to host cells, non-phagocytic host cells internalize BEVs through the following mechanisms[129,131,170]: (1) macropinocytosis; (2) clathrin-mediated endocytosis; (3)
Figure 2. Mechanisms of uptake of bacterial extracellular vesicles by human (host) cells. BEVs enter human cells by macropinocytosis/phagocytosis, clathrin- and caveolin-mediated endocytosis, lipid rafts, and direct fusion with the plasma membrane. ER: endoplasmic reticulum; MVB: multivesicular bodies; GL: glycolipid; GPI: GPI-anchored proteins; PL: phospholipid; RTK: receptor tyrosine kinases; SL: sphingolipid. Created with BioRender.com.
Mechanisms of BEV uptake by human (host) cells
Mechanism/receptors | Cells | Bacterial species |
Macropinocytosis/phagocytosis (actin-dependent) | ||
Macrophages | P. aeruginosa[171] | |
N-WASP | Respiratory tract epithelial cells | P. aeruginosa[172] |
Rac-1 and Cdc42 | Cervical and gingival epithelial cells | P. gingivalis[173] |
Clathrin-mediated endocytosis | ||
VacA | Gastric epithelial cells | H. pylori[174,175] |
Dynamin | Colorectal epithelial cells | EHEC[176] |
Dynamin | Intestinal epithelial cells | E. coli[177] |
Cervical epithelial cells and monocytes | B. abortus[178] | |
Caveolin-mediated endocytosis | ||
Caveolin 1 | Pharyngeal epithelial cells | H. influenza[179] |
CT-receptor | Intestinal epithelial cells | V. cholerae[180] |
Caveolin | Adrenal and intestinal epithelial cells | ETEC[181] |
Lipid raft-mediated endocytosis | ||
PaAP | Lung epithelial cells | P. aeruginosa[182] |
Intestinal epithelial cells | C. jejuni[183] | |
Dynamin-independent | Intestinal epithelial cells | V. cholerae[184] |
Dynamin-dependent | Gastric epithelial cells | H. pylori[174,185] |
TLR2 | Alveolar epithelial cells | M. catarrhalis[186] |
IgD BCR, TLR9 and TLR2 | Tonsillar B cells | M. catarrhalis[187] |
Cervical and gingival epithelial cells | P. gingivalis[173] | |
Cervical epithelial cells | A. baumannii[188] | |
Direct membrane fusion | ||
Respiratory tract epithelial cells | P. aeruginosa[172] | |
Cervical epithelial cells and gingival fibroblasts | A. actinomycetemcomitans[189,190] | |
Macrophages | L. pneumophilia[191] |
Actin-dependent macropinocytosis: This is similar to phagocytosis but does not require direct contact with the internalized material[192]. It involves the rearrangement (polymerization) of actin filaments to form a ring under the cell membrane, which eventually envelopes a portion of the extracellular space by closing at the top[131,193]. Actin polymerization is driven by the receptor tyrosine kinases (RTKs) activation via Rac and Rho GTPases. The largest endocytic vesicles (> 1 μm) are produced by an actin-dependent pathway[194], which could be N-WASP-mediated as in Pseudomonas aeruginosa[172] or Rac1-regulated pinocytic pathway employed by Porphyromonas gingivalis OMVs that is independent of clathrin, dynamin, and caveolin[173]
Clathrin-dependent endocytosis (CDE): This is the major vesicular trafficking pathway from the cell surface to the interior in mammalian cells[195,196]. Clathrin-coated pits that mature into clathrin-coated endocytic vesicles are assembled following the binding of a ligand (BEV) to a cell surface receptor[195,197,198]. CDE also requires adaptor protein complexes, a variety of endocytic accessory proteins, and phosphatidylinositol lipids[195,196] and permits uptake of BEVs with a maximum size of 120 nm diameter. Using inhibitors of clathrin pit formation (Chlorpromazine) and dynamin (dynasore), this pathway was reported to be the preferred route of entry by OMVs from different strains of E. coli, Helicobacter pylori, and Brucella abortus, as well as free cytotoxic virulence factors including cholera and Shiga toxins and VacA of H. pylori[131,175,177,178,199-201].
Caveolin-mediated endocytosis: Membrane invaginations (caveolae) are formed and internalized in a dynamin-dependent fashion[192]. The formation of caveolae is due to the enrichment of membrane lipid-raft domains with caveolin, cholesterol, and sphingolipids. Smaller OMVs (20-100 nm) from E. coli, H. pylori, Moraxella catarrhalis, Vibro cholerae, and Haemophilus influenzae are preferentially taken up by host cells via caveolin-mediated endocytosis[179,180,186,202,203]. The cholera toxin from V. cholerae and heat-labile enterotoxin LT1 contained in E. coli-derived vesicles bind to the glycosphingolipid GM1 that is found on membrane lipid rafts enriched with caveolin, facilitating their uptake via caveolin-enriched endocytic vesicles[180,203]. This mechanism is preferred by E. coli, P. aeruginosa, Campylobacter jejuni, V. cholerae, S. typhimurium, H. influenzae and some viruses[204], as the pathogens avoid trafficking to lysosomes and subsequent degradation when they are internalized via caveolae compared to clathrin-coated membrane invaginations[170]. Although caveolin-mediated endocytosis is five times slower than the clathrin-mediated pathway, it facilitates the efficient delivery of cargo to the cytosol[192,198,205].
Lipid raft-mediated endocytosis: Lipid rafts are highly organized and rigid cholesterol and
Direct membrane fusion: As demonstrated for P. aeruginosa, A. actinomycetemcomitans, and Legionella pneumophila, OMVs also enter host cells by direct fusion with the host cell plasma membrane often at lipid raft domains[172,189,191] [Figure 2 and Table 2].
The mechanism of uptake of BEVs by host cells is dependent on their size, content, biogenesis, bacterial growth rate, and the fate of vesicles after uptake, even when derived from the same bacterial species. Specific protein or lipid cargos of BEVs could guide them to a specific uptake route[131,192]. This advocates cell-specific EV uptake[192]. The route of uptake of BEVs, in turn, determines the delivery and fate of the vesicles and their cargo[131].
Uptake of human EVs by bacterial cells
The mucosae of various human body sites harbor trillions of different bacterial strains that form respective microbiota, of which the oral, gut, and vaginal microbiotas are quintessential examples[2,20-22,207,208]. Bacteria present in these microbiotas produce BEVs involved in bacteria-bacteria and bacteria-host interactions[2,134,207] that promote health or propagate infections[209]. For instance, HIV-1 bound to the OMVs of P. gingivalis (an oral bacteria) was able to enter nonpermissive human oral keratinocytes (HOK) cells via vesicle endocytosis [Figure 2] and cause infection[210]. On the other hand, BEVs from commensal intestinal bacteria - Enterobacter cloacae and Bacteroides thetaiotaomicron-reduced replication of norovirus during coinfection of RAW 264.7 macrophages by inducing the gene expression of IL-1β, IL-6, TNF-α, and
Although the mechanisms of uptake of BEVs by human host cells have been proposed as described above [Figure 2], whether the same mechanisms apply to the uptake of human EVs by bacterial cells remains unclear. Even BEV interactions with bacterial cells appear confined to their cargo, with vague data on the uptake of BEVs by bacterial cells. Meanwhile, we can speculate that bacterial cells may package human and BEVs and/or their cargo during host-bacteria and bacteria-bacteria interactions in various body microbiomes or sites distant from the microbiomes, including the feto-maternal interface. BEV-mediated bacteria-bacteria interaction is displayed succinctly in quorum sensing, antibiotic resistance, biofilm formation, and survival[2]. For example, BEV-mediated antibiotic resistance is exhibited by Bacteroides spp., a dominant genus in the gut and vaginal microbiota that is associated with bacterial vaginosis (BV) and premature birth[20-22,212]. Bacteroides BEVs contain cephalosporinases that hydrolyze the β-lactam rings in antibiotics, thereby protecting both pathogens and commensal bacteria from antibiotics of the β-lactam type[213].
Recently, our group isolated and characterized BEVs from human placental tissues[26]. We posited that the BEVs probably reached the placenta through hematogenous spread from various maternal body sites harboring microbiomes. Furthermore, their presence may trigger a low-grade localized inflammation to prime an antigen response in the placenta. However, it is insufficient to cause an overt fetal inflammatory response that can lead to adverse pregnancy events[26]. Nevertheless, whether the BEVs contained human EV cargo and vice versa was not determined.
It is known that human EVs can take up free or EV-associated bacterial products, and BEVs may exchange their cargo with human cells or EVs. As the vagina is the most common route of bacterial colonization of the choriodecidual membranes and amniotic cavity[214], it is pertinent for researchers to investigate whether part of the mechanisms of infectious and inflammatory adverse reproductive outcomes is mediated by human cells/EVs carrying bacterial products from the vagina or bacterial cells/EVs carrying human cargo that help them evade immune clearance.
BEV-induced inflammatory responses
During bacterial colonization of tissues, either as microbiota or infection, BEVs may be shed directly by pathogens or EVs by pathogen-infected cells[215]. BEVs carry microbial- or pathogen-associated molecular patterns (MAMPs/PAMPs) that can be recognized by pattern recognition receptors (PRRs) on the surface and in the cytoplasm of immune and non-immune cells[14,216,217] [Figure 3]. The interaction of these
Figure 3. Bacterial extracellular vesicle (BEV) pathogen-associated molecular patterns (PAMPs) recognize surface membrane and cytoplasmic host cell pattern recognition receptors and activate downstream inflammatory signaling pathways. Omp, LTA, and porin only bind to TLRs on the cell membrane surface. At the same time, both surface membrane and cytoplasmic receptors recognize the other PAMPs. AIM2: absent in melanoma 2; IRF: interferon regulatory transcription factor; LPS: lipopolysaccharide; LTA: lipoteichoic acid; MAPK: mitogen-associated protein kinase; NF-κB: nuclear factor kappa B; NLR: nucleotide-binding oligomerization domain-like receptor; NLRP: NOD-like receptor thermal protein domain-associated protein;Omp: outer membrane protein; PGN: peptidoglycan; STING: stimulator of interferon genes; TLR: toll-like receptor. Created with BioRender.com.
For example, LPS, adhesins, and other virulence factors in the periodontal bacterium Fusobacterium nucleatum-derived EVs interact with Toll-like receptor (TLR)-4 and induce the expression of IL-8 and TNF-α in patients with inflammatory bowel disease (IBD)[219]. In contrast, M. tuberculosis and M. bovis EVs stimulate the release of IL-1β, IL-6, IL-10, IL-12, TNF, CXCL1, and MIP-1α in macrophages through TLR2 signaling[220,221]. LPS, lipoproteins, flagellin, and DNA carried by OMVs can interact with TLRs on microglia and macrophages to induce the release of TNF-α and IL-10[222,223]. DNA, RNA, and peptidoglycan cargo in
BEV-induced inflammatory responses at the feto-maternal interface (placental tissues), resulting in adverse pregnancy outcomes
Intra-amniotic injections of Group B Streptococcus (GBS) EVs promoted the upregulation of
Microbial vesicles and their potential contributions during pregnancy
The controversy surrounding the presence of a microbiome in the placenta is somewhat mitigated, and the consensus has emerged that the intrauterine environment is rather sterile. However, our reporting of BEVs in the placenta[26] and a recent report that amniotic fluid also contains BEVs[227,228] suggest that amplification of microbial nucleic acid, and identification of microbial antigens and other cellular fragments are more likely the confirmation of microbial vesicles than the presence of microbe itself. Amplification of placental BEVs and lack of any microbiome beyond noise levels expected from procedural aspects of experiments confirm that placental microbiome is a mistaken identity. The world of microbial vesicles in a sterile environment of pregnancy opens a plethora of questions and may answer several mysteries of pregnancy maintenance, immune homeostasis, microbiome formation in the fetus, and fetal immune privileges to commensal bacteria in utero, at birth, and during the early developmental stages.
Maternal gut microbiota-derived EVs can cross the placental barrier
Although the role of EVs in human pregnancy has been reviewed extensively[35,36,229,230], data on microbial EVs in healthy pregnancies are still limited. However, maternal gut microbiota-derived EVs can cross the placental barrier to reach the fetus[227]. This EV-mediated in utero communication between the fetus and the mother was evidenced by the presence of bacterial RNA and proteins in the amniotic fluid of pregnant women[227]. The EVs derived from both compartments shared similar bacterial composition and protein cargo. This EV-mediated interaction may be required for priming the fetal immune system, which is essential for neonatal gut colonization[227]. A mouse model also reproduced these findings[227].
This report is an extension of our previous study that identified BEVs in placental tissues[26] and the findings of Nunzi et al., who reported the presence of BEVs in human amniotic fluid via 16S-rRNA gene sequencing[228]. Furthermore, our study[26] and that of Kaisanlahti et al. opined that the presence of BEVs in placental tissues or fetal compartments, irrespective of the source, is crucial for fetal immune priming, perhaps through low-grade immune stimulation[227]. The point at which this ‘beneficial’ immune stimulation becomes deleterious and the mechanism(s) that drive such phenotype require further investigation. Future years will generate evidence that BEVs have a significant role in priming human immune system development, and immune system development starts in utero and not during the early stages of development after birth in response to environmental microbial exposures.
EV-DNA and its role in placental inflammatory response
EV-DNA is considered a mediator of cellular homeostasis[231] as well as innate and adaptive immune responses[79,232]. Cellular excretion of potentially harmful damaged DNA prevents the induction of apoptosis and promotes survival[231]. Active release of EV-DNA, as indicated by increased concentrations of circulating DNA, correlates with the proportion of cells preparing for division (G1 phase)[233].
Although whether BEVs and human EVs found at the feto-maternal interface exchange cargos has not been established, we hypothesize that human cells in this compartment can take up BEVs. In contrast, BEVs can internalize human products such as DNA, RNA, proteins, metabolites, and lipids. For instance, the human cells may internalize BEVs, or human EVs may bind to LPS released by BEVs, presenting it to membrane PRRs or facilitating its access to the cytosol, where it can trigger an inflammatory response[234] [Figure 4]. Uptake of G. vaginalis BEVs by vaginal epithelial cells leads to vaginolysin-mediated cytotoxicity and increased release of IL-8[235,236].
Figure 4. DNA-driven immune response mediated by DNA exchange between human and bacterial extracellular vesicles. Human/bacterial genomic/mitochondrial DNA carried by EVs can be delivered to cells as damage- (DAMP) or pathogen-associated molecular pattern (PAMP). EV-DNA can activate inflammatory pathways through cytosolic DNA receptors such as cGAS (cyclic GMP-AMP) and AIM2 (absent in melanoma 2 or interferon-inducible protein). Activation of the cGAS/STING pathway causes downstream release of type I interferons (IFN-α, IFN-β, IFN-ε). Activation of AIM2 produces interleukins and tumor necrosis factor (TNF). This may manifest as a subclinical sterile inflammatory response in gestational tissues such as the placenta and amniochorion. bDNA: bacterial DNA; BEV: bacterial extracellular vesicles; cfDNA: cell-free DNA; DNA: human DNA; HEV: human extracellular vesicles; IRF: interferon regulatory factor; mtDNA: mitochondrial DNA; MVB: multivesicular bodies; NF-κB: nuclear factor-kappa B; STING: stimulator of interferon genes. Created with BioRender.com.
There could also be HGT between EVs from both species or between EVs and host cell genomic and mtDNA[105]. OMVs can carry DNA into human cells and exchange it with the human DNA via HGT[145]. The altered/damaged DNA in EV and cfDNA (from dead cells) that stick to the surface of EVs could subsequently be taken up by a recipient cell in an autocrine or paracrine manner. The DNA can trigger several cytosolic DNA receptors, including cyclic GMP-AMP synthase (cGAS), leading to the downstream release of type 1 IFN through activation of stimulator of interferon genes (STING) and translocation of
Placenta inflammatory response
It is plausible that the “sterile” inflammation that leads to adverse birth outcomes[241,242] may be a product of chronic inflammatory response to damaged/altered EV-DNA or other PAMPs/DAMPS (LPS, Hsp70, HMGB1) carried by human/BEVs[13,30,69,77,243-250], and delivered to the placenta. cfDNA can stick to EVs, be internalized by cells, and induce several inflammatory responses mediated by TLRs and other nucleic acid receptors through the mechanisms described in Figures 3 and 4[106,251].
We postulate that in gestational tissues such as the placenta and amniochorion, EV-DNA from pathogenic bacteria (due to maternal infections such as periodontitis, BV, urinary tract infection, sexually transmitted diseases during pregnancy) can be delivered to the cytosol and activate the cGAS/STING and AIM2 signaling pathways to release type I IFN and interleukins [Figures 3 and 4]. Because there may be no clinical infection by any pathogen, this may manifest as subclinical sterile inflammation that can induce adverse reproductive outcomes.
Placental inflammatory responses that underpin maternal-fetal allograft rejection, preeclampsia, intrauterine growth restriction (IUGR), and preterm birth are induced by infectious and sterile stimuli[242]. However, sterile intra-amniotic inflammation is more common than microbial-associated intra-amniotic inflammation[250,252-254]. Chronic placental inflammatory lesions are mediated by cytotoxic T cells and IFN-γ-inducible CXCR3 ligands[255]. Upon antigenic stimulation, T cells can secrete EVs carrying both gDNA and mtDNA that trigger IFN1 response from DCs via the cGAS/STING pathway. This primes the DCs against subsequent viral infections[232]. T cells’ local EV-DNA inflammatory activities can be explored further in the context of immune tolerance surveillance at the feto-maternal interface.
A considerable proportion of pregnant women with inflammation of gestational tissues, which eventually deliver prematurely, do not have an infection[254,256,257]. There can be innumerable reasons for a sterile inflammatory condition associated with premature delivery; however, recent findings on BEVs support the concept that maternal morbid or behavioral factors that may be linked to adverse events, may also be compounded by pathogenic microbial EVs, or subclinical infection in mothers may deliver BEVs to facilitate a sterile inflammatory condition. This sterile inflammation often mimics infectious inflammation seen during adverse pregnancies. In these instances, microbial culture or other molecular diagnostic approaches may render negative reports and mismanagement of a patient with a subclinical infection that sheds BEVs loaded with immunogenic factors. Therefore, the possible role of EV-DNA, as well as other alarmins carried by EVs, in chronic inflammation of gestational tissues that lead to adverse outcomes requires more investigation. Moreover, the threshold at which BEVs' proposed immune priming role in gestational tissues such as the placenta and fetal membranes changes to an overt labor-inducing inflammatory response needs to be determined along with the underpinning mechanisms. EV-DNA could be used for priming the immune system to defend against subsequent infection[108]. However, this is yet to be demonstrated in the fetus in utero.
Benefits and disadvantages of EV-mediated responses at the feto-maternal interface (placental tissues)
Priming of the fetal immune system
Commensal bacteria such as lactobacilli can produce BEVs that stimulate innate immune responses to control infection by pathogens. This immunostimulation may prime the host cells to mount an adequate immune response against foreign invaders upon subsequent exposure[209]. However, in uncomplicated pregnancies, the most prevalent BEVs resident in the placenta and fetal membranes and their origins
Figure 5. Extracellular vesicle-mediated feto-maternal immune tolerance. The feto-maternal immune tolerance that maintains normal pregnancy is partly mediated by BEVs from various microbiotas. Maternal BEVs can also carry DAMPs/PAMPs to the feto-maternal interface (placenta tissues) and trigger adverse immune responses that may manifest as feto-maternal allograft rejection in the form of graft-versus-host disease (GVHD). However, the threshold at which such a switch occurs, and the mechanisms involved are yet to be determined. Conversely, pathogenic bacteria can shed BEVs that can cause inflammatory responses often associated with adverse pregnancy conditions. BEV: bacterial extracellular vesicle; DAMPs/PAMPs: damage- and pathogen-associated molecular patterns; FM: feto-maternal; HEV: human extracellular vesicle. Created with BioRender.com.
Furthermore, the Ureaplasma DNA load seen in amniotic fluid[268-278] may have been transported by EVs, and this may not necessarily indicate pathogenesis. However, molecular diagnostic strategies such as polymerase chain reaction and 16S gene sequencing employed in those studies may have omitted bacterial DNA packaged in EVs with pathogenic or immune priming potentials. If Ureaplasma spp., G. vaginalis, and GBS can find their way to the amniotic fluid, they may continue to shed EVs to enhance inflammatory pathologies. So, even if the bacterial load is less, thousands of BEVs shed can cause damage and may be resistant to antimicrobial treatment. Therefore, controlling inflammation becomes of utmost importance in reducing adverse incidences compared to mere antibiotic treatment. Additionally, because the most prevalent BEVs in gestational tissues at a given time point may not be of vaginal origin, the investigation needs to be expanded to BEVs from other niches in the body and external environment.
Suppose the immune priming action of placental BEVs is confirmed. In that case, such BEVs can be explored for vaccine development and therapeutic purposes[279,280] [Table 3] to either stimulate or suppress feto-maternal immune responses against intrauterine infection or microbial invasion of the amniotic cavity that cause preterm premature rupture of membranes (PPROM) and preterm labor[258,265-267,285]. This is because, rather than aiding pathogenesis, exosomes and OMVs are potent immune modulators[286], as demonstrated in pertussis (whooping cough) caused by Bordetella pertussis[287]. Furthermore, S. aureus EVs engineered to serve as vaccine candidates elicited adaptive immune response and conferred protection against lethal sepsis caused by S. aureus in mice[133]. Additionally, S. pneumoniae-derived EVs incubated with murine DCs were rapidly internalized and promoted the release of TNF-α, which constitutes the inflammatory response[288].
Summary of usage and applications of bacterial and human extracellular vesicles and future aspects for exploration
Use/application | Extracellular vesicle | Feature/summary of use | References |
Immune priming | BEV | ● Antigen-presenting and immune stimulation ● Recognized as self-antigen | [26,227,228] |
HEV | ● EVs from APCs carry p-MHC-II and costimulatory signals, and directly present the peptide antigen to specific T cells to induce their activation ● Placental EVs mediate immune tolerance during gestation | [230,281] | |
Vaccine and adjuvant development | BEV | ● Present multiple antigens simultaneously in a native state to elicit effective immune responses ● Stimulate immune responses against bacterial and viral infections ● Examples: BEVs from N. meningitidis, V. cholerae, B. pertussis, S. aureus, S. pneumoniae, C. perfringes, B. anthracis, etc. ● Engineered OMVs as adjuvant-free vaccine platform for important pathogens | [2,4,25,209,282,279,280,151] |
HEV | ● EVs from macrophages and DCs against M. tuberculosis and T. gondii infections ● EVs are also used to modulate immune response against tumor development | [44] | |
Therapeutics and drug delivery | BEV | ● Carry and deliver several bioactive molecules ● Enter distant organs from the circulation ● Non-replicating and great biostability ● Easy to modify by electroporation ● Treatment of gut, brain, bone diseases and cancers | [209,216,283] |
HEV | ● EVs can be packed with small molecule drugs to evade immune surveillance and delivered intact and directly to the target tissue ● MSC-derived EVs promote wound healing via Wnt-β catenin pathways and used in the treatment of cardiovascular, lung, renal, and liver diseases, GVHD, etc. | [44,281,284] | |
Biomarkers | BEV | ● Readily found in body fluids ● Diagnosis of bacterial infections, lung disease, bone disease, colorectal cancer, and pancreatic adenocarcinoma | [25,283] |
HEV | ● Widely distributed and more readily available through liquid biopsies using blood, saliva, urine, breast milk, sperm, CSF, and vaginal fluid ● Contain DNA, miRNA, and protein biomarkers ● Applied in the diagnosis of cancer, asthma, COPD, GVHD, COVID-19 infection, etc. | [44,105,106,108,281] | |
Future aspects for exploration | BEV | ● Address which receptors determine the uptake of BEVs by host cells ● Mechanism of cargo packaging ● Address the challenge of LPS-associated biosafety when Gram-negative BEVs are used as vaccines or drug delivery vehicles ● Gram-positive BEVs may be a better choice for drug delivery ● Identify a faster, cost-effective and efficient (high yield) isolation method from a wide range of biological specimens ● Identify unique (surface) markers present on BEVs from different sources | [2,283] |
Placenta and fetal membranes: ● Identify the most prevalent BEVs ● Targeting BEVs specifically to these tissues ● Do BEVs in these tissues differ between term vs. preterm deliveries or PE vs. normotensive pregnancies? ● Do BEVs from placenta elicit similar immune responses in other gestational tissues ● Do BEVs from BV/PTB-associated bacteria elicit inflammation in gestational tissues? | |||
HEV | ● Identify mechanisms responsible for the uptake of EVs containing DNA ● Identify mechanisms for DNA packaging in EVs ● Standardization of EV-DNA isolation and analysis techniques ● Resolution of differences in EV-DNA distribution, localization, and structure for diagnostic and functional studies | ||
Placenta and fetal membranes: ● Are there human cells/EVs containing bacterial EVs or products in gestational tissues? ● Do bacterial cells contain human EVs in gestational tissues? |
Stimulation of preeclampsia, preterm labor and birth
The proposed priming action of BEVs in the feto-maternal interface is adjudged to be mediated by a low-grade inflammatory stimulation insufficient to induce labor[26]. Pregnancy is characterized by low-grade systemic inflammation and immune tolerance that allow implantation and placentation[255,289]. Disruption of this physiologic inflammatory state could lead to a breakdown in fetal-maternal tolerance and uncontrolled pro-inflammatory responses that trigger labor prematurely or other undesired reproductive outcomes such as preeclampsia and IUGR[255,290]. For instance, intraperitoneal injection of fetal DNA or CpG, which eukaryotic/BEVs can transport to uterine tissues or feto-maternal interface [Figures 3-5], induced TLR-9, STING, and NF-κB-mediated inflammatory release of IL-6, leading to fetal resorption, preeclampsia, preterm labor, and preterm birth in mice with additional immune impairment[291-294]. The BEV-associated immune stimulation must remain optimal throughout gestation, or an overt EV-mediated inflammatory response with deleterious effects may ensue without any identified pathogen [Figures 4 and 5].
What is yet unknown is the threshold at which preeclampsia- or labor-associated pro-inflammatory responses can be triggered by BEVs in placental tissues. We speculate that, like an increase in bacterial load or altered microbiota, an increase in placental BEVs and their virulence factors carried as cargos beyond the tolerable/immune priming threshold may induce an overt inflammatory response that could lead to premature expulsion of the fetus or pregnancy complications such as preeclampsia and IUGR or neonatal complications[230]. Such immune response could manifest as acute placental inflammation (i.e., maternal and fetal inflammatory responses)[242,295-298] or chronic placental inflammation[255,299]. If determined, this will improve our understanding of the concept of "sterile inflammation", which could be induced by
Novel preeclampsia or preterm birth-associated inflammatory biomarker targets and preventive/therapeutic interventions
EV-DNA has been speculated to be more beneficial than plasma cfDNA in cancer diagnosis using certain liquid biopsies[302-304]. Elevated serum BEV IgG antibody-based asthma and chronic obstructive pulmonary disease diagnosis have also been tested[137]. Additionally, metagenomic and metabolomic profiling of stool BEVs revealed significantly altered Firmicutes and Proteobacteria as well as amino acids, carboxylic acids, and short-chain to long-chain fatty acids metabolism in colorectal cancer patients[305]. Similarly, human EVs and BEVs found in placental tissues can facilitate the identification of new preeclampsia or preterm
Meanwhile, human EV-derived inflammatory proteins, miRNAs, and lipids that are associated with placental dysfunction, preterm labor, and birth have also been identified in maternal plasma across different gestational time points[35,306,307]. Moreover, after vaginal infection with E. coli, intravenous IL-10 encapsulated in exosomes delayed preterm birth by reducing feto-maternal uterine immune cell inflammation[308]. These reports indicate great promise for the utility of EVs as early predictors for preterm birth and candidates for safe and efficient drug delivery to the placenta and fetal membranes [Table 3]. However, these reports are primarily on human EVs, whereas data on BEVs are still minimal.
It is plausible that the drivers of placental inflammatory responses could be culpable for the disruptive phenotypes. This is because, even in the absence of clinical infection, PAMPs/DAMPs carried by and exchanged between human and BEVs can be transferred to placental tissues, gain access to the amniotic cavity, and trigger fetal inflammatory response, leading to maternal anti-fetal rejection[242,255]. For example, B. fragilis OMVs (without the bacteria) activated a broader range of host innate immune receptors (TLR2, TLR4, LR7, and NOD) compared to their parent bacteria (TLR2 alone) due to their enrichment with RNA and peptidoglycan cargo and their ability to transport this cargo directly into host epithelial cells[309]. Though this was demonstrated in intestinal epithelial cells, B. fragilis is a bona fide member of the vaginal microbiota as well[21,22]. Therefore, it is possible for OMVs released by this species to transport their cargo to the placenta, either hematogenous or through the vagina, and stimulate what may seem like a sterile inflammatory response.
The EVs and their inflammatory cargo could also emanate from the fetal cells and be delivered to maternal gestational tissues[310]. So, there could be bidirectional trafficking of inflammatory human EVs (and possibly BEVs) between the mother and fetus[29,30,230]. This is a precursor for inflammation-associated placental dysfunction, preeclampsia, fetal growth restriction, spontaneous preterm labor and postnatal developmental impairments[35,230,311-314].
S. aureus-derived BEVs that contain proteins involved in metal ion acquisition may compete with bacterial and host cells, depriving them of such nutrients and thereby inhibiting their growth and survival[153]. This may be particularly beneficial in preventing the colonization of gestational tissues by BV and preterm
Consequently, the health-promoting low-grade inflammation induced by lactobacilli in the lower genital tract can be replicated in the feto-maternal interface using
CONCLUDING REMARKS AND FUTURE PERSPECTIVES
As discussed in this review, host-bacteria interaction is facilitated by BEVs and not only through direct contact between human and bacterial cells. The EVs are released by both bacterial and human cells and transfer bioactive molecules that influence the activity of the recipient cells, which may be distant from the producing cells. BEVs package multiple PAMPs that mimic and sometimes surpass the immunogenic properties of the secreting bacteria[42,320]. That is, direct colonization of tissues by bacterial cells is not required for immunogenic stimulation. This phenomenon is important in the feto-maternal interface, where optimum tolerance between the mother and fetus is required until delivery at term (37-40 weeks). Though the sterility of the placenta is still debatable, BEVs from diverse sources have been identified in this tissue[26] and the amniotic cavity[227]. These BEVs can be internalized by human cells, which may help them evade host immune clearance. Though it appears logical, whether bacterial cells internalize human EVs is yet to be determined. However, the presence of BEVs in placental tissues or the amniotic cavity is believed to trigger a low-grade immune response that primes the fetal immune system for ex-utero survival but is insufficient to disrupt the progression of pregnancy. This appears to be another mechanism that propagates feto-maternal immune tolerance, as observed in BEV-mediated maintenance of intestinal immune homeostasis[216,321]. However, because some pregnant women still experience inflammation-associated diseases such as preeclampsia and preterm birth without clinical infection, the point or dose at which the BEV-mediated low-grade inflammation develops into a suboptimal immunological response capable of disrupting the typical sequence of pregnancy needs to be elucidated. Furthermore, the mechanisms that drive such feto-maternal intolerance should enhance our understanding of EV-associated pregnancy complications.
On the beneficial side, BEV vaccines exhibit immunostimulatory efficiency akin to that of the inactivated whole-cell vaccine, stimulating both cell-mediated and humoral immune responses in animals[150,322,323]. BEV vaccines have also been applied to tackle meningococcal group B disease in humans[324]. OMV vaccines or adjuvants could be targeted at the placenta to prime the fetus against future exposure to harmful
Moreover, as demonstrated in the administration of Ligilactobacillus animalis, Akkermancia muciniphila, Lactobacillus plantarum, and Proteus mirabilis BEVs to treat bone disease, glucose intolerance, obesity, IBD, and stress-induced depression-like behaviors[325-329], native/natural BEVs from commensal or probiotic Gram-positive bacteria such as lactobacilli (to avoid LPS action) could serve as potential therapeutic candidates to promote a eubiotic and anti-inflammatory state in the feto-maternal interface while keeping the intrauterine environment “sterile”. This requires a comprehensive omic analysis of lactobacilli-derived EVs in comparison with EVs from common vaginal pathogens. We could also determine whether placental BEVs differ between women who deliver at term without labor and those who deliver preterm.
In summary, the exchange of cargo between human and BEVs and the isolation of BEVs in placental tissues and amniotic cavity have revealed new perspectives on the pathologic mechanisms of inflammatory pregnancy complications. The presence of BEVs in the placenta primes the fetal immune system. BEVs in gestational tissues appear to be good candidates for predicting adverse reproductive outcomes, as well as for vaccine development and drug delivery during gestation. Future studies should determine whether bacterial cells take up human EVs, the diagnostic potential of placental BEVs, and the mechanistic link between placental BEVs and pregnancy complications such as preeclampsia and preterm labor and birth.
DECLARATIONS
Acknowledgments
The authors would like to thank the members of the Menon lab for their feedback during the conceptualization of the study.
Author’s contribution
Conceived and designed the concepts for this review: Menon R, Amabebe E
Performed the literature search and produced the first draft of the manuscript: Amabebe E
Reviewed and edited the manuscript: Amabebe E, Kumar A, Tatiparthy M, Kammala AK, Taylor BD, Menon R
Read and approved the final manuscript for submission: Amabebe E, Kumar A, Tatiparthy M, Kammala AK, Taylor BD, Menon R
Availability of data and materials
Not applicable.
Financial support and sponsorship
Not applicable.
Conflicts of interest
All authors declared that there are no conflicts of interest.
Ethical approval and consent to participate
Not applicable.
Consent for publication
Not applicable.
Copyright
© The Author(s) 2024.
REFERENCES
1. Gill S, Catchpole R, Forterre P. Extracellular membrane vesicles in the three domains of life and beyond. FEMS Microbiol Rev 2019;43:273-303.
2. Ñahui Palomino RA, Vanpouille C, Costantini PE, Margolis L. Microbiota-host communications: bacterial extracellular vesicles as a common language. PLoS Pathog 2021;17:e1009508.
3. Welsh JA, Goberdhan DCI, O'Driscoll L, et al. MISEV Consortium. Minimal information for studies of extracellular vesicles (MISEV2023): from basic to advanced approaches. J Extracell Vesicles 2024;13:e12404.
4. Bose S, Aggarwal S, Singh DV, Acharya N. Extracellular vesicles: an emerging platform in gram-positive bacteria. Microb Cell 2020;7:312-22.
5. Sáez T, de Vos P, Sobrevia L, Faas MM. Is there a role for exosomes in foetoplacental endothelial dysfunction in gestational diabetes mellitus? Placenta 2018;61:48-54.
6. Kalra H, Drummen GP, Mathivanan S. Focus on extracellular vesicles: introducing the next small big thing. Int J Mol Sci 2016;17:170.
7. Manier S, Liu CJ, Avet-Loiseau H, et al. Prognostic role of circulating exosomal miRNAs in multiple myeloma. Blood 2017;129:2429-36.
8. Lee YT, Tran BV, Wang JJ, et al. The role of extracellular vesicles in disease progression and detection of hepatocellular carcinoma. Cancers 2021;13:3076.
9. Pezzana C, Agnely F, Bochot A, Siepmann J, Menasché P. Extracellular vesicles and biomaterial design: new therapies for cardiac repair. Trends Mol Med 2021;27:231-47.
10. Ge Q, Zhou Y, Lu J, Bai Y, Xie X, Lu Z. miRNA in plasma exosome is stable under different storage conditions. Molecules 2014;19:1568-75.
11. Maas SLN, Breakefield XO, Weaver AM. Extracellular vesicles: unique intercellular delivery vehicles. Trends Cell Biol 2017;27:172-88.
12. Liu YJ, Wang C. A review of the regulatory mechanisms of extracellular vesicles-mediated intercellular communication. Cell Commun Signal 2023;21:77.
13. Buzas EI. The roles of extracellular vesicles in the immune system. Nat Rev Immunol 2023;23:236-50.
14. Pitt JM, Kroemer G, Zitvogel L. Extracellular vesicles: masters of intercellular communication and potential clinical interventions. J Clin Invest 2016;126:1139-43.
15. Toyofuku M, Schild S, Kaparakis-Liaskos M, Eberl L. Composition and functions of bacterial membrane vesicles. Nat Rev Microbiol 2023;21:415-30.
16. Luo X, Yan X, Yin D, et al. A bibliometric systematic review of extracellular vesicles in eye diseases from 2003 to 2022. Medicine 2023;102:e34831.
17. Jafari N, Khoradmehr A, Moghiminasr R, Seyed Habashi M. Mesenchymal stromal/stem cells-derived exosomes as an antimicrobial weapon for orodental infections. Front Microbiol 2021;12:795682.
18. Sartorio MG, Pardue EJ, Scott NE, Feldman MF. Human gut bacteria tailor extracellular vesicle cargo for the breakdown of diet- and host-derived glycans. Proc Natl Acad Sci U S A 2023;120:e2306314120.
19. Segal LN, Blaser MJ. A brave new world: the lung microbiota in an era of change. Ann Am Thorac Soc 2014;11 Suppl 1:S21-7.
20. Amabebe E, Robert FO, Agbalalah T, Orubu ESF. Microbial dysbiosis-induced obesity: role of gut microbiota in homoeostasis of energy metabolism. Br J Nutr 2020;123:1127-37.
21. Amabebe E, Anumba DOC. Female gut and genital tract microbiota-induced crosstalk and differential effects of short-chain fatty acids on immune sequelae. Front Immunol 2020;11:2184.
22. Amabebe E, Anumba DOC. The vaginal microenvironment: the physiologic role of lactobacilli. Front Med 2018;5:181.
23. Tian CM, Yang MF, Xu HM, et al. Emerging role of bacterial outer membrane vesicle in gastrointestinal tract. Gut Pathog 2023;15:20.
24. Fucarino A, Pitruzzella A, Burgio S, Zarcone MC, Modica DM, et al. Extracellular vesicles in airway homeostasis and pathophysiology. Applied Sciences 2021;11:9933.
25. Hosseini-Giv N, Basas A, Hicks C, El-Omar E, El-Assaad F, Hosseini-Beheshti E. Bacterial extracellular vesicles and their novel therapeutic applications in health and cancer. Front Cell Infect Microbiol 2022;12:962216.
26. Menon R, Khanipov K, Radnaa E, et al. Amplification of microbial DNA from bacterial extracellular vesicles from human placenta. Front Microbiol 2023;14:1213234.
27. Yáñez-Mó M, Siljander PR, Andreu Z, et al. Biological properties of extracellular vesicles and their physiological functions. J Extracell Vesicles 2015;4:27066.
28. Koeppen K, Hampton TH, Jarek M, et al. A novel mechanism of host-pathogen interaction through srna in bacterial outer membrane vesicles. PLoS Pathog 2016;12:e1005672.
29. Sheller-Miller S, Choi K, Choi C, Menon R. Cyclic-recombinase-reporter mouse model to determine exosome communication and function during pregnancy. Am J Obstet Gynecol 2019;221:502.e1-502.e12.
30. Sheller-Miller S, Lei J, Saade G, Salomon C, Burd I, Menon R. Feto-maternal trafficking of exosomes in murine pregnancy models. Front Pharmacol 2016;7:432.
31. Joshi B, Singh B, Nadeem A, et al. Transcriptome profiling of staphylococcus aureus associated extracellular vesicles reveals presence of small RNA-cargo. Front Mol Biosci 2020;7:566207.
32. Gangoda L, Boukouris S, Liem M, Kalra H, Mathivanan S. Extracellular vesicles including exosomes are mediators of signal transduction: are they protective or pathogenic? Proteomics 2015;15:260-71.
33. van der Pol E, Böing AN, Harriso P, Sturk A, Nieuwland R. Classification, functions, and clinical relevance of extracellular vesicles. Pharmacol Rev 2012;64:676-705.
34. Spencer N, Yeruva L. Role of bacterial infections in extracellular vesicles release and impact on immune response. Biomed J 2021;44:157-64.
35. Menon R, Shahin H. Extracellular vesicles in spontaneous preterm birth. Am J Reprod Immunol 2021;85:e13353.
36. Paul N, Sultana Z, Fisher JJ, Maiti K, Smith R. Extracellular vesicles- crucial players in human pregnancy. Placenta 2023;140:30-8.
37. Anand S, Samuel M, Kumar S, Mathivanan S. Ticket to a bubble ride: cargo sorting into exosomes and extracellular vesicles. Biochim Biophys Acta Proteins Proteom 2019;1867:140203.
38. Wei H, Chen Q, Lin L, et al. Regulation of exosome production and cargo sorting. Int J Biol Sci 2021;17:163-77.
39. Zhang Y, Tang Y, Sun X, et al. Exporting proteins associated with senescence repair via extracellular vesicles may be associated with early pregnancy loss. Cells 2022;11:2772.
40. Théry C, Witwer KW, Aikawa E, et al. Minimal information for studies of extracellular vesicles 2018 (MISEV2018): a position statement of the international society for extracellular vesicles and update of the MISEV2014 guidelines. J Extracell Vesicles 2018;7:1535750.
41. Konoshenko MY, Lekchnov EA, Vlassov AV, Laktionov PP. Isolation of extracellular vesicles: general methodologies and latest trends. Biomed Res Int 2018;2018:8545347.
42. Luo R, Chang Y, Liang H, Zhang W, Song Y, et al. Interactions between extracellular vesicles and microbiome in human diseases: new therapeutic opportunities. iMeta 2023;2:e86.
43. Schorey JS, Cheng Y, McManus WR. Bacteria- and host-derived extracellular vesicles - two sides of the same coin? J Cell Sci 2021:134.
44. Ozkocak DC, Phan TK, Poon IKH. Translating extracellular vesicle packaging into therapeutic applications. Front Immunol 2022;13:946422.
45. Bettio V, Mazzucco E, Antona A, et al. Extracellular vesicles from human plasma for biomarkers discovery: impact of anticoagulants and isolation techniques. PLoS One 2023;18:e0285440.
46. Buzás EI, Tóth EÁ, Sódar BW, Szabó-Taylor KÉ. Molecular interactions at the surface of extracellular vesicles. Semin Immunopathol 2018;40:453-64.
47. Meloni M, Agliardi C, Guerini FR, et al. Oligomeric alpha-synuclein and STX-1A from neural-derived extracellular vesicles (NDEVs) as possible biomarkers of REM sleep behavior disorder in Parkinson’s disease: a preliminary cohort study. Int J Mol Sci 2023;24:8839.
48. Mansur S, Habib S, Hawkins M, Brown SR, Weinman ST, Bao Y. Preparation of nanoparticle-loaded extracellular vesicles using direct flow filtration. Pharmaceutics 2023;15:1551.
49. Ahmed W, Kuniyan MS, Jawed AM, Chen L. Engineered extracellular vesicles for drug delivery in therapy of stroke. Pharmaceutics 2023;15:2173.
50. Ekström K, Crescitelli R, Pétursson HI, Johansson J, Lässer C, Olofsson Bagge R. Characterization of surface markers on extracellular vesicles isolated from lymphatic exudate from patients with breast cancer. BMC Cancer 2022;22:50.
51. Minic Z, Li Y, Hüttmann N, Uppal GK, D'Mello R, Berezovski MV. Lysine acetylome of breast cancer-derived small extracellular vesicles reveals specific acetylation patterns for metabolic enzymes. Biomedicines 2023;11:1076.
52. Giovanazzi A, van Herwijnen MJC, Kleinjan M, van der Meulen GN, Wauben MHM. Surface protein profiling of milk and serum extracellular vesicles unveils body fluid-specific signatures. Sci Rep 2023;13:8758.
53. Honorato-Mauer J, Xavier G, Ota VK, et al. Alterations in microRNA of extracellular vesicles associated with major depression, attention-deficit/hyperactivity and anxiety disorders in adolescents. Transl Psychiatry 2023;13:47.
54. Nabhan JF, Hu R, Oh RS, Cohen SN, Lu Q. Formation and release of arrestin domain-containing protein 1-mediated microvesicles (ARMMs) at plasma membrane by recruitment of TSG101 protein. Proc Natl Acad Sci U S A 2012;109:4146-51.
55. Hoshino A, Kim HS, Bojmar L, et al. Extracellular vesicle and particle biomarkers define multiple human cancers. Cell 2020;182:1044-1061.e18.
56. Dunlop RA, Banack SA, Cox PA. L1CAM immunocapture generates a unique extracellular vesicle population with a reproducible miRNA fingerprint. RNA Biol 2023;20:140-8.
57. Hsu CC, Yang Y, Kannisto E, et al. Simultaneous detection of tumor derived exosomal protein-microRNA pairs with an Exo-PROS biosensor for cancer diagnosis. ACS Nano 2023;17:8108-22.
58. Senda A, Kojima M, Watanabe A, et al. Profiles of lipid, protein and microRNA expression in exosomes derived from intestinal epithelial cells after ischemia-reperfusion injury in a cellular hypoxia model. PLoS One 2023;18:e0283702.
59. Yan H, Wen Y, Tian Z, et al. A one-pot isothermal Cas12-based assay for the sensitive detection of microRNAs. Nat Biomed Eng 2023;7:1583-601.
60. Moreno-Gonzalo O, Fernandez-Delgado I, Sanchez-Madrid F. Post-translational add-ons mark the path in exosomal protein sorting. Cell Mol Life Sci 2018;75:1-19.
61. Christ L, Raiborg C, Wenzel EM, Campsteijn C, Stenmark H. Cellular functions and molecular mechanisms of the ESCRT membrane-scission machinery. Trends Biochem Sci 2017;42:42-56.
62. Colombo M, Moita C, van Niel G, et al. Analysis of ESCRT functions in exosome biogenesis, composition and secretion highlights the heterogeneity of extracellular vesicles. J Cell Sci 2013;126:5553-65.
63. Stuffers S, Sem Wegner C, Stenmark H, Brech A. Multivesicular endosome biogenesis in the absence of ESCRTs. Traffic 2009;10:925-37.
64. Trajkovic K, Hsu C, Chiantia S, et al. Ceramide triggers budding of exosome vesicles into multivesicular endosomes. Science 2008;319:1244-7.
65. Andreu Z, Yáñez-Mó M. Tetraspanins in extracellular vesicle formation and function. Front Immunol 2014;5:442.
66. Chen K, Liang J, Qin T, Zhang Y, Chen X, Wang Z. The role of extracellular vesicles in embryo implantation. Front Endocrinol 2022;13:809596.
67. Dehghan Z, Rezaee D, Noori E, et al. Exosomes as modulators of embryo implantation. Mol Biol Rep 2024;51:284.
68. Kowalczyk A, Wrzecińska M, Czerniawska-Piątkowska E, Kupczyński R. Exosomes - spectacular role in reproduction. Biomed Pharmacother 2022;148:112752.
69. Ghafourian M, Mahdavi R, Akbari Jonoush Z, et al. The implications of exosomes in pregnancy: emerging as new diagnostic markers and therapeutics targets. Cell Commun Signal 2022;20:51.
70. Shi S, Tan Q, Liang J, et al. Placental trophoblast cell-derived exosomal microRNA-1290 promotes the interaction between endometrium and embryo by targeting LHX6. Mol Ther Nucleic Acids 2021;26:760-72.
71. Aloi N, Drago G, Ruggieri S, Cibella F, Colombo P, Longo V. Extracellular vesicles and immunity: at the crossroads of cell communication. Int J Mol Sci 2024;25:1205.
72. Yang J, Li L, Wang L, et al. Trophoblast-derived miR-410-5p induces M2 macrophage polarization and mediates immunotolerance at the fetal-maternal interface by targeting the STAT1 signaling pathway. J Transl Med 2024;22:19.
73. Bai K, Li J, Lin L, et al. Placenta exosomal miRNA-30d-5p facilitates decidual macrophage polarization by targeting HDAC9. J Leukoc Biol 2023;113:434-44.
74. Menon R. Fetal inflammatory response at the fetomaternal interface: a requirement for labor at term and preterm. Immunol Rev 2022;308:149-67.
75. Shepherd MC, Radnaa E, Tantengco OA, et al. Extracellular vesicles from maternal uterine cells exposed to risk factors cause fetal inflammatory response. Cell Commun Signal 2021;19:100.
76. Tantengco OAG, Radnaa E, Shahin H, Kechichian T, Menon R. Cross talk: trafficking and functional impact of maternal exosomes at the feto-maternal interface under normal and pathologic states†. Biol Reprod 2021;105:1562-76.
77. Radnaa E, Richardson LS, Sheller-Miller S, et al. Extracellular vesicle mediated feto-maternal HMGB1 signaling induces preterm birth. Lab Chip 2021;21:1956-73.
78. Buca D, Bologna G, D'Amico A, et al. Extracellular vesicles in feto-maternal crosstalk and pregnancy disorders. Int J Mol Sci 2020;21:2120.
79. Zhou X, Xie F, Wang L, Zhang L, Zhang S, et al. The function and clinical application of extracellular vesicles in innate immune regulation. Cell Mol Immunol 2020;17:323-34.
80. Sherman CD, Lodha S, Sahoo S. EV cargo sorting in therapeutic development for cardiovascular disease. Cells 2021;10:1500.
81. Carnino JM, Lee H, Jin Y. Isolation and characterization of extracellular vesicles from Broncho-alveolar lavage fluid: a review and comparison of different methods. Respir Res 2019;20:240.
82. De Toro J, Herschlik L, Waldner C, Mongini C. Emerging roles of exosomes in normal and pathological conditions: new insights for diagnosis and therapeutic applications. Front Immunol 2015;6:203.
83. Babst M, Sato TK, Banta LM, Emr SD. Endosomal transport function in yeast requires a novel AAA-type ATPase, Vps4p. EMBO J 1997;16:1820-31.
84. Smith VL, Jackson L, Schorey JS. Ubiquitination as a mechanism to transport soluble mycobacterial and eukaryotic proteins to exosomes. J Immunol 2015;195:2722-30.
85. Frankel EB, Audhya A. ESCRT-dependent cargo sorting at multivesicular endosomes. Semin Cell Dev Biol 2018;74:4-10.
86. Dores MR, Chen B, Lin H, et al. ALIX binds a YPX3L motif of the GPCR PAR1 and mediates ubiquitin-independent ESCRT-III/MVB sorting. J Cell Bioll 2012;197:407-19.
87. Dores MR, Grimsey NJ, Mendez F, Trejo J. ALIX Regulates the ubiquitin-independent lysosomal sorting of the P2Y1 purinergic receptor via a YPX3L motif. PLoS One 2016;11:e0157587.
88. Knorre DG, Kudryashova NV, Godovikova TS. Chemical and functional aspects of posttranslational modification of proteins. Acta Naturae 2009;1:29-51.
89. Atukorala I, Mathivanan S. The role of post-translational modifications in targeting protein cargo to extracellular vesicles. In: Mathivanan S, Fonseka P, Nedeva C, Atukorala I, editors. New Frontiers: Extracellular Vesicles. Cham: Springer International Publishing; 2021. pp. 45-60.
90. Carnino JM, Ni K, Jin Y. Post-translational modification regulates formation and cargo-loading of extracellular vesicles. Front Immunol 2020;11:948.
91. Li S, Iakoucheva LM, Mooney SD, Radivojac P. Loss of post-translational modification sites in disease. Pac Symp Biocomput ;2010:337-47.
92. Moreno-Gonzalo O, Villarroya-Beltri C, Sánchez-Madrid F. Post-translational modifications of exosomal proteins. Front Immunol 2014;5:383.
93. Buschow SI, van Balkom BW, Aalberts M, Heck AJ, Wauben M, Stoorvogel W. MHC class II-associated proteins in B-cell exosomes and potential functional implications for exosome biogenesis. Immunol Cell Biol 2010;88:851-6.
94. Buschow SI, Nolte-'t Hoen EN, van Niel G, et al. MHC II in dendritic cells is targeted to lysosomes or T cell-induced exosomes via distinct multivesicular body pathways. Traffic 2009;10:1528-42.
95. Gauvreau ME, Côté MH, Bourgeois-Daigneault MC, et al. Sorting of MHC class II molecules into exosomes through a ubiquitin-independent pathway. Traffic 2009;10:1518-27.
96. Chitti SV, Fonseka P, Mathivanan S. Emerging role of extracellular vesicles in mediating cancer cachexia. Biochem Soc Trans 2018;46:1129-36.
97. Murillo OD, Thistlethwaite W, Rozowsky J, et al. exRNA atlas analysis reveals distinct extracellular RNA cargo types and their carriers present across human biofluids. Cell 2019;177:463-477.e15.
98. Gallo A, Tandon M, Alevizos I, Illei GG. The majority of microRNAs detectable in serum and saliva is concentrated in exosomes. PLoS One 2012;7:e30679.
99. Cheng L, Sharples RA, Scicluna BJ, Hill AF. Exosomes provide a protective and enriched source of miRNA for biomarker profiling compared to intracellular and cell-free blood. J Extracell Vesicles 2014;26:3.
100. Groot M, Lee H. Sorting mechanisms for micrornas into extracellular vesicles and their associated diseases. Cells 2020;9:1044.
101. Zhang J, Li S, Li L, et al. Exosome and exosomal microRNA: trafficking, sorting, and function. Genom Proteom Bioinf 2015;13:17-24.
102. Zheng D, Huo M, Li B, et al. The role of exosomes and exosomal microRNA in cardiovascular disease. Front Cell Dev Biol 2020;8:616161.
103. Lázaro-Ibáñez E, Lässer C, Shelke GV, et al. DNA analysis of low- and high-density fractions defines heterogeneous subpopulations of small extracellular vesicles based on their DNA cargo and topology. J Extracell Vesicles 2019;8:1656993.
105. Elzanowska J, Semira C, Costa-Silva B. DNA in extracellular vesicles: biological and clinical aspects. Mol Oncol 2021;15:1701-14.
106. Ghanam J, Chetty VK, Barthel L, Reinhardt D, Hoyer PF, et al. DNA in extracellular vesicles: from evolution to its current application in health and disease. Cell Biosci 2022;12:37.
107. Yokoi A, Villar-Prados A, Oliphint PA, et al. Mechanisms of nuclear content loading to exosomes. Sci Adv 2019;5:eaax8849.
108. Malkin EZ, Bratman SV. Bioactive DNA from extracellular vesicles and particles. Cell Death Dis 2020;11:584.
109. Sansone P, Savini C, Kurelac I, et al. Packaging and transfer of mitochondrial DNA via exosomes regulate escape from dormancy in hormonal therapy-resistant breast cancer. Proc Natl Acad Sci U S A 2017;114:E9066-75.
110. Skotland T, Sandvig K, Llorente A. Lipids in exosomes: current knowledge and the way forward. Prog Lipid Res 2017;66:30-41.
111. Haraszti RA, Didiot MC, Sapp E, et al. High-resolution proteomic and lipidomic analysis of exosomes and microvesicles from different cell sources. J Extracell Vesicles 2016;5:32570.
112. Tan A, Rajadas J, Seifalian AM. Exosomes as nano-theranostic delivery platforms for gene therapy. Adv Drug Deliv Rev 2013;65:357-67.
113. Xiu F, Côté MH, Bourgeois-Daigneault MC, et al. Cutting edge: HLA-DO impairs the incorporation of HLA-DM into exosomes. J Immunol 2011;187:1547-51.
114. van Niel G, D'Angelo G, Raposo G. Shedding light on the cell biology of extracellular vesicles. Nat Rev Mol Cell Biol 2018;19:213-28.
115. Shen B, Wu N, Yang JM, Gould SJ. Protein targeting to exosomes/microvesicles by plasma membrane anchors. J Biol Chem 2011;286:14383-95.
116. Cesselli D, Parisse P, Aleksova A, et al. Extracellular vesicles: how drug and pathology interfere with their biogenesis and function. Front Physiol 2018;9:1394.
117. Tejera E, Rocha-Perugini V, López-Martín S, et al. CD81 regulates cell migration through its association with Rac GTPase. Mol Biol Cell 2013;24:261-73.
118. Chairoungdua A, Smith DL, Pochard P, Hull M, Caplan MJ. Exosome release of β-catenin: a novel mechanism that antagonizes Wnt signaling. J Cell Biol 2010;190:1079-91.
119. Rana S, Yue S, Stadel D, Zöller M. Toward tailored exosomes: the exosomal tetraspanin web contributes to target cell selection. Int J Biochem Cell Biol 2012;44:1574-84.
120. Barnes BJ, Somerville CC. Modulating cytokine production via select packaging and secretion from extracellular vesicles. Front Immunol 2020;11:1040.
121. Toribio V, Yáñez-Mó M. Tetraspanins interweave EV secretion, endosomal network dynamics and cellular metabolism. Eur J Cell Biol 2022;101:151229.
122. Kim JH, Lee J, Park J, Gho YS. Gram-negative and gram-positive bacterial extracellular vesicles. Semin Cell Dev Biol 2015;40:97-104.
123. Yu YJ, Wang XH, Fan GC. Versatile effects of bacterium-released membrane vesicles on mammalian cells and infectious/inflammatory diseases. Acta Pharmacol Sin 2018;39:514-33.
124. Liu Y, Defourny KAY, Smid EJ, Abee T. Gram-positive bacterial extracellular vesicles and their impact on health and disease. Front Microbiol 2018;9:1502.
125. Lee J, Lee EY, Kim SH, et al. Staphylococcus aureus extracellular vesicles carry biologically active β-lactamase. Antimicrob Agents Chemother 2013;57:2589-95.
126. Brown L, Kessler A, Cabezas-Sanchez P, Luque-Garcia JL, Casadevall A. Extracellular vesicles produced by the gram-positive bacterium Bacillus subtilis are disrupted by the lipopeptide surfactin. Mol Microbiol 2014;93:183-98.
127. Brown L, Wolf JM, Prados-Rosales R, Casadevall A. Through the wall: extracellular vesicles in gram-positive bacteria, mycobacteria and fungi. Nat Rev Microbiol 2015;13:620-30.
128. Kaparakis-Liaskos M, Ferrero RL. Immune modulation by bacterial outer membrane vesicles. Nat Rev Immunol 2015;15:375-87.
129. Toyofuku M, Tashiro Y, Hasegawa Y, Kurosawa M, Nomura N. Bacterial membrane vesicles, an overlooked environmental colloid: Biology, environmental perspectives and applications. Adv Colloid Interface Sci 2015;226:65-77.
130. Tulkens J, De Wever O, Hendrix A. Analyzing bacterial extracellular vesicles in human body fluids by orthogonal biophysical separation and biochemical characterization. Nat Protoc 2020;15:40-67.
131. Caruana JC, Walper SA. Bacterial membrane vesicles as mediators of microbe - microbe and microbe - host community interactions. Front Microbiol 2020;11:432.
132. Toyofuku M, Nomura N, Eberl L. Types and origins of bacterial membrane vesicles. Nat Rev Microbiol 2019;17:13-24.
133. Wang X, Thompson CD, Weidenmaier C, Lee JC. Release of staphylococcus aureus extracellular vesicles and their application as a vaccine platform. Nat Commun 2018;9:1379.
134. Xie S, Zhang Q, Jiang L. Current knowledge on exosome biogenesis, cargo-sorting mechanism and therapeutic implications. Membranes 2022;12:498.
135. Mashburn LM, Whiteley M. Membrane vesicles traffic signals and facilitate group activities in a prokaryote. Nature 2005;437:422-5.
136. Toyofuku M, Morinaga K, Hashimoto Y, Uhl J, Shimamura H, et al. Membrane vesicle-mediated bacterial communication. ISME J 2017;11:1504-9.
137. Kim YY, Joh JS, Lee JY. Importance of microbial extracellular vesicle in the pathogenesis of asthma and chronic obstructive pulmonary disease and its diagnostic potential. Asia Pac Allergy 2020;10:e25.
138. Chang X, Wang SL, Zhao SB, et al. Extracellular vesicles with possible roles in gut intestinal tract homeostasis and IBD. Mediators Inflamm 2020;2020:1945832.
139. Kuipers ME, Hokke CH, Smits HH, Nolte-'t Hoen ENM. Pathogen-derived extracellular vesicle-associated molecules that affect the host immune system: an overview. Front Microbiol 2018;9:2182.
140. Mashburn-Warren LM, Whiteley M. Special delivery: vesicle trafficking in prokaryotes. Mol Microbiol 2006;61:839-46.
141. Chen F, Cui G, Wang S, et al. Outer membrane vesicle-associated lipase FtlA enhances cellular invasion and virulence in Francisella tularensis LVS. Emerg Microbes Infect 2017;6:e66.
142. Finethy R, Luoma S, Orench-Rivera N, et al. Inflammasome activation by bacterial outer membrane vesicles requires guanylate binding proteins. mBio 2017;8:e01188-17.
143. Losier TT, Akuma M, McKee-Muir OC, et al. AMPK promotes Xenophagy through priming of autophagic kinases upon detection of bacterial outer membrane vesicles. Cell Rep 2019;26:2150-2165.e5.
144. MacDonald IA, Kuehn MJ. Offense and defense: microbial membrane vesicles play both ways. Res Microbiol 2012;163:607-18.
145. Bitto NJ, Chapman R, Pidot S, Costin A, Lo C, et al. Bacterial membrane vesicles transport their DNA cargo into host cells. Sci Rep 2017;7:7072.
146. Schaar V, Uddbäck I, Nordström T, Riesbeck K. Group A streptococci are protected from amoxicillin-mediated killing by vesicles containing β-lactamase derived from Haemophilus influenzae. J Antimicrob Chemother 2014;69:117-20.
147. Schaar V, Paulsson M, Mörgelin M, Riesbeck K. Outer membrane vesicles shield Moraxella catarrhalis β-lactamase from neutralization by serum IgG. J Antimicrob Chemother 2013;68:593-600.
148. Bitto NJ, Kaparakis-Liaskos M. The therapeutic benefit of bacterial membrane vesicles. Int J Mol Sci 2017;18:1287.
149. Schetters STT, Jong WSP, Horrevorts SK, et al. Outer membrane vesicles engineered to express membrane-bound antigen program dendritic cells for cross-presentation to CD8+ T cells. Acta Biomater 2019;91:248-57.
150. Choi SJ, Kim MH, Jeon J, et al. Active immunization with extracellular vesicles derived from staphylococcus aureus effectively protects against staphylococcal lung infections, mainly via Th1 cell-mediated immunity. PLoS One 2015;10:e0136021.
151. Chen G, Bai Y, Li Z, Wang F, Fan X, Zhou X. Bacterial extracellular vesicle-coated multi-antigenic nanovaccines protect against drug-resistant Staphylococcus aureus infection by modulating antigen processing and presentation pathways. Theranostics 2020;10:7131-49.
152. Acevedo R, Fernández S, Zayas C, et al. Bacterial outer membrane vesicles and vaccine applications. Front Immunol 2014;5:121.
153. Tartaglia NR, Nicolas A, Rodovalho VR, et al. Extracellular vesicles produced by human and animal staphylococcus aureus strains share a highly conserved core proteome. Sci Rep 2020;10:8467.
154. Jan AT. Outer membrane vesicles (OMVs) of gram-negative bacteria: a perspective update. Front Microbiol 2017;8:1053.
155. Lee J, Kim OY, Gho YS. Proteomic profiling of gram-negative bacterial outer membrane vesicles: current perspectives. . Proteomics Clin Appl 2016;10:897-909.
156. Cahill BK, Seeley KW, Gutel D, Ellis TN. Klebsiella pneumoniae O antigen loss alters the outer membrane protein composition and the selective packaging of proteins into secreted outer membrane vesicles. Microbiol Res 2015;180:1-10.
157. Chen C, Kawamoto J, Kawai S, et al. Isolation of a novel bacterial strain capable of producing abundant extracellular membrane vesicles carrying a single major cargo protein and analysis of its transport mechanism. Front Microbiol 2019;10:3001.
158. Schwechheimer C, Kuehn MJ. Outer-membrane vesicles from Gram-negative bacteria: biogenesis and functions. Nat Rev Microbiol 2015;13:605-19.
159. Haurat MF, Aduse-Opoku J, Rangarajan M, et al. Selective sorting of cargo proteins into bacterial membrane vesicles. J Biol Chem 2011;286:1269-76.
160. Resch U, Tsatsaronis JA, Le Rhun A, et al. A two-component regulatory system impacts extracellular membrane-derived vesicle production in group a streptococcus. mBio 2016;7:e00207-16.
161. Veith PD, Chen YY, Gorasia DG, et al. Porphyromonas gingivalis outer membrane vesicles exclusively contain outer membrane and periplasmic proteins and carry a cargo enriched with virulence factors. J Proteome Res 2014;13:2420-32.
162. Sharp PM, Li WH. The codon Adaptation Index--a measure of directional synonymous codon usage bias, and its potential applications. Nucleic Acids Res 1987;15:1281-95.
163. dos Reis M, Wernisch L, Savva R. Unexpected correlations between gene expression and codon usage bias from microarray data for the whole Escherichia coli K-12 genome. Nucleic Acids Res 2003;31:6976-85.
164. Ishihama Y, Schmidt T, Rappsilber J, et al. Protein abundance profiling of the Escherichia coli cytosol. BMC Genomics 2008;9:102.
165. Nguyen TT, Saxena A, Beveridge TJ. Effect of surface lipopolysaccharide on the nature of membrane vesicles liberated from the Gram-negative bacterium Pseudomonas aeruginosa. J Electron Microsc 2003;52:465-9.
166. Lo Cicero A, Stahl PD, Raposo G. Extracellular vesicles shuffling intercellular messages: for good or for bad. Curr Opin Cell Biol 2015;35:69-77.
167. György B, Hung ME, Breakefield XO, Leonard JN. Therapeutic applications of extracellular vesicles: clinical promise and open questions. Annu Rev Pharmacol Toxicol 2015;55:439-64.
168. Skog J, Würdinger T, van Rijn S, et al. Glioblastoma microvesicles transport RNA and proteins that promote tumour growth and provide diagnostic biomarkers. Nat Cell Biol 2008;10:1470-6.
169. Cecil JD, Sirisaengtaksin N, O'Brien-Simpson NM, Krachler AM. Outer membrane vesicle-host cell interactions. Microbiol Spectr 2019;7:Online ahead of print.
170. O'Donoghue EJ, Krachler AM. Mechanisms of outer membrane vesicle entry into host cells. Cell Microbiol 2016;18:1508-17.
171. Ellis TN, Leiman SA, Kuehn MJ. Naturally produced outer membrane vesicles from Pseudomonas aeruginosa elicit a potent innate immune response via combined sensing of both lipopolysaccharide and protein components. Infect Immun 2010;78:3822-31.
172. Bomberger JM, Maceachran DP, Coutermarsh BA, Ye S, O'Toole GA, Stanton BA. Long-distance delivery of bacterial virulence factors by Pseudomonas aeruginosa outer membrane vesicles. PLoS Pathog 2009;5:e1000382.
173. Furuta N, Tsuda K, Omori H, Yoshimori T, Yoshimura F, Amano A. Porphyromonas gingivalis outer membrane vesicles enter human epithelial cells via an endocytic pathway and are sorted to lysosomal compartments. Infect Immun 2009;77:4187-96.
174. Olofsson A, Nygård Skalman L, Obi I, Lundmark R, Arnqvist A. Uptake of Helicobacter pylori vesicles is facilitated by clathrin-dependent and clathrin-independent endocytic pathways. mBio 2014;5:e00979-14.
175. Parker H, Chitcholtan K, Hampton MB, Keenan JI. Uptake of helicobacter pylori outer membrane vesicles by gastric epithelial cells. Infect Immun 2010;78:5054-61.
176. Bielaszewska M, Rüter C, Bauwens A, et al. Host cell interactions of outer membrane vesicle-associated virulence factors of enterohemorrhagic Escherichia coli O157: intracellular delivery, trafficking and mechanisms of cell injury. PLoS Pathog 2017;13:e1006159.
177. Kunsmann L, Ruter C, Bauwens A, Greune L, Gluder M, et al. Virulence from vesicles: novel mechanisms of host cell injury by Escherichia coli O104:H4 outbreak strain. Sci Rep 2015;5:13252.
178. Pollak CN, Delpino MV, Fossati CA, Baldi PC. Outer membrane vesicles from Brucella abortus promote bacterial internalization by human monocytes and modulate their innate immune response. PLoS One 2012;7:e50214.
179. Sharpe SW, Kuehn MJ, Mason KM. Elicitation of epithelial cell-derived immune effectors by outer membrane vesicles of nontypeable Haemophilus influenzae. Infect Immun 2011;79:4361-9.
180. Chatterjee D, Chaudhuri K. Association of cholera toxin with Vibrio cholerae outer membrane vesicles which are internalized by human intestinal epithelial cells. FEBS Lett 2011;585:1357-62.
181. Kesty NC, Mason KM, Reedy M, Miller SE, Kuehn MJ. Enterotoxigenic Escherichia coli vesicles target toxin delivery into mammalian cells. EMBO J 2004;23:4538-49.
182. Bauman SJ, Kuehn MJ. Pseudomonas aeruginosa vesicles associate with and are internalized by human lung epithelial cells. BMC Microbiol 2009;9:26.
183. Elmi A, Watson E, Sandu P, et al. Campylobacter jejuni outer membrane vesicles play an important role in bacterial interactions with human intestinal epithelial cells. Infect Immun 2012;80:4089-98.
184. Mondal A, Tapader R, Chatterjee NS, et al. Cytotoxic and inflammatory responses induced by outer membrane vesicle-associated biologically active proteases from vibrio cholerae. Infect Immun 2016;84:1478-90.
185. Kaparakis M, Turnbull L, Carneiro L, et al. Bacterial membrane vesicles deliver peptidoglycan to NOD1 in epithelial cells. Cell Microbiol 2010;12:372-85.
186. Schaar V, de Vries SP, Perez Vidakovics ML, et al. Multicomponent Moraxella catarrhalis outer membrane vesicles induce an inflammatory response and are internalized by human epithelial cells. Cell Microbiol 2011;13:432-49.
187. Vidakovics ML, Jendholm J, Mörgelin M, et al. B cell activation by outer membrane vesicles--a novel virulence mechanism. PLoS Pathog 2010;6:e1000724.
188. Jin JS, Kwon SO, Moon DC, et al. Acinetobacter baumannii secretes cytotoxic outer membrane protein A via outer membrane vesicles. PLoS One 2011;6:e17027.
189. Rompikuntal PK, Thay B, Khan MK, et al. Perinuclear localization of internalized outer membrane vesicles carrying active cytolethal distending toxin from Aggregatibacter actinomycetemcomitans. Infect Immun 2012;80:31-42.
190. Thay B, Damm A, Kufer TA, Wai SN, Oscarsson J. Aggregatibacter actinomycetemcomitans outer membrane vesicles are internalized in human host cells and trigger NOD1- and NOD2-dependent NF-κB activation. Infect Immun 2014;82:4034-46.
191. Jäger J, Keese S, Roessle M, Steinert M, Schromm AB. Fusion of legionella pneumophila outer membrane vesicles with eukaryotic membrane systems is a mechanism to deliver pathogen factors to host cell membranes. Cell Microbiol 2015;17:607-20.
192. Mulcahy LA, Pink RC, Carter DR. Routes and mechanisms of extracellular vesicle uptake. J Extracell Vesicles 2014;3:24641.
194. Amano A, Takeuchi H, Furuta N. Outer membrane vesicles function as offensive weapons in host-parasite interactions. Microbes Infect 2010;12:791-8.
195. Mettlen M, Chen PH, Srinivasan S, Danuser G, Schmid SL. Regulation of clathrin-mediated endocytosis. Annu Rev Biochem 2018;87:871-96.
196. Kaksonen M, Roux A. Mechanisms of clathrin-mediated endocytosis. Nat Rev Mol Cell Biol 2018;19:313-26.
197. McMahon HT, Boucrot E. Molecular mechanism and physiological functions of clathrin-mediated endocytosis. Nat Rev Mol Cell Biol 2011;12:517-33.
198. Rewatkar PV, Parton RG, Parekh HS, Parat MO. Are caveolae a cellular entry route for non-viral therapeutic delivery systems? Adv Drug Deliv Rev 2015;91:92-108.
199. Sandvig K, van Deurs B. Transport of protein toxins into cells: pathways used by ricin, cholera toxin and Shiga toxin. FEBS Lett 2002;529:49-53.
200. Vanaja SK, Russo AJ, Behl B, et al. Bacterial outer membrane vesicles mediate cytosolic localization of LPS and caspase-11 activation. Cell 2016;165:1106-19.
201. Bielaszewska M, Rüter C, Kunsmann L, et al. Enterohemorrhagic Escherichia coli hemolysin employs outer membrane vesicles to target mitochondria and cause endothelial and epithelial apoptosis. PLoS Pathog 2013;9:e1003797.
202. Turner L, Bitto NJ, Steer DL, et al. Helicobacter pylori outer membrane vesicle size determines their mechanisms of host cell entry and protein content. Front Immunol 2018;9:1466.
203. Kesty NC, Kuehn MJ. Incorporation of heterologous outer membrane and periplasmic proteins into Escherichia coli outer membrane vesicles. J Biol Chem 2004;279:2069-76.
204. Machado FS, Rodriguez NE, Adesse D, et al. Recent Developments in the Interactions Between Caveolin and Pathogens. In: Jasmin J, Frank PG, Lisanti MP, editors. Caveolins and Caveolae. New York: Springer US; 2012. pp. 65-82.
205. Ritter TE, Fajardo O, Matsue H, Anderson RG, Lacey SW. Folate receptors targeted to clathrin-coated pits cannot regulate vitamin uptake. Proc Natl Acad Sci U S A 1995;92:3824-8.
206. Kim YR, Kim BU, Kim SY, et al. Outer membrane vesicles of Vibrio vulnificus deliver cytolysin-hemolysin VvhA into epithelial cells to induce cytotoxicity. Biochem Biophys Res Commun 2010;399:607-12.
207. Haas-Neill S, Forsythe P. A budding relationship: bacterial extracellular vesicles in the microbiota-gut-brain Axis. Int J Mol Sci 2020;21:8899.
208. Sender R, Fuchs S, Milo R. Revised estimates for the number of human and bacteria cells in the body. PLoS Biol 2016;14:e1002533.
209. Zhao G, Jones MK. Role of bacterial extracellular vesicles in manipulating infection. Infect Immun 2023;91:e0043922.
210. Dong XH, Ho MH, Liu B, et al. Role of porphyromonas gingivalis outer membrane vesicles in oral mucosal transmission of HIV. Sci Rep 2018;8:8812.
211. Bhar S, Zhao G, Bartel JD, et al. Bacterial extracellular vesicles control murine norovirus infection through modulation of antiviral immune responses. Front Immunol 2022;13:909949.
212. Amabebe E, Anumba DOC. Mechanistic insights into immune suppression and evasion in bacterial vaginosis. Curr Microbiol 2022;79:84.
213. Stentz R, Horn N, Cross K, et al. Cephalosporinases associated with outer membrane vesicles released by Bacteroides spp. protect gut pathogens and commensals against β-lactam antibiotics. J Antimicrob Chemother 2015;70:701-9.
215. Kulp A, Kuehn MJ. Biological functions and biogenesis of secreted bacterial outer membrane vesicles. Annu Rev Microbiol 2010;64:163-84.
216. Wang Y, Luo X, Xiang X, Hao C, Ma D. Roles of bacterial extracellular vesicles in systemic diseases. Front Microbiol 2023;14:1258860.
217. Alvarez CS, Badia J, Bosch M, Giménez R, Baldomà L. Outer membrane vesicles and soluble factors released by probiotic escherichia coli nissle 1917 and commensal ECOR63 enhance barrier function by regulating expression of tight junction proteins in intestinal epithelial cells. Front Microbiol 2016;7:1981.
218. Cecil JD, O'Brien-Simpson NM, Lenzo JC, et al. Outer membrane vesicles prime and activate macrophage inflammasomes and cytokine secretion in vitro and in vivo. Front Immunol 2017;8:1017.
219. Engevik MA, Danhof HA, Ruan W, et al. Fusobacterium nucleatum secretes outer membrane vesicles and promotes intestinal inflammation. mBio 2021;12:e02706-20.
220. Hong GE, Kim DG, Park EM, Nam BH, Kim YO, Kong IS. Identification of Vibrio anguillarum outer membrane vesicles related to immunostimulation in the Japanese flounder, Paralichthys olivaceus. Biosci Biotechnol Biochem 2009;73:437-9.
221. Prados-Rosales R, Baena A, Martinez LR, et al. Mycobacteria release active membrane vesicles that modulate immune responses in a TLR2-dependent manner in mice. J Clin Invest 2011;121:1471-83.
222. Balhuizen MD, Versluis CM, van Grondelle MO, Veldhuizen EJA, Haagsman HP. Modulation of outer membrane vesicle-based immune responses by cathelicidins. Vaccine 2022;40:2399-408.
223. Liu P, Wang X, Yang Q, et al. Collaborative action of microglia and astrocytes mediates neutrophil recruitment to the CNS to defend against Escherichia coli K1 infection. Int J Mol Sci 2022;23:6540.
224. Bitto NJ, Cheng L, Johnston EL, et al. Staphylococcus aureus membrane vesicles contain immunostimulatory DNA, RNA and peptidoglycan that activate innate immune receptors and induce autophagy. J Extracell Vesicles 2021;10:e12080.
225. Vitse J, Devreese B. The contribution of membrane vesicles to bacterial pathogenicity in cystic fibrosis infections and healthcare associated pneumonia. Front Microbiol 2020;11:630.
226. Surve MV, Anil A, Kamath KG, et al. Membrane vesicles of group B streptococcus disrupt feto-maternal barrier leading to preterm birth. PLoS Pathog 2016;12:e1005816.
227. Kaisanlahti A, Turunen J, Byts N, et al. Maternal microbiota communicates with the fetus through microbiota-derived extracellular vesicles. Microbiome 2023;11:249.
228. Nunzi E, Mezzasoma L, Bellezza I, et al. Microbiota-associated HAF-EVs regulate monocytes by triggering or inhibiting inflammasome activation. Int J Mol Sci 2023;24:2527.
229. Farrelly R, Kennedy MG, Spencer R, Forbes K. Extracellular vesicles as markers and mediators of pregnancy complications: gestational diabetes, pre-eclampsia, preterm birth and fetal growth restriction. J Physiol 2023;601:4973-88.
230. Morelli AE, Sadovsky Y. Extracellular vesicles and immune response during pregnancy: a balancing act. Immunol Rev 2022;308:105-22.
231. Takahashi A, Okada R, Nagao K, et al. Exosomes maintain cellular homeostasis by excreting harmful DNA from cells. Nat Commun 2017;8:15287.
232. Torralba D, Baixauli F, Villarroya-Beltri C, et al. Priming of dendritic cells by DNA-containing extracellular vesicles from activated T cells through antigen-driven contacts. Nat Commun 2018;9:2658.
233. Wang W, Kong P, Ma G, et al. Characterization of the release and biological significance of cell-free DNA from breast cancer cell lines. Oncotarget 2017;8:43180-91.
234. Kumari P, Vasudevan SO, Russo AJ, et al. Host extracellular vesicles confer cytosolic access to systemic LPS licensing non-canonical inflammasome sensing and pyroptosis. Nat Cell Biol 2023;25:1860-72.
235. Gelber SE, Aguilar JL, Lewis KL, Ratner AJ. Functional and phylogenetic characterization of Vaginolysin, the human-specific cytolysin from Gardnerella vaginalis. J Bacteriol 2008;190:3896-903.
236. Shishpal P, Kasarpalkar N, Singh D, Bhor VM. Characterization of Gardnerella vaginalis membrane vesicles reveals a role in inducing cytotoxicity in vaginal epithelial cells. Anaerobe 2020;61:102090.
237. Hopfner KP, Hornung V. Molecular mechanisms and cellular functions of cGAS-STING signalling. Nat Rev Mol Cell Biol 2020;21:501-21.
238. Allen ER, Whitefoot-Keliin KM, Palmatier EM, Mahon AR, Greenlee-Wacker MC. Extracellular vesicles from A23187-treated neutrophils cause cGAS-STING-dependent IL-6 production by macrophages. Front Immunol 2022;13:949451.
239. Cui JZ, Chew ZH, Lim LHK. New insights into nucleic acid sensor AIM2: The potential benefit in targeted therapy for cancer. Pharmacol Res 2024;200:107079.
240. Liu S, Feng M, Guan W. Mitochondrial DNA sensing by STING signaling participates in inflammation, cancer and beyond. Int J Cancer 2016;139:736-41.
241. Behnia F, Sheller S, Menon R. Mechanistic differences leading to infectious and sterile inflammation. Am J Reprod Immunol 2016;75:505-18.
242. Parris KM, Amabebe E, Cohen MC, Anumba DO. Placental microbial-metabolite profiles and inflammatory mechanisms associated with preterm birth. J Clin Pathol 2021;74:10-8.
243. Sheller-Miller S, Urrabaz-Garza R, Saade G, Menon R. Damage-associated molecular pattern markers HMGB1 and cell-Free fetal telomere fragments in oxidative-stressed amnion epithelial cell-derived exosomes. J Reprod Immunol 2017;123:3-11.
244. Menon R. Initiation of human parturition: signaling from senescent fetal tissues via extracellular vesicle mediated paracrine mechanism. Obstet Gynecol Sci 2019;62:199-211.
245. Tong M, Johansson C, Xiao F, et al. Antiphospholipid antibodies increase the levels of mitochondrial DNA in placental extracellular vesicles: alarmin-g for preeclampsia. Sci Rep 2017;7:16556.
246. Condrat CE, Varlas VN, Duică F, et al. Pregnancy-related extracellular vesicles revisited. Int J Mol Sci 2021;22:3904.
247. Nadeau-Vallée M, Obari D, Palacios J, et al. Sterile inflammation and pregnancy complications: a review. Reproduction 2016;152:R277-92.
248. Romero R, Chaiworapongsa T, Alpay Savasan Z, et al. Damage-associated molecular patterns (DAMPs) in preterm labor with intact membranes and preterm PROM: a study of the alarmin HMGB1. J Matern Fetal Neonatal Med 2011;24:1444-55.
249. Romero R, Chaiworapongsa T, Savasan ZA, et al. Clinical chorioamnionitis is characterized by changes in the expression of the alarmin HMGB1 and one of its receptors, sRAGE. J Matern Fetal Neonatal Med 2012;25:558-67.
250. Romero R, Miranda J, Chaiworapongsa T, et al. Prevalence and clinical significance of sterile intra-amniotic inflammation in patients with preterm labor and intact membranes. Am J Reprod Immunol 2014;72:458-74.
251. Marsman G, Zeerleder S, Luken BM. Extracellular histones, cell-free DNA, or nucleosomes: differences in immunostimulation. Cell Death Dis 2016;7:e2518.
252. Romero R, Espinoza J, Kusanovic JP, et al. The preterm parturition syndrome. BJOG 2006;113 Suppl 3:17-42.
253. Romero R, Miranda J, Chaemsaithong P, et al. Sterile and microbial-associated intra-amniotic inflammation in preterm prelabor rupture of membranes. J Matern Fetal Neonatal Med 2015;28:1394-409.
254. Romero R, Gómez R, Chaiworapongsa T, Conoscenti G, Kim JC, Kim YM. The role of infection in preterm labour and delivery. Paediatr Perinat Epidemiol 2001;15 Suppl 2:41-56.
255. Amabebe E, Anumba DO. The transmembrane G protein-coupled CXCR3 receptor-ligand system and maternal foetal allograft rejection. Placenta 2021;104:81-8.
256. Srinivasan U, Misra D, Marazita ML, Foxman B. Vaginal and oral microbes, host genotype and preterm birth. Med Hypotheses 2009;73:963-75.
257. Keelan JA. Intrauterine inflammatory activation, functional progesterone withdrawal, and the timing of term and preterm birth. J Reprod Immunol 2018;125:89-99.
258. Romero R, Gomez-Lopez N, Winters AD, et al. Evidence that intra-amniotic infections are often the result of an ascending invasion - a molecular microbiological study. J Perinat Med 2019;47:915-31.
259. Stafford GP, Parker JL, Amabebe E, et al. Spontaneous preterm birth is associated with differential expression of vaginal metabolites by lactobacilli-dominated microflora. Front Physiol 2017;8:615.
260. Gudnadottir U, Debelius JW, Du J, et al. The vaginal microbiome and the risk of preterm birth: a systematic review and network meta-analysis. Sci Rep 2022;12:7926.
261. Kosti I, Lyalina S, Pollard KS, Butte AJ, Sirota M. Meta-analysis of vaginal microbiome data provides new insights into preterm birth. Front Microbiol 2020;11:476.
262. Tantengco OAG, Menon R. Effects of Ureaplasma parvum infection in the exosome biogenesis-related proteins in ectocervical epithelial cells. Am J Reprod Immunol 2024;91:e13803.
263. Bento GFC, Richardson LS, da Silva MG, Tantengco OAG, Menon R. Modeling an ascending infection by Ureaplasma parvum and its cell signaling and inflammatory response at the feto-maternal interface. Am J Reprod Immunol 2023;90:e13770.
264. Amabebe E, Richardson LS, Bento GFC, et al. Ureaplasma parvum infection induces inflammatory changes in vaginal epithelial cells independent of sialidase. Mol Biol Rep 2023;50:3035-43.
265. Romero R, Miranda J, Chaiworapongsa T, et al. A novel molecular microbiologic technique for the rapid diagnosis of microbial invasion of the amniotic cavity and intra-amniotic infection in preterm labor with intact membranes. Am J Reprod Immunol 2014;71:330-58.
266. Cobo T, Vives I, Rodríguez-Trujillo A, et al. Impact of microbial invasion of amniotic cavity and the type of microorganisms on short-term neonatal outcome in women with preterm labor and intact membranes. Acta Obstet Gynecol Scand 2017;96:570-9.
267. Ovalle S A, Gómez M R, Martínez T MA, et al. [Outcome of microbial invasion of amniotic cavity in the preterm premature rupture of membranes]. Rev Med Chil 2005;133:51-61.
268. Oh KJ, Lee SE, Jung H, Kim G, Romero R, Yoon BH. Detection of ureaplasmas by the polymerase chain reaction in the amniotic fluid of patients with cervical insufficiency. J Perinat Med 2010;38:261-8.
269. Rowlands S, Danielewski JA, Tabrizi SN, Walker SP, Garland SM. Microbial invasion of the amniotic cavity in midtrimester pregnancies using molecular microbiology. Am J Obstet Gynecol 2017;217:71.e1-5.
270. Kasprzykowska U, Elias J, Elias M, Mączyńska B, Sobieszczańska BM. Colonization of the lower urogenital tract with Ureaplasma parvum can cause asymptomatic infection of the upper reproductive system in women: a preliminary study. Arch Gynecol Obstet 2014;289:1129-34.
271. Noda-Nicolau NM, Tantengco OAG, Polettini J, et al. Genital mycoplasmas and biomarkers of inflammation and their association with spontaneous preterm birth and preterm prelabor rupture of membranes: a systematic review and meta-analysis. Front Microbiol 2022;13:859732.
272. Kacerovsky M, Kukla R, Bolehovska R, et al. Prevalence and load of cervical Ureaplasma species with respect to intra-amniotic complications in women with preterm prelabor rupture of membranes before 34 weeks. Front Pharmacol 2022;13:860498.
273. Kacerovsky M, Stranik J, Kukla R, et al. Intra-amniotic infection and sterile intra-amniotic inflammation in women with preterm labor with intact membranes are associated with a higher rate of Ureaplasma species DNA presence in the cervical fluid. J Matern Fetal Neonatal Med 2022;35:7344-52.
274. Motomura K, Romero R, Xu Y, et al. Intra-amniotic infection with ureaplasma parvum causes preterm birth and neonatal mortality that are prevented by treatment with clarithromycin. mBio 2020;11:e00797-20.
275. Sweeney EL, Dando SJ, Kallapur SG, Knox CL. The human ureaplasma species as causative agents of chorioamnionitis. Clin Microbiol Rev 2017;30:349-79.
276. Miralles R, Hodge R, McParland PC, et al. Relationship between antenatal inflammation and antenatal infection identified by detection of microbial genes by polymerase chain reaction. Pediatr Res 2005;57:570-7.
277. Yoon BH, Romero R, Kim M, et al. Clinical implications of detection of Ureaplasma urealyticum in the amniotic cavity with the polymerase chain reaction. Am J Obstet Gynecol 2000;183:1130-7.
278. Šket T, Ramuta TŽ, Starčič Erjavec M, Kreft ME. The role of innate immune system in the human amniotic membrane and human amniotic fluid in protection against intra-amniotic infections and inflammation. Front Immunol 2021;12:735324.
279. Santos P, Almeida F. Exosome-based vaccines: history, current state, and clinical trials. Front Immunol 2021;12:711565.
280. Pordanjani PM, Bolhassani A, Milani A, Pouriayevali MH. Extracellular vesicles in vaccine development and therapeutic approaches for viral diseases. Process Biochem 2023;128:167-80.
281. Kalluri R, LeBleu VS. The biology, function, and biomedical applications of exosomes. Science 2020;367:eaau6977.
282. Li Q, Zhou G, Fei X, Tian Y, Wang S, Shi H. Engineered bacterial outer membrane vesicles with lipidated heterologous antigen as an adjuvant-free vaccine platform for streptococcus suis. Appl Environ Microbiol 2023;89:e0204722.
283. Liu H, Zhang Q, Wang S, Weng W, Jing Y, Su J. Bacterial extracellular vesicles as bioactive nanocarriers for drug delivery: advances and perspectives. Bioact Mater 2022;14:169-81.
284. Xunian Z, Kalluri R. Biology and therapeutic potential of mesenchymal stem cell-derived exosomes. Cancer Sci 2020;111:3100-10.
285. Kacerovsky M, Stranik J, Matulova J, et al. Clinical characteristics of colonization of the amniotic cavity in women with preterm prelabor rupture of membranes, a retrospective study. Sci Rep 2022;12:5062.
286. Dagnelie MA, Corvec S, Khammari A, Dréno B. Bacterial extracellular vesicles: a new way to decipher host-microbiota communications in inflammatory dermatoses. Exp Dermatol 2020;29:22-8.
287. Liu Z, Liu S, Shu Y, et al. Severe bordetella pertussis infection and vaccine issue in Chongqing, from 2012 to 2018. Int J Infect Dis 2019;84:102-8.
288. Mehanny M, Koch M, Lehr CM, Fuhrmann G. Streptococcal extracellular membrane vesicles are rapidly internalized by immune cells and alter their cytokine release. Front Immunol 2020;11:80.
289. Amabebe E, Anumba DO. Diabetogenically beneficial gut microbiota alterations in third trimester of pregnancy. Reprod Fertil 2021;2:R1-R12.
290. Amabebe E, Ikumi N, Pillay K, Matjila M, Anumba D. Maternal obesity-related placental dysfunction: from peri-conception to late gestation. PRM 2023;2:Online ahead of print.
291. Thaxton JE, Romero R, Sharma S. TLR9 activation coupled to IL-10 deficiency induces adverse pregnancy outcomes. J Immunol 2009;183:1144-54.
292. Scharfe-Nugent A, Corr SC, Carpenter SB, et al. TLR9 provokes inflammation in response to fetal DNA: mechanism for fetal loss in preterm birth and preeclampsia. J Immunol 2012;188:5706-12.
293. van Boeckel SR, Davidson DJ, Norman JE, Stock SJ. Cell-free fetal DNA and spontaneous preterm birth. Reproduction 2018;155:R137-45.
294. Sun Y, Qin X, Shan B, et al. Differential effects of the CpG-Toll-like receptor 9 axis on pregnancy outcome in nonobese diabetic mice and wild-type controls. Fertil Steril 2013;99:1759-67.
295. Liu Z, Tang Z, Li J, Yang Y. Effects of placental inflammation on neonatal outcome in preterm infants. Pediatr Neonatol 2014;55:35-40.
296. Kim MA, Lee YS, Seo K. Assessment of predictive markers for placental inflammatory response in preterm births. PLoS One 2014;9:e107880.
297. Kim CJ, Romero R, Chaemsaithong P, Chaiyasit N, Yoon BH, Kim YM. Acute chorioamnionitis and funisitis: definition, pathologic features, and clinical significance. Am J Obstet Gynecol 2015;213:S29-52.
298. Kemp MW. Preterm birth, intrauterine infection, and fetal inflammation. Front Immunol 2014;5:574.
299. Goldstein JA, Gallagher K, Beck C, Kumar R, Gernand AD. Maternal-fetal inflammation in the placenta and the developmental origins of health and disease. Front Immunol 2020;11:531543.
300. Hantoushzadeh S, Anvari Aliabad R, Norooznezhad AH. Antibiotics, inflammation, and preterm labor: a missed conclusion. J Inflamm Res 2020;13:245-54.
301. McClure EM, Goldenberg RL. Use of antibiotics to reduce preterm birth. Lancet Glob Health 2019;7:e18-9.
302. Möhrmann L, Huang HJ, Hong DS, et al. Liquid biopsies using plasma exosomal nucleic acids and plasma cell-free DNA compared with clinical outcomes of patients with advanced cancers. Clin Cancer Res 2018;24:181-8.
303. Wan Y, Liu B, Lei H, et al. Nanoscale extracellular vesicle-derived DNA is superior to circulating cell-free DNA for mutation detection in early-stage non-small-cell lung cancer. Ann Oncol 2018;29:2379-83.
304. Keserű JS, Soltész B, Lukács J, et al. Detection of cell-free, exosomal and whole blood mitochondrial DNA copy number in plasma or whole blood of patients with serous epithelial ovarian cancer. J Biotechnol 2019;298:76-81.
305. Kim DJ, Yang J, Seo H, Lee WH, Ho Lee D, et al. Colorectal cancer diagnostic model utilizing metagenomic and metabolomic data of stool microbial extracellular vesicles. Sci Rep 2020;10:2860.
306. Ramos BRA, Tronco JA, Carvalho M, et al. Circulating extracellular vesicles microRNAs are altered in women undergoing preterm birth. Int J Mol Sci 2023;24:5527.
307. Zhao Q, Ma Z, Wang X, et al. Lipidomic biomarkers of extracellular vesicles for the prediction of preterm birth in the early second trimester. J Proteome Res 2020;19:4104-13.
308. Kammala AK, Mosebarger A, Radnaa E, et al. Extracellular vesicles-mediated recombinant IL-10 protects against ascending infection-associated preterm birth by reducing fetal inflammatory response. Front Immunol 2023;14:1196453.
309. Gilmore WJ, Johnston EL, Bitto NJ, et al. Bacteroides fragilis outer membrane vesicles preferentially activate innate immune receptors compared to their parent bacteria. Front Immunol 2022;13:970725.
310. Hadley EE, Sheller-Miller S, Saade G, et al. Amnion epithelial cell-derived exosomes induce inflammatory changes in uterine cells. Am J Obstet Gynecol 2018;219:478.e1-478.e21.
311. Han C, Han L, Huang P, Chen Y, Wang Y, Xue F. Syncytiotrophoblast-derived extracellular vesicles in pathophysiology of preeclampsia. Front Physiol 2019;10:1236.
312. Han C, Wang C, Chen Y, et al. Placenta-derived extracellular vesicles induce preeclampsia in mouse models. Haematologica 2020;105:1686-94.
313. Cooke WR, Jones GD, Redman CW, Vatish M. Syncytiotrophoblast derived extracellular vesicles in relation to preeclampsia. Maternal-Fetal Medicine 2021;3:151-60.
314. Powell JS, Gandley RE, Lackner E, et al. Small extracellular vesicles from plasma of women with preeclampsia increase myogenic tone and decrease endothelium-dependent relaxation of mouse mesenteric arteries. Pregnancy Hypertens 2022;28:66-73.
315. Jarosik GP, Land CB, Duhon P, Chandler R Jr, Mercer T. Acquisition of iron by Gardnerella vaginalis. Infect Immun 1998;66:5041-7.
316. Jarosik GP, Land CB. Identification of a human lactoferrin-binding protein in Gardnerella vaginalis. Infect Immun 2000;68:3443-7.
317. Khan S, Voordouw MJ, Hill JE. Competition among Gardnerella subgroups from the human vaginal microbiome. Front Cell Infect Microbiol 2019;9:374.
318. Ñahui Palomino RA, Zicari S, Vanpouille C, Vitali B, Margolis L. Vaginal lactobacillus inhibits HIV-1 replication in human tissues ex vivo. Front Microbiol 2017;8:906.
319. Costantini PE, Vanpouille C, Firrincieli A, Cappelletti M, Margolis L, Ñahui Palomino RA. Extracellular vesicles generated by gram-positive bacteria protect human tissues ex vivo from HIV-1 infection. Front Cell Infect Microbiol 2021;11:822882.
320. Gilmore WJ, Bitto NJ, Kaparakis-liaskos M. Pathogenesis mediated by bacterial membrane vesicles. In: Mathivanan S, Fonseka P, Nedeva C, Atukorala I, editors. New Frontiers: Extracellular Vesicles. Cham: Springer International Publishing; 2021. pp. 101-50.
321. Gilmore WJ, Johnston EL, Zavan L, Bitto NJ, Kaparakis-Liaskos M. Immunomodulatory roles and novel applications of bacterial membrane vesicles. Mol Immunol 2021;134:72-85.
322. Huang W, Yao Y, Long Q, et al. Immunization against multidrug-resistant Acinetobacter baumannii effectively protects mice in both pneumonia and sepsis models. PLoS One 2014;9:e100727.
323. Raeven RH, Brummelman J, Pennings JL, et al. Bordetella pertussis outer membrane vesicle vaccine confers equal efficacy in mice with milder inflammatory responses compared to a whole-cell vaccine. Sci Rep 2016;6:38240.
325. Chen CY, Rao SS, Yue T, et al. Glucocorticoid-induced loss of beneficial gut bacterial extracellular vesicles is associated with the pathogenesis of osteonecrosis. Sci Adv 2022;8:eabg8335.
326. Liu JH, Chen CY, Liu ZZ, et al. Extracellular vesicles from child gut microbiota enter into bone to preserve bone mass and strength. Adv Sc 2021;8:2004831.
327. Díez-Sainz E, Milagro FI, Riezu-Boj JI, Lorente-Cebrián S. Effects of gut microbiota-derived extracellular vesicles on obesity and diabetes and their potential modulation through diet. J Physiol Biochem 2022;78:485-99.
328. Chelakkot C, Choi Y, Kim DK, et al. Akkermansia muciniphila-derived extracellular vesicles influence gut permeability through the regulation of tight junctions. Exp Mol Med 2018;50:e450.
Cite This Article
Export citation file: BibTeX | EndNote | RIS
OAE Style
Amabebe E, Kumar A, Tatiparthy M, Kammala AK, Taylor BD, Menon R. Cargo exchange between human and bacterial extracellular vesicles in gestational tissues: a new paradigm in communication and immune development. Extracell Vesicles Circ Nucleic Acids 2024;5:297-328. http://dx.doi.org/10.20517/evcna.2024.21
AMA Style
Amabebe E, Kumar A, Tatiparthy M, Kammala AK, Taylor BD, Menon R. Cargo exchange between human and bacterial extracellular vesicles in gestational tissues: a new paradigm in communication and immune development. Extracellular Vesicles and Circulating Nucleic Acids. 2024; 5(2): 297-328. http://dx.doi.org/10.20517/evcna.2024.21
Chicago/Turabian Style
Emmanuel Amabebe, Awanit Kumar, Madhuri Tatiparthy, Ananth Kumar Kammala, Brandie D. Taylor, Ramkumar Menon. 2024. "Cargo exchange between human and bacterial extracellular vesicles in gestational tissues: a new paradigm in communication and immune development" Extracellular Vesicles and Circulating Nucleic Acids. 5, no.2: 297-328. http://dx.doi.org/10.20517/evcna.2024.21
ACS Style
Amabebe, E.; Kumar A.; Tatiparthy M.; Kammala AK.; Taylor BD.; Menon R. Cargo exchange between human and bacterial extracellular vesicles in gestational tissues: a new paradigm in communication and immune development. Extracell. Vesicles. Circ. Nucleic. Acids. 2024, 5, 297-328. http://dx.doi.org/10.20517/evcna.2024.21
About This Article
Copyright
Data & Comments
Data
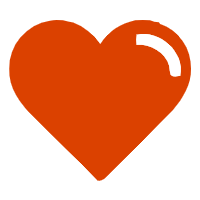
Comments
Comments must be written in English. Spam, offensive content, impersonation, and private information will not be permitted. If any comment is reported and identified as inappropriate content by OAE staff, the comment will be removed without notice. If you have any queries or need any help, please contact us at support@oaepublish.com.