Properties and modulation strategies of ZnIn2S4 for photoelectrochemical water splitting: opportunities and prospects
Abstract
The global energy crisis has driven significant research into renewable energy sources, and photoelectrochemical (PEC) water splitting stands out as one of the most promising solutions for hydrogen production. Among the various materials developed for PEC water splitting, ternary metal sulfides, particularly ZnIn2S4, have garnered considerable attention due to their unique combination of electronic, optical, and chemical properties. This paper presents a comprehensive analysis of the structure and properties of ZnIn2S4, explores modification strategies for ZnIn2S4-based photoanodes, and discusses their application in PEC water splitting. Furthermore, we address the challenges and limitations of ZnIn2S4-based photoanodes and highlight prospects for their future development.
Keywords
INTRODUCTION
The global energy landscape is increasingly characterized by a precarious balance between growing energy demand and the urgent need to mitigate environmental degradation. The extensive reliance on fossil fuels has been central to human development, facilitating unprecedented economic growth and technological advancements[1,2]. However, this advancement has come at a significant environmental cost, contributing to severe issues such as air and water pollution, greenhouse gas emissions, and climate change[3]. These challenges have spurred a global call for sustainable and renewable energy sources that can meet growing energy needs without exacerbating environmental problems. Among various renewable energy alternatives, solar energy stands out as the most abundant and widely distributed resource[4]. The sun delivers an immense amount of energy to Earth’s surface, far exceeding the current global energy consumption. Harnessing even a fraction of this energy could effectively address the global energy crisis. Solar energy conversion technologies, such as photovoltaics, solar thermal systems, and photoelectrochemical (PEC) water splitting, offer promising pathways to sustainable energy[5]. Among these, PEC water splitting has garnered significant attention for its potential to produce hydrogen-a clean and efficient energy
PEC water splitting combines the principles of photovoltaics and electrochemistry to generate hydrogen from water using solar energy [Figure 1][7]. The process begins with the absorption of sunlight by a semiconductor material, which generates electron-hole pairs[8]. These charge carriers then participate in redox reactions at the surface of the photoelectrode, resulting in the splitting of water molecules into hydrogen and oxygen. The overall reaction can be divided into two half-reactions: the oxygen evolution reaction (OER) at the photoanode and the hydrogen evolution reaction (HER) at the cathode[9]. The OER, a four-electron process, is particularly challenging due to its sluggish kinetics and the high overpotential (η) required to drive the reaction[10]. Consequently, the development of efficient and stable photoanode materials is critical to the advancement of PEC water-splitting technology. The ideal photoanode material should possess several key attributes: a suitable bandgap for visible light absorption, appropriate band edge positions for driving the OER, high charge carrier mobility, and excellent stability in aqueous environments. Over the years, numerous materials have been explored as potential photoanodes for PEC water splitting, including titanium dioxide (TiO2)[11], tungsten trioxide (WO3)[12], bismuth vanadate (BiVO4)[13], iron oxide (Fe2O3)[14], and more recently, various metal sulfides and oxides. Among these, titanium dioxide has been the most extensively studied due to its excellent stability, low cost, and abundance. However, its wide bandgap (3.2 eV) limits its light absorption to the ultraviolet (UV) region, which constitutes only a small fraction of the solar spectrum. This limitation, combined with the high recombination rate of photogenerated charge carriers, has led to the search for alternative materials with enhanced light absorption and improved charge carrier dynamics[15-17].
Figure 1. Schematic diagram of PEC water splitting[7]. Copyright 2019, Wiley-VCH. PEC: Photoelectrochemical.
In recent years, the ternary metal sulfide ZnIn2S4 has emerged as a highly promising candidate for PEC photoanodes[2,3]. ZnIn2S4 possesses a direct bandgap in the range of 2.4-2.7 eV, making it capable of absorbing a significant portion of visible light. This material exhibits a layered structure, which not only enhances its surface area but also provides ample pathways for efficient charge carrier transport. The combination of these properties allows ZnIn2S4 to achieve higher solar energy conversion efficiencies compared to traditional photoanode materials[16]. The working mechanism of ZnIn2S4 as a photoanode involves several key processes. When illuminated, ZnIn2S4 absorbs photons, generating electron-hole pairs. The conduction band electrons are then transferred to the external circuit, driving the reduction reactions, while the valence band holes migrate to the surface, where they participate in the oxidation reaction. Additionally, ZnIn2S4 is relatively non-toxic, abundant, and cost-effective, making it an attractive alternative to other metal sulfides such as cadmium sulfide (CdS), which suffers from toxicity issues[18]. Despite its promising characteristics, ZnIn2S4-based photoanodes face several challenges that limit their practical application in PEC water splitting. One of the primary issues is the rapid recombination of photogenerated electron-hole pairs, which reduces the overall efficiency of the water-splitting process. Furthermore, the slow kinetics of the surface hole oxidation reaction in ZnIn2S4 contributes to a high η, further diminishing the device’s performance[19]. To address these challenges, researchers have explored various modification strategies aimed at enhancing the charge carrier dynamics and surface reactivity of ZnIn2S4 photoanodes.
Modification approaches such as metal doping, heterojunction construction, and surface passivation have shown significant promise in improving the performance of ZnIn2S4 photoanodes[20-22]. Metal doping involves introducing foreign atoms into the ZnIn2S4 lattice to modify its electronic structure, thereby improving its light absorption properties and charge carrier mobility[23]. Heterojunction construction, on the other hand, involves coupling ZnIn2S4 with other semiconductors to form a junction with favorable band alignment[24], which facilitates charge separation and reduces recombination losses. Surface passivation techniques aim to mitigate surface states that act as recombination centers, thereby improving the charge transfer efficiency at the photoanode/electrolyte interface. These modification strategies have yielded notable improvements in the PEC performance of ZnIn2S4-based photoanodes[25]. However, the field still lacks a comprehensive review that systematically examines the progress made in this area. Such a review is crucial to consolidate the existing knowledge, identify the remaining challenges, and guide future research efforts. This paper seeks to address this gap by providing a detailed overview of recent advances in
BASICS OF ZNIN2S4 AND ADVANTAGES OF ZNIN2S4 AS A PEC PHOTOANODE
Crystal structures and their properties
ZnIn2S4 exists in three main crystal structures, each exhibiting distinct properties that influence their photocatalytic and PEC performance[26] [Figure 3]. In the cubic ZnIn2S4 structure, sulfur atoms are arranged in an ABC stacking pattern, forming a direct cubic spinel phase. In this structure, zinc (Zn) atoms are tetrahedrally coordinated with sulfur (S) atoms, while indium (In) atoms are octahedrally coordinated with S atoms. This coordination results in a stable structure that facilitates efficient charge transport. Although the cubic form is not as efficient as the hexagonal form, it still demonstrates reasonable photocatalytic activity. The hexagonal ZnIn2S4 structure is considered the most efficient for photocatalytic applications[27]. It features a unique atomic alignment along the c-axis, with a repeating sequence of S-In-S-In-S-Zn-S[27,28]. In this structure, Zn and In atoms are coordinated in tetrahedral and octahedral arrangements with S atoms, respectively. The hexagonal form is particularly effective in charge separation and transport, which significantly contributes to its superior photocatalytic performance. Within the hexagonal structure, stacking variations lead to different polytypes, ranging from the 1H polytype (with one packet per unit cell) to the 24R polytype (with up to 24 packets per unit cell). These polytype variations influence the electronic properties and light absorption efficiency of the material. In the less commonly studied trigonal ZnIn2S4 structure, sulfur atoms are tetrahedrally coordinated with zinc atoms and either tetrahedrally or octahedrally coordinated with indium atoms. This polymorph features strong Zn-S and In-S bonds, while the S-S bonds are relatively weaker, which imparts distinctive photocatalytic characteristics. However, due to its lower efficiency compared to the hexagonal form, research on the trigonal variant has been limited[29].
Figure 3. (A) The crystal structure of cubic ZnIn2S4; (B) the crystal structure of hexagonal ZnIn2S4; (C) the crystal structure of triangular ZnIn2S4; and (D) XRD patterns of different ZnIn2S4 structures[26]. Copyright 2021, Elsevier. XRD: X-ray Diffraction.
To summarize, the crystal structure of ZnIn2S4 plays a crucial role in determining its photocatalytic and PEC performance. The cubic structure, while stable and efficient in charge transport, shows moderate photocatalytic activity[15]. The hexagonal structure, with its superior charge separation and transport capabilities, stands out as the most efficient for photocatalytic applications. The trigonal structure, despite being less efficient, provides unique properties that could be valuable in niche applications. Understanding these structural variations helps in tailoring ZnIn2S4 for specific applications, ensuring optimal performance[30-33].
Synthesis methods for ZnIn2S4
The synthesis of ZnIn2S4 is a critical step in optimizing its properties for photocatalytic and PEC applications. The choice of synthesis methods significantly influences the material’s morphology, crystallinity, phase composition, and ultimately, its performance. Several synthesis techniques have been developed, each offering unique advantages in tailoring the properties of ZnIn2S4 for specific applications.
Hydrothermal/Solvothermal synthesis
Hydrothermal and solvothermal synthesis methods are among the most widely used techniques for producing ZnIn2S4[19,34]. These methods involve reacting metal precursors, such as zinc and indium salts, with sulfur sources in high-temperature, high-pressure aqueous (hydrothermal) or non-aqueous (solvothermal) environments. The primary advantage of these methods lies in their ability to control the growth of ZnIn2S4 crystals by adjusting parameters such as temperature, pressure, reaction time, and the type of solvent used. One of the key benefits of hydrothermal/solvothermal synthesis is the production of ZnIn2S4 with highly uniform morphologies, such as nanospheres[35-37], nanosheets[38,39], or nanoflowers[40,41]. These structures enhance the material’s light absorption and charge transport properties, both of which are critical for efficient photocatalysis and PEC processes. For example, nanosheets provide a large surface area and short diffusion paths for charge carriers, which reduce recombination rates and improve photocatalytic efficiency[42]. Furthermore, these methods allow for fine-tuning of the crystallinity and phase composition, ensuring the formation of the desired hexagonal phase of ZnIn2S4, known for its superior photocatalytic activity. Additionally, hydrothermal/solvothermal synthesis is relatively straightforward and scalable, making it suitable for large-scale production of ZnIn2S4. The process can be carried out at relatively low temperatures, which helps preserve the material’s stability and reduce energy consumption.
Chemical vapor deposition
Chemical Vapor Deposition (CVD) is another effective method for synthesizing ZnIn2S4, particularly for applications requiring thin films with excellent surface coverage and crystallinity[28,43,44]. In the CVD process, gaseous precursors containing zinc, indium, and sulfur are introduced into a reactor, where they decompose on a heated substrate, resulting in the formation of ZnIn2S4 films. The temperature, pressure, and flow rate of the gases can be precisely controlled, allowing for the deposition of high-quality films with uniform thickness and controlled crystallinity.
One of the main advantages of CVD is its ability to produce ZnIn2S4 films with exceptional uniformity and purity, which is essential for ensuring consistent photocatalytic and PEC performance. The thin films produced by CVD are particularly valuable in applications that require a high surface area-to-volume ratio, such as in PEC cells. Furthermore, CVD allows for the deposition of ZnIn2S4 on various substrates, including glass, silicon, and conductive oxides, making it a versatile technique for integrating ZnIn2S4 into different device architectures.
Microwave-assisted synthesis
Microwave-assisted synthesis is an emerging technique for producing ZnIn2S4, known for its rapid reaction times and energy efficiency. In this method, microwave radiation is used to rapidly heat the reactants, leading to the quick formation of ZnIn2S4 with unique nanostructures[37,45-49]. The use of microwave energy accelerates the reaction kinetics, significantly reducing the synthesis time compared to conventional heating methods. One of the key benefits of microwave-assisted synthesis is the ability to produce ZnIn2S4 with a high surface area and enhanced light absorption properties. The rapid heating and cooling cycles associated with microwave synthesis often result in the formation of nanostructures, such as nanospheres, nanorods, or hierarchical nanostructures, which can improve the material’s photocatalytic activity. These nanostructures provide a larger active surface area for photocatalytic reactions and better light-harvesting capabilities, both of which are crucial for enhancing the overall efficiency of PEC devices. Additionally, microwave-assisted synthesis is considered an energy-saving method due to its reduced reaction times and lower energy consumption compared to traditional heating methods. The process is also highly reproducible, making it a suitable option for the large-scale production of ZnIn2S4.
Other synthesis methods
Apart from the above-mentioned techniques, other synthesis methods such as solvothermal microwave synthesis, co-precipitation[50,51], and solid-state reactions[52,53] have also been explored for the production of ZnIn2S4. Each of these methods offers distinct advantages in terms of controlling the material’s morphology, crystallinity, and phase composition. For example, solvothermal microwave synthesis combines the benefits of solvothermal and microwave-assisted methods[37], allowing for the rapid production of ZnIn2S4 with controlled nanostructures and enhanced photocatalytic properties. Co-precipitation, on the other hand, is a simple and cost-effective method that involves the simultaneous precipitation of zinc, indium, and sulfur precursors, leading to the formation of ZnIn2S4. Although this method may result in products with lower crystallinity, post-synthesis treatments, such as annealing, can be employed to improve the material’s crystallinity and phase purity.
Advantages of ZnIn2S4 as a PEC photoanode
ZnIn2S4 has gained considerable attention as a promising material for PEC photoanodes due to several inherent advantages that make it well-suited for solar energy conversion and water-splitting applications[2,3,18,26,54]. One of its key strengths is its appropriate bandgap of approximately 2.3 eV. This direct bandgap is particularly beneficial because it allows ZnIn2S4 to efficiently absorb visible light, capturing a substantial portion of the solar spectrum. By harnessing this broad range of solar energy, ZnIn2S4 can effectively convert sunlight into electrical energy, which is crucial for PEC applications focused on sustainable energy production. The layered structure of ZnIn2S4 is another notable feature that contributes to its effectiveness as a PEC photoanode. This structure facilitates efficient charge separation by minimizing the recombination of photogenerated electron-hole pairs[27]. In PEC processes, the separation of these charges is critical for maximizing the photocurrent and overall efficiency of the device. The layered arrangement in ZnIn2S4 helps to create a favorable pathway for charge carriers, ensuring that more electrons and holes reach the respective electrodes without recombining, which would otherwise reduce the efficiency of the water-splitting reaction[55-58]. Among the various crystal polymorphs of ZnIn2S4, the hexagonal form stands out for its high photocatalytic activity[27]. This superior performance is attributed to the hexagonal structure’s favorable electronic properties, which enhance charge transport and reduce recombination losses[59-62]. The hexagonal ZnIn2S4, with its optimized electronic structure, demonstrates an exceptional ability to drive PEC water splitting and other photocatalytic processes, making it an ideal candidate for applications requiring efficient and robust solar energy conversion.
Stability and durability are crucial considerations for any material used in PEC cells, and ZnIn2S4 excels in this regard. It is chemically stable in aqueous environments, ensuring its long-term functionality under operational conditions. This stability is vital for sustained performance in PEC devices, where materials are exposed to water and sunlight over extended periods[15]. Resistance of ZnIn2S4 to corrosion and degradation further enhances its reliability as a photoanode material, making it suitable for practical, large-scale applications in solar fuel production[63-65]. Another significant advantage of ZnIn2S4 is its versatility in doping, which allows for the fine-tuning of its electronic and optical properties[66,67]. Doping ZnIn2S4 with various metal and non-metal elements, such as cadmium (Cd)[21] or magnesium (Mg)[42], can enhance its charge separation efficiency, reduce recombination, and optimize its overall PEC performance. These doped versions of ZnIn2S4 have been shown in studies to exhibit improved photocatalytic efficiency, making them even more effective in driving the PEC water-splitting process. The ability to tailor the properties of ZnIn2S4 through doping adds a layer of flexibility in designing high-performance photoanodes for specific applications. From an economic perspective, ZnIn2S4 offers cost-effectiveness that makes it attractive for widespread adoption in PEC applications. The material is relatively inexpensive compared to other semiconductors commonly used in PEC cells, and its synthesis is straightforward, enabling easy scale-up for industrial production. This affordability, combined with its high efficiency, positions ZnIn2S4 as a practical option for large-scale solar energy conversion projects aimed at reducing the cost of sustainable energy technologies. Finally, ZnIn2S4 is an environmentally friendly material, further enhancing its appeal in green energy technologies. Its environmental benignity ensures that its use in PEC applications does not contribute to pollution or ecological harm, aligning with the global shift toward more sustainable and
In summary, the suitable bandgap, layered structure, high photocatalytic activity, stability, versatility in doping, cost-effectiveness, and environmental friendliness of ZnIn2S4 collectively make it an exceptional material for PEC photoanodes. These advantages not only enhance its performance in solar energy conversion but also ensure that it can be deployed in a sustainable and economically viable manner, positioning ZnIn2S4 as a key player in the future of renewable energy technologies.
MODIFICATION METHODS FOR ZNIN2S4-BASED PHOTOANODES AND APPLICATION IN PEC WATER SPLITTING
Although ZnIn2S4 shows significant promise for PEC water splitting, its overall performance is hindered by the rapid recombination of photogenerated charge carriers and the inefficient transfer of photogenerated electron-hole pairs. In practical PEC applications, the reaction kinetics and hydrogen/oxygen evolution selectivity may fall short of meeting specific efficiency requirements. Under extreme conditions, such as high temperatures, strong acids, or strong alkalis, ZnIn2S4 could experience structural degradation or performance deterioration, affecting its long-term stability and operational reliability. Therefore, to enhance the PEC performance of ZnIn2S4, suitable modification strategies, such as element doping, heterostructure construction, and surface modification [Table 1], are crucial. The following sections will discuss how these three approaches can improve the PEC water-splitting performance of ZnIn2S4.
A summary of the reported performance of ZIS-based photoanodes
Photoanodes | Synthetic method | Maximum current density | (Applied bias photon-to-current efficiency) ABPE | (Incident photon-to-current efficiency) IPCE | Cocatalyst | Electrolyte | Light source | Ref(year) |
MWCNT/H-ZnIn2S4 | Hydrothermal | 224 μA/cm2(0.8 V vs. Ag/AgCl) | --- | 8.06% (430 nm) | --- | 0.5 M Na2SO4 | 100 W halogen lamp (100 mW/cm2) | [68] |
Co-Pi/ZnIn2S4/Pt | Hydrothermal method electrochemical deposition photo-assisted electrodeposition | 0.91 mA/cm2 (1.23 V vs. RHE) | --- | 11% (370 nm) | Co-Pi catalyst and Pt NPs | 0.25 M Na2SO3 Na2S | Xe lamp | [69] |
ZnIn2S4:MA | Hydrothermal method, low-temperature water bath method and heat treatment | 4.91 mA/cm2 (1.23 V vs. RHE) | 1.61% | --- | --- | 0.5 M Na2SO4 (pH≈6.8) | Xe lamp (325 W) | [42] |
Cd-ZIS | One-step solvothermal method | 5.1 mA/cm2 (1.23 V vs. RHE) | 3.00% | 48% (355 nm) | --- | 1 M Na2SO4 and 0.5 M Na2SO3 (pH=9.6) | Xe lamp (100 mW/cm2) | [21] |
FO/MQDs/ZIS | Hydrothermal method | 2.62 mA/cm2 (1.23 V vs. RHE) | 0.87% | 15% (440 nm) | --- | 0.5 M Na2SO4 (pH=6.8) | 300 W Xe lamp (100 mW/cm2) | [70] |
Highly exposed (110) | Hydrothermal method | 0.36 mA/cm2 (1.23 V vs. RHE) | 0.10% | --- | --- | 0.2 M Na2SO4 (pH≈6.8) | Xe lamp (100 mW/cm2) | [71] |
ZnIn2S4-O-TS | Hydrothermal method | 4.57 mA/cm2 (1.23 V vs. RHE) | 1.67% | --- | ---- | 0.5 M Na2SO4 | 325 W Xe lamp (100 mW/cm2) | [72] |
2D H@R-ZnIn2S4 | In-situ solvothermal method | 3.1 mA/cm2 (1.2 V vs. RHE) | 2.65% | 40% | --- | 0.5 M Na2SO4 and | Xe lamp (100 mW/cm2) | [73] |
H-ZnIn2S4/CN | Hydrothermal method facile chemisorption method | 110 μA/cm2(0.8 V vs. Ag/AgCl) | --- | 8.52% (430 nm) | --- | 0.5 M Na2SO4 | 100 W halogen lamp (100 mW/cm2) | [74] |
Sb2S3/ZnIn2S4/Cu2S | Hydrothermal method and submerging method | 2.81 mA/cm2 (1.23 V vs. RHE) | 23.46% | 21.09% | --- | 0.2 M Na2SO4 (pH=5.75) | Xe lamp (100 mW/cm2) | [75] |
ZnIn2S4/Au/PB | Hydrothermal method and electrochemical method | 0.42 mA/cm2 (1.23 V vs. RHE) | --- | 24.6% (350 nm) | --- | 0.1 M Na2SO4 | 300 W Xe lamp (100 mW/cm2) | [76] |
TiO2/ZnIn2S4/Co-Pi | Hydrothermal method, annealing method and photo-assisted electrodeposition | 5.05 mA/cm2 (1.23 V vs. RHE) | 2.90% | --- | Co-Pi | 1 M KOH (pH = 13.6) | 300 W Xe lamp (100 mW/cm2) | [22] |
Sv-ZnIn2S4/ N-TiO2 | Solvothermal method | 4.9 mA/cm2 (1.23 V vs. RHE) | 0.48% | 57.9% (350 nm) | --- | 0.5 M Na2SO4 | 300 W Xe lamp (100 mW/cm2) | [25] |
Ni-doped ZnIn2S4 | One-pot hydrothermal method | --- | --- | --- | --- | 0.2 M Na2SO4 | 300 W Xe lamp (100 mW/cm2) | [77] |
SiO2/ZnIn2S4/TiO2 (ZT) | Hydrothermal method, dipping method and annealing method | 0.786 mA/cm2 (0.1 V vs. Ag/AgCl) | 0.90% | --- | --- | 0.1 M Na2S and | --- | [20] |
Hexagonal phase/cubic phase homogeneous | Two-step hydrothermal method | 0.53 mA/cm2 (1.23 V vs. RHE) | --- | --- | ---- | 2.4 M Na2S and 1.5 M Na2SO3 | 500 W Xe lamp (100 mW/cm2) | [78] |
Mg:ZnIn2S4/Co:ZnIn2S4 | Hydrothermal method | 0.91 mA/cm2 (1.23V vs. RHE) | 0.13% | --- | Co2+ | 0.2 M Na2SO4 (pH=6.8) | --- | [79] |
n-ZnIn2S4-p | Hydrothermal method and heat treatment | 6.34 mA/cm2 (1.23 V vs. RHE) | 72% (350 nm) | --- | 0.5 M Na2SO4 | 450 W Xe lamp (100 mW/cm2) | [80] | |
ZnIn2S4-O-S | Hydrothermal method and facile air annealing | 3.52 mA/cm2 (1.23 V vs. RHE) | 1.70% | --- | --- | 0.5 M Na2HPO4/ | 325 W Xe lamp (100 mW/cm2) | [81] |
Metal/non-metal doping
Element doping is a technique that involves introducing external atomic elements into the crystal structure through physical or chemical means, which may create defects or alter the crystal type. This process can adjust the band structure and catalytic performance of the catalyst[82,83]. Doping can be categorized into two types: metal doping[84] and non-metal doping[85], each with distinct effects and mechanisms. Metal doping typically occurs throughout the bulk structure with adjustable contents and types, while non-metal doping often involves surface chemisorption following structural reconstruction. These differences in doping mechanisms significantly influence the electronic properties, charge carrier dynamics, and photocatalytic performance of materials. Below, we will discuss these two doping strategies separately to present their effects more clearly.
Metal doping
Metal doping in ZnIn2S4 is a commonly used strategy to optimize the electronic structure and enhance the separation efficiency of photogenerated carriers. Metal dopants can modify the conduction and valence band positions, create new energy levels, or improve the conductivity of the material. Pan et al.[21] developed an enhanced in-situ growth method to synthesize a two-dimensional (2D) Cd-doped ZnIn2S4 (Cd-ZIS) photoanode with a vertically aligned nanosheet array structure, demonstrating significant improvements in PEC performance. Utilizing the excellent lattice compatibility of Cd2+ ions with the hexagonal ZnIn2S4 (ZIS) crystal structure, Cd dopants were successfully incorporated into the ZIS matrix [Figure 4A]. The substitution of Zn sites by Cd atoms on the 2D ZIS basal planes was confirmed, resulting in the formation of a highly uniform Cd-ZIS nanosheet array [Figure 4B]. Notably, the Cd-doped photoanodes exhibited higher photocurrents at 1.23 VRHE compared to undoped ZIS, with a positive correlation observed between doping concentration and photocurrent up to a Cd concentration of 5%. This suggests that substituting Zn sites with Cd dopants effectively enhances the electrochemical performance of the photoanodes. However, further increasing the Cd concentration to 7% led to a slight decrease in photocurrent, likely due to the formation of unfavorable lattice defects, underscoring the necessity of optimizing defect type and concentration. The optimized 5% Cd-ZIS photoanode achieved a maximum photocurrent of
Figure 4. (A) Fabrication process scheme for ZIS and Cd-ZIS photoanodes. Crystal structure and atomic configuration of ZIS viewed from the (110) plane, and Cd-ZIS viewed from the (110) plane; (B) The top view FESEM images of 5%Cd-ZIS nanosheet array photoanodes; (C) ABPE plots of ZIS and 5%Cd-ZIS; (D) Schematic illustration of the photocarrier dynamics mechanism achieving highly efficient PEC conversion for Cd-ZIS photoanode[21]. Copyright 2024, Wiley-VCH. ZIS: ZnIn2S4; Cd-ZIS: Cd-doped ZnIn2S4; PEC: photoelectrochemical; ABPE: applied bias photon-to-current efficiency; FESEM: field emission scanning electron microscope.
In another study, Huang et al.[42] employed a hydrothermal method to dope Mg2+ into ZIS nanosheets (referred to as ZIS:Mg), forming a type-II band structure to facilitate charge separation. By using a simple thermal treatment, the doping-induced excess oxygen defects were converted into Mg-O bonds [Figure 5A and B]. Both experimental and theoretical calculations demonstrated that the formation of Mg-O bonds increased the number of surface reaction active sites. This was achieved by shifting the catalytic activity from In or Zn to the Mg-O bonds. This enhancement promoted the transfer of photogenerated carriers, reduced carrier recombination, and lowered the OER η. Compared to the reversible hydrogen electrode (VRHE), the photocurrent (J) of this photoanode significantly increased to 4.91 mA/cm2 at 1.23 V, and the onset potential (Von) shifted from 0.28 to -0.06 VRHE. This performance is comparable to or even better than other recently reported ZIS-based photoanodes. By employing N2 annealing techniques, the defects were reused to achieve efficient PEC water splitting. The significant enhancement in ZIS:Mg performance can be attributed to several key factors: First, the Mg2+ doping effectively adjusted the energy level structure of ZIS, increasing the band bending, which in turn promoted more efficient bulk charge transfer. Second, converting the excess oxygen defects generated during Mg doping into Mg-O bonds not only reduced harmful defects but also achieved the active utilization of these defects, effectively suppressing the recombination of surface electron-hole pairs. Finally, the annealing treatment enabled the Mg-O bonds to become surface active centers, optimizing the distribution of catalytic active sites and reducing the η by facilitating the transfer of surface holes from In or Zn to Mg-O. Under operational conditions at 1.23 VRHE, the photocurrent reached 4.91 mA/cm2, demonstrating the substantial potential of these synergistic effects in enhancing PEC performance
Figure 5. (A) Schematic diagram of the synthesis process for the photoanode; (B) Top view SEM images of the ZIS:MA nanosheet arrays; (C) J-V curves and (D) ABPE values for ZIS, ZIS:Mg, and ZIS:MA photoanodes[42]. Copyright 2024, Wiley-VCH. ABPE: Applied bias photon-to-current efficiency; ZIS: ZnIn2S4; J-V: photocurrent density-voltage; SEM: scanning electron microscope.
Non-metal doping
Non-metal doping in ZIS differs from metal doping in that it generally occurs through surface chemisorption after structural reconstruction. Non-metal dopants can modify the electronic structure, increase the light absorption capacity, and promote charge carrier separation. The surface interactions between non-metal dopants and the ZIS surface often lead to the formation of new energy states that help to capture photogenerated carriers, particularly holes, thereby improving the PEC activity. For example, certain non-metal anionic groups, such as phosphate (PO43-), selenate (SeO42-), and sulfate (SO42-), have been explored as potential options to enhance the OER capability. These non-metallic groups can optimize the electronic structure of active sites, reduce the OER η by adjusting the adsorption behavior of reaction intermediates, and promote the transfer of surface carriers. Particularly, the SO42- group is capable of breaking the adsorption energy scaling relationship between OH* and OOH* (OER intermediates), thereby lowering the OER η. For example, Xu et al.[81] successfully introduced SO42- anionic groups in situ into ZIS photoanodes through a combination of hydrothermal and air annealing processes, which also generated bulk and surface sulfur vacancies (Sv). They subsequently used Na2S treatment to remove excess surface Sv without affecting the overall Sv. Experimental results and density functional theory (DFT) calculations confirmed that the SO42- group has a significantly positive impact on reducing the OER η, which helps to reduce the recombination of interface carriers and improve OER activity. The appropriate bulk vacancies and suppressed surface vacancies can promote bulk charge separation and inhibit surface recombination. The study results showed that, compared to the VRHE, the optimized photoanode exhibited a significantly enhanced photocurrent density of 3.52 mA/cm2 at 1.23 VRHE, with the Von measured in 0.5 M Na2HPO4/NaH2PO4 aqueous solution negatively shifted to 0.01 VRHE.
Both metal and non-metal doping strategies offer promising avenues for enhancing the PEC performance of ZIS photoanodes. Metal doping, particularly with Cd and Mg, effectively modulates energy levels, converts defects into active sites, and optimizes surface reactions. Non-metal anionic groups, such as SO42-, similarly enhance OER activity by altering electronic structures and promoting efficient carrier transfer. The combination of these doping strategies can potentially lead to further advancements in PEC water splitting and other solar energy applications. Future research should focus on optimizing doping concentrations, understanding the precise mechanisms by which dopants affect PEC performance, and exploring new dopant materials to continue improving the efficiency and stability of ZIS-based photoanodes.
Heterostructure construction
To address the inherent limitations of unmodified single-semiconductor photocatalysts, such as the rapid recombination of photogenerated electron-hole pairs and low electron utilization, researchers have widely adopted the strategy of constructing heterojunctions by coupling two semiconductor materials[39,86,87]. This approach not only significantly broadens the light response range of the photocatalyst but also accelerates the migration and separation of photogenerated carriers through various transfer pathways, thereby effectively enhancing the photocatalytic activity. Heterojunctions come in various configurations, including Type I, Type II, Type III, and Z-scheme[88]. In recent years, significant progress has been made, especially in constructing heterojunctions between ZIS and other semiconductors. This strategy not only increases the exposure of active sites but also promotes the separation of charge carriers by adjusting the band structure and Fermi level of the semiconductors, thereby creating more efficient and targeted photocatalytic systems.
Constructing heterojunctions is a widely used strategy to enhance the PEC performance of materials. A heterojunction is formed by coupling two different semiconductor materials, which creates a band alignment that facilitates the effective separation of photoexcited electron-hole pairs at the interface, thereby reducing carrier recombination. This results in an increase in photocurrent density and an overall improvement in the PEC performance of the photoelectrode. Typically, a heterojunction consists of a combination of a wide-bandgap material and a narrow-bandgap material. This combination enhances the material’s absorption of visible and even near-infrared light, thereby improving solar energy utilization. Compared to random interfaces, heterojunctions generally feature well-defined interfaces with fewer defects, which contributes to improved charge transfer and separation efficiency[89-92].
Traditional methods for preparing ZIS-based heterojunctions involve combining ZIS with secondary catalysts to form binary heterojunctions. However, the utilization of the band structure is often limited by spatial constraints, resulting in a single internal electric field within the heterojunction. Moreover, the limited light absorption due to the use of a single absorber negatively affects the PEC performance of traditional ZIS-based heterojunctions. To overcome these limitations, researchers have enhanced the spatial utilization of the band structure by adjusting the spatial arrangement of ZIS and introducing multiple light absorbers. The resulting multiple embedded electric fields effectively enhance the PEC performance of
Figure 6. (A) SEM images of TiO2/ZnIn2S4/Co-Pi; (B) Photocurrent density-voltage (J-V) curves; (C) Applied bias photon-to-current efficiency (ABPE) curves; (D) Schematic diagram of the TiO2/ZnIn2S4/Co-Pi photoanode demonstrating the band positions and electron/hole pairs transformation processes[22]. Copyright 2022, Elsevier. SEM: Scanning electron microscope.
Traditional Type-II heterojunctions are effective at separating photogenerated charges; however, this enhanced charge separation efficiency often comes at the expense of reduced redox capabilities. This limitation arises because the photogenerated electrons and holes are primarily localized in energy bands with lower redox potentials, which is unfavorable for PEC water-splitting reactions. To address this issue,
Figure 7. (A) The schematic illustration outlines the fabrication process of the ZnIn2S4/CdS/ZnO photoanode; (B) SEM images offer a close-up view of the ZnIn2S4/CdS/ZnO photoanode, revealing the surface morphology; (C) Cross-sectional SEM images provide insight into the layered structure of the ZnIn2S4/CdS/ZnO photoanode; (D) The diagram compares the charge transfer mechanisms in the traditional Type-II and Z-scheme configurations within the ZnIn2S4/CdS heterojunction during a (5,5-Dimethyl-1-pyrroline N-oxide) DMPO trapping experiment; (E) Schematic illustrates charge carrier migration in the Z-scheme ZnIn2S4/CdS heterojunction, highlighting the influence of the internal electric field; (F) LSV curves demonstrate the enhanced performance of the ZnIn2S4/CdS/ZnO photoanode under varying conditions[93]. Copyright 2021, Wiley-VCH. LSV: Linear sweep voltammetry; SEM: scanning electron microscope.
Recent scientific research has identified homojunctions, formed by combining two different crystal phases of the same semiconductor material, as a highly promising strategy for promoting the effective separation of photogenerated electron-hole pairs and significantly extending carrier lifetime. The unique advantage of this approach lies in the similar electron affinities of the two crystal phases within the homojunction, which results in minimal interface defects and greatly increases the likelihood of forming high-quality interfaces at the junction[31]. Compared to traditional heterojunctions, homojunctions offer more pronounced benefits. Constructing n-n junctions, in particular, has shown significant potential for enhancing the PEC performance of materials[94-96]. Qian et al.[78] designed a homogeneous n-n junction composed of hexagonal and cubic phases of ZIS, achieved by synthesizing the two different crystal phases of ZIS. The close lattice matching between the two phases promotes tight bonding, helping to form an effective interface with fewer defects, thereby improving PEC performance. Additionally, the combination of different phase band structures drives the transport of photogenerated carriers, enhancing the effective separation of these carriers. Experimental results demonstrated that the n-n junction of homogeneous hexagonal/cubic ZIS exhibited 1.36 times and 1.89 times higher performance compared to hexagonal ZIS and cubic ZIS, respectively, achieving a performance of 0.53 mA/cm2 at 1.23 V. Unlike heterojunctions, the homogeneous ZIS junction avoids the introduction of other materials, thereby reducing interface defects to some extent. At the same time, the homojunction inherits the advantages of heterojunctions, accelerating the separation and transfer of photogenerated carriers, which is crucial for achieving significant improvements in PEC water-splitting performance. Subsequently, Qian et al.[79] combined homojunctions with doping to design a photoanode based on Co2+-doped ZIS loaded onto Mg2+-doped ZIS. The experimental results showed a significant improvement in the PEC performance of the Mg:ZIS/Co:ZIS photoanode compared to pure ZIS. This improvement can be attributed to ion doping, which adjusted the energy level gradient and formed a homojunction, thereby enhancing internal charge separation efficiency. The surface Co2+ participated in the Co2+/Co3+ and Co3+/Co4+ redox reactions, improving the utilization efficiency of surface carriers. The doping of Co2+ played a dual role in enhancing the PEC performance. Compared to simply forming a homojunction, introducing doped metal ions as cocatalysts can further enhance the PEC performance of ZIS. The constructed composite structure of Mg:ZIS/Co:ZIS improved the bulk charge separation and interface charge utilization efficiency after Co2+ doping, significantly boosting the PEC performance. Results showed that the photocurrent generated by the Mg:ZIS/Co:ZIS photoanode at 1.23 V increased significantly to 0.91 mA/cm2, which is 1.71 times, 2.42 times, and 7.49 times that of Co:ZIS, Mg: ZIS, and pure ZIS, respectively [Figure 8A and B]. Notably, the doping of Mg2+ and Co2+ altered the band position and formed a stable junction. Furthermore, the homojunction has the advantage of eliminating lattice mismatches. Under the influence of the built-in electric field, the separation efficiency of carriers was improved [Figure 8C]. Additionally, the Co2+ on the surface of the Co: ZIS layer can act as a catalytic reaction center for water oxidation, promoting the separation of interface charges. The doping of Co2+ endows ZIS with the dual advantages of a homojunction and a cocatalyst.
Figure 8. (A) J-V curves of ZnIn2S4, Mg:ZnIn2S4, Co:ZnIn2S4 and Mg:ZnIn2S4/Co:ZnIn2S4 homojunction photoanodes measured in 0.2 M Na2SO4 solution under AM 1.5G illumination; (B) J-t curves measured at 1.23 V vs. RHE under chopped simulated sunlight illumination with 30 s light on/off cycles; (C) Schematic diagram of Mg:ZnIn2S4/Co:ZnIn2S4 photoanode to enhance the photoelectric performance by enhancing the separation of bulk and interface charges[79]. Copyright 2021, Elsevier. J-V: Photocurrent density-voltage; J-t: transient photocurrent density; RHE: reversible hydrogen electrode.
Thus, heterojunctions and homojunctions represent two key strategies for enhancing the PEC performance of ZIS-based photocatalysts. By exploiting the advantages of band structure modulation, charge separation, and light absorption across a wide range of wavelengths, researchers have made significant progress in overcoming the limitations of single semiconductors, thereby paving the way for more efficient solar energy conversion technologies.
Surface modification
The sluggish surface reaction kinetics remain a significant bottleneck in improving the efficiency of PEC water splitting. Surface modification has been demonstrated as an effective strategy to enhance the PEC performance of semiconductor materials. Common surface modification techniques include cocatalyst loading, surface passivation, and plasma treatments, among others[70,97-101].
Loading active metals or metal compounds (such as Pt, Ni, Co, etc.) onto semiconductor surfaces can significantly enhance PEC performance by serving as cocatalysts. These materials introduce additional active sites or optimize existing ones, thereby accelerating reaction rates and reducing charge recombination. For instance, Zhou et al.[69] improved PEC performance by controlling the morphology of ZIS while simultaneously depositing Co-Pi cocatalysts and Pt NPs on its surface. This design not only reduced surface and interfacial charge recombination but also enhanced light absorption due to the combination of 0D (NPs) and 2D (nanosheets) structures. The unique “edamame-shaped” ZIS configuration was composed of NPs and nanosheets deposited on an indium tin oxide (ITO) substrate, offering multiple reflections and scattering of light, which contributed to better light harvesting. As a result, the Co-Pi/ZIS/Pt device achieved a photocurrent density of 0.91 mA/cm2.
Metal oxides such as Fe2O3, TiO2, Al2O3, and SiO2 have been used as top coatings on transition metal oxide semiconductors to enhance photoelectrocatalytic performance. These coating materials, acting as passivation layers, stabilize surface defects, monitor charge transfer processes at the semiconductor/solution interface, and reduce charge carrier recombination. Mahadik et al.[20] designed metal oxide-coated ZIS/TiO2 (ZT) heterostructure photoanodes and successfully fabricated metal oxide (TiO2, Al2O3, SiO2)-coated ZT heterostructures on fluorine-doped tin oxide (FTO) substrates using a hydrothermal method and an effective dip-coating technique [Figure 9A-C]. Coating the ZT photoanode surface with metal oxides significantly enhanced the PEC performance of the ZT photoanodes [Figure 9D]. Under 0.1V vs. Ag/AgCl, the photocurrent density of the SiO2-treated ZT (SZT) increased to approximately 0.786 mA/cm2, double that of the original ZT [Figure 9E]. The study revealed that the SiO2 surface treatment enhanced interfacial charge transfer while simultaneously reducing carrier recombination losses.
Figure 9. (A) Schematic of the hydrothermal synthesis of TiO2 and ZT on FTO, and fabrication of the metal oxide-coated ZT/FTO heterostructured photoanode; (B) SEM images of the surface view of SZT thin films; (C) SEM images of cross-section of SZT thin films; (D) Schematic of charge transfer mechanism in metal oxide coated ZT photoanode; (E) Evolution of H2 and photocurrent density as a function of time measured at 0.1V vs. Ag/AgCl using SZT as a photoelectrode under 100 mW/cm2 light illumination in a three-electrode configuration, Inset shows a schematic of the PEC hydrogen generation[20]. Copyright 2016, Elsevier. ZT: ZIS/TiO2; ZIS:
In summary, surface modification plays a crucial role in enhancing the PEC performance of semiconductor photocatalysts. By carefully selecting and combining cocatalysts, passivation layers, and advanced surface treatments, it is possible to significantly improve charge separation, increase light absorption, and ultimately enhance the overall efficiency of PEC water splitting.
CONCLUSION AND FUTURE PROSPECTS
In light of the escalating global energy crisis and worsening environmental degradation, the development of sustainable and environmentally friendly materials has become a critical priority. PEC water splitting, which enables the production of hydrogen-a clean and renewable energy source-has emerged as a particularly promising solution. Among the diverse array of n-type semiconductors, ZIS has garnered considerable attention due to its optimal bandgap, which facilitates extensive visible light absorption and efficient solar energy conversion. Its high stability, low toxicity, and distinctive bilayered structure, which shortens the diffusion distance for photogenerated carriers, further enhance its potential by reducing charge recombination and boosting overall water-splitting efficiency. Additionally, the tunable electronic structure of ZIS allows for performance optimization through strategic doping and surface modifications.
Despite these advantages, ZIS-based photoanodes face several challenges that must be addressed to unlock their full potential. One of the primary issues is the material’s light absorption efficiency, which may be insufficient for certain applications. This limitation could stem from an imperfect bandgap width that fails to capture specific wavelengths effectively or from an inherently limited light absorption capacity. Furthermore, the effective separation and transport of photogenerated carriers are crucial for PEC performance. However, ZIS-based materials may exhibit suboptimal carrier separation efficiency or encounter obstacles in carrier transport pathways, leading to diminished photoelectric conversion efficiency. Long-term stability is another concern, as ZIS-based photoanodes may be prone to photocorrosion or chemical instability, which could compromise their durability and lifespan. Additionally, the presence of unintended doping and defects in ZIS materials might negatively affect their electronic structure and overall PEC performance, posing a significant technical challenge in the precise control of these factors.
An important aspect that has been largely overlooked is the surface reconstruction that occurs during PEC reactions[102-104]. During PEC processes, the material’s surface can undergo structural changes, which might influence its catalytic activity and stability. This surface reconstruction has yet to receive sufficient attention in the existing literature, but it represents a critical area for future research, as it can significantly affect the performance of ZIS-based photoanodes. The dynamic nature of the surface during PEC reactions may alter charge transfer rates, carrier separation efficiency, and overall stability, thus influencing the long-term performance of these materials in practical applications. Understanding and controlling these surface dynamics will be essential for optimizing the efficiency and stability of ZIS-based photoanodes in PEC systems.
Looking ahead, the future of ZIS-based photoanodes is filled with promise. With continued advancements in material design and optimization, there is significant potential to enhance the solar-to-hydrogen conversion efficiency of these materials. Innovations in fabrication techniques and scalable production processes could lead to reduced costs, making ZIS-based photoanodes more commercially viable. Moreover, improvements in material and interface stability could significantly extend the lifespan and reliability of these photoanodes in practical applications. Future iterations of ZIS-based photoanodes may also incorporate additional functionalities tailored to specific environmental conditions, further enhancing their performance.
To achieve these goals, future research should prioritize the optimization of the electronic structure and optical properties of ZIS. This can be accomplished through advanced doping techniques, surface modifications, and innovative structural designs aimed at maximizing light absorption and improving carrier separation efficiency. Additionally, understanding the effects of surface reconstruction during PEC reactions and developing strategies to mitigate any negative impacts will be essential for enhancing the material’s performance. Precise control over defects and doping will be crucial to optimizing the generation, transport, and collection of carriers, ultimately enhancing photoelectric conversion efficiency. Additionally, integrating ZIS photoanodes with other high-efficiency PEC components could result in a more optimized and effective overall system, maximizing energy conversion efficiency. It is also imperative to explore the degradation mechanisms of ZIS-based materials within PEC environments. Developing robust anti-corrosion and chemical stability strategies will be essential to extending the operational lifespan of these materials.
In conclusion, ZIS-based photoanodes represent a significant advancement in the pursuit of efficient and sustainable PEC water-splitting systems. While considerable progress has been made, overcoming the remaining challenges and further refining these materials are essential steps toward practical implementation. Through ongoing research and innovation, ZIS-based photoanodes have the potential to play a pivotal role in the transition to a sustainable energy future, contributing meaningfully to global efforts in combating climate change and achieving energy security.
DECLARATIONS
Authors’ contributions
Reviewed literature extensively, wrote and modified articles, created figures, and set the layout: Wang, S.
Polished the article and designed the figures: Cao, S.; Wang, L.
Revised the article, provided research direction, and offered funding support: Hou, H.; Yang, W.
Availability of data and materials
Not applicable.
Financial support and sponsorship
This work was supported by the National Natural Science Foundation of China (NSFC, Grant Nos. 52272085, 52372063 and 51972178), Natural Science Foundation of Zhejiang Province (Grant No. LY23E020002), China Postdoctoral Science Foundation (Grant No. 2024M753526), Ningbo Youth Science and Technology Innovation Leading Talents Project (Grant No. 2023QL031), and Postdoctoral Fellowship Program of CPSF under Grant Number GZC20233006.
Conflicts of interest
All authors declared that there are no conflicts of interest.
Ethical approval and consent to participate
Not applicable.
Consent for publication
Not applicable.
Copyright
© The Author(s) 2025.
REFERENCES
1. Roda, D.; Trzciński, K.; Łapiński, M.; et al. The new method of ZnIn2S4 synthesis on the titania nanotubes substrate with enhanced stability and photoelectrochemical performance. Sci. Rep. 2023, 13, 21263.
2. Zhang, G.; Wu, H.; Chen, D.; et al. A mini-review on ZnIn2S4-based photocatalysts for energy and environmental application. Green. Energy. Environ. 2022, 7, 176-204.
3. Song, Y.; Zhang, J.; Dong, X.; Li, H. A review and recent developments in full-spectrum photocatalysis using ZnIn2S4-based photocatalysts. Energy. Tech. 2021, 9, 2100033.
4. Feng, C.; Wu, Z. P.; Huang, K. W.; Ye, J.; Zhang, H. Surface modification of 2D photocatalysts for solar energy conversion. Adv. Mater. 2022, 34, 2200180.
5. Yang, L.; Li, F.; Xiang, Q. Advances and challenges in the modification of photoelectrode materials for photoelectrocatalytic water splitting. Mater. Horiz. 2024, 11, 1638-57.
6. Gong, Y.; Liu, J.; Shao, B.; Zhong, D.; Lu, T. Stable metal-organic frameworks for PEC water splitting. FlatChem 2021, 27, 100240.
7. Xu, X. T.; Pan, L.; Zhang, X.; Wang, L.; Zou, J. J. Rational design and construction of cocatalysts for semiconductor-based photo-electrochemical oxygen evolution: a comprehensive review. Adv. Sci. 2019, 6, 1801505.
8. Sun, J.; Wang, J.; Zhang, X.; et al. Using VO2 as a hole storage layer to improve PEC water splitting performance of BiVO4 photoanode. Int. J. Hydrogen. Energy. 2024, 69, 95-102.
9. Dong, G.; Yan, L.; Bi, Y. Advanced oxygen evolution reaction catalysts for solar-driven photoelectrochemical water splitting. J. Mater. Chem. A. 2023, 11, 3888-903.
10. Song, Y.; Ren, Y.; Cheng, H.; et al. Metal-organic framework glass catalysts from melting glass-forming cobalt-based zeolitic imidazolate framework for boosting photoelectrochemical water oxidation. Angew. Chem. Int. Ed. 2023, 62, e202306420.
11. Singh, I.; Bhullar, V.; Mahajan, A. Interfacial engineering of a TiO2 photoanode via graphene nanoribbons for efficient quantum-dot-sensitized solar cells and photoelectrochemical water splitting. Energy. Fuels. 2023, 37, 15054-66.
12. Feng, Y.; Guan, L.; Li, J.; et al. Fabrication of WO3 photoanode on crystalline Si solar cell for water splitting. J. Mater. Sci. Mater. Electron. 2020, 31, 14137-44.
13. Krysiak, O. A.; Junqueira, J. R.; Conzuelo, F.; et al. Importance of catalyst-photoabsorber interface design configuration on the performance of Mo-doped BiVO4 water splitting photoanodes. J. Solid. State. Electrochem. 2021, 25, 173-85.
14. Choi, M. J.; Kim, T. L.; Choi, K. S.; et al. Controlled band offsets in ultrathin hematite for enhancing the photoelectrochemical water splitting performance of heterostructured photoanodes. ACS. Appl. Mater. Interfaces. 2022, 14, 7788-95.
15. Zheng, X.; Song, Y.; Liu, Y.; et al. ZnIn2S4-based photocatalysts for photocatalytic hydrogen evolution via water splitting. Coord. Chem. Rev. 2023, 475, 214898.
16. Yan, Y.; Chen, Z.; Cheng, X.; Shi, W. Research progress of ZnIn2S4-based catalysts for photocatalytic overall water splitting. Catalysts 2023, 13, 967.
17. Long, C.; Dong, X.; Huang, J. Latest progress on photocatalytic H2 production by water splitting and H2 production coupled with selective oxidation of organics over ZnIn2S4-based photocatalysts. Energy. Fuels. 2023, 37, 136-58.
18. Ren, Y.; Foo, J. J.; Zeng, D.; Ong, W. ZnIn2S4-based nanostructures in artificial photosynthesis: insights into photocatalytic reduction toward sustainable energy production. Small. Struct. 2022, 3, 2200017.
19. Khosya, M.; Kumar, D.; Faraz, M.; Khare, N. Enhanced photoelectrochemical water splitting and photocatalytic degradation performance of visible light active ZnIn2S4/PANI nanocomposite. Int. J. Hydrogen. Energy. 2023, 48, 2518-31.
20. Mahadik, M. A.; Shinde, P. S.; Cho, M.; Jang, J. S. Metal oxide top layer as an interfacial promoter on a ZnIn2S4/TiO2 heterostructure photoanode for enhanced photoelectrochemical performance. Appl. Catal. B. Environ. 2016, 184, 337-46.
21. Pan, F.; Long, L.; Li, Z.; et al. Substitutional Cd dopant as photohole transfer mediator boosting photoelectrochemical solar energy conversion of 2D Cd-ZnIn2S4 photoanode. Small 2024, 20, 2304846.
22. Lin, Y.; Fang, W.; Xv, R.; Fu, L. TiO2 nanoparticles modified with ZnIn2S4 nanosheets and Co-Pi groups: type II heterojunction and cocatalysts coexisted photoanode for efficient photoelectrochemical water splitting. Int. J. Hydrogen. Energy. 2022, 47, 33361-73.
23. Chen, J.; Li, K.; Cai, X.; Zhao, Y.; Gu, X.; Mao, L. Sulfur vacancy-rich ZnIn2S4 nanosheet arrays for visible-light-driven water splitting. Mater. Sci. Semicond. Process. 2022, 143, 106547.
24. Song, Y.; Zheng, X.; Yang, Y.; et al. Heterojunction engineering of multinary metal sulfide-based photocatalysts for efficient photocatalytic hydrogen evolution. Adv. Mater. 2024, 36, 2305835.
25. Bao, Z.; Jiang, Y.; Zhang, Z.; et al. Visible-light-responsive S-vacancy ZnIn2S4/N-doped TiO2 nanoarray heterojunctions for high-performance photoelectrochemical water splitting. J. Mater. Chem. A. 2024, 12, 15902-13.
26. Wang, J.; Sun, S.; Zhou, R.; et al. A review: synthesis, modification and photocatalytic applications of ZnIn2S4. J. Mater. Sci. Technol. 2021, 78, 1-19.
27. Lee, J.; Kim, H.; Lee, T.; Jang, W.; Lee, K. H.; Soon, A. Revisiting polytypism in hexagonal ternary sulfide ZnIn2S4 for photocatalytic hydrogen production within the Z-scheme. Chem. Mater. 2019, 31, 9148-55.
28. Yang, W.; Liu, B.; Fang, T.; et al. Layered crystalline ZnIn2S4 nanosheets: CVD synthesis and photo-electrochemical properties. Nanoscale 2016, 8, 18197-203.
29. Wang, J.; Chen, Y.; Zhou, W.; et al. Cubic quantum dot/hexagonal microsphere ZnIn2S4 heterophase junctions for exceptional visible-light-driven photocatalytic H2 evolution. J. Mater. Chem. A. 2017, 5, 8451-60.
30. Luan, Q.; Xue, X.; Li, R.; et al. Boosting photocatalytic hydrogen evolution: orbital redistribution of ultrathin ZnIn2S4 nanosheets via atomic defects. Appl. Catal. B. Environ. 2022, 305, 121007.
31. Chong, W.; Ng, B.; Kong, X. Y.; Tan, L.; Putri, L. K.; Chai, S. Non-metal doping induced dual p-n charge properties in a single ZnIn2S4 crystal structure provoking charge transfer behaviors and boosting photocatalytic hydrogen generation. Appl. Catal. B. Environ. 2023, 325, 122372.
32. Zhang, W.; Zhao, S.; Xing, Y.; et al. Sandwich-like P-doped h-BN/ZnIn2S4 nanocomposite with direct Z-scheme heterojunction for efficient photocatalytic H2 and H2O2 evolution. Chem. Eng. J. 2022, 442, 136151.
33. Dai, M.; He, Z.; Zhang, P.; Li, X.; Wang, S. ZnWO4-ZnIn2S4 S-scheme heterojunction for enhanced photocatalytic H2 evolution. J. Materi. Sci. Technol. 2022, 122, 231-42.
34. Hao, C.; Tang, Y.; Shi, W.; Chen, F.; Guo, F. Facile solvothermal synthesis of a Z-scheme 0D/3D CeO2/ZnIn2S4 heterojunction with enhanced photocatalytic performance under visible light irradiation. Chem. Eng. J. 2021, 409, 128168.
35. Alshgari, R. A.; Kumar, O. P.; Shah, J. H.; Mohammad, S.; Abid, A. G. Nanosphere-like ZnIn2S4 intercalated g-C3N4 for improved green oxygen production. J. Korean. Ceram. Soc. 2024, 61, 1013-26.
36. Li, H.; Chen, Z.; Zhao, L.; Yang, G. Synthesis of TiO2@ZnIn2S4 hollow nanospheres with enhanced photocatalytic hydrogen evolution. Rare. Met. 2019, 38, 420-7.
37. Ye, L.; Li, Z. ZnIn2S4: a photocatalyst for the selective aerobic oxidation of amines to imines under visible light. ChemCatChem 2014, 6, 2540-3.
38. Gou, X.; Cheng, F.; Shi, Y.; et al. Shape-controlled synthesis of ternary chalcogenide ZnIn2S4 and CuIn(S,Se)2 nano-/microstructures via facile solution route. J. Am. Chem. Soc. 2006, 128, 7222-9.
39. Geng, H.; Ying, P.; Li, K.; Zhao, Y.; Gu, X. Epitaxial In2S3/ZnIn2S4 heterojunction nanosheet arrays on FTO substrates for photoelectrochemical water splitting. Appl. Surf. Sci. 2021, 563, 150289.
40. Kale, B. B.; Bhirud, A. P.; Baeg, J. O.; Kulkarni, M. V. Template free architecture of hierarchical nanostructured ZnIn2S4 rose-like flowers for solar hydrogen production. J. Nanosci. Nanotechnol. 2017, 17, 1447-454.
41. Zhou, M. J.; Cui, P. Synthesis and photocatalytic properties of flower-like ZnIn2S4 microspheres by a solvothermal method. Adv. Mater. Res. 2013, 881-3, 1101-4.
42. Huang, Y.; He, J.; Xu, W.; et al. Converting Undesirable defects into activity sites enhances the photoelectrochemical performance of the ZnIn2S4 photoanode. Adv. Energy. Mater. 2024, 14, 2304376.
43. Cheng, K.; Liang, C. Preparation of Zn-In-S film electrodes using chemical bath deposition for photoelectrochemical applications. Sol. Energy. Mater. Sol. Cells. 2010, 94, 1137-45.
44. Sun, Y.; Xue, C.; Chen, L.; et al. Enhancement of interfacial charge transportation through construction of 2D-2D p-n heterojunctions in hierarchical 3D CNFs/MoS2/ZnIn2S 4 composites to enable high-efficiency photocatalytic hydrogen evolution. Solar. RRL. 2021, 5, 2000722.
45. Gao, Y.; Ji, X.; Zhang, D.; Liu, Z.; Lu, J. Microwave-assisted fabrication of CQDs/ZnIn2S4 nanocomposites for synergistic photocatalytic removal of Cr(VI) and rhodamine B. Inorg. Nano-Metal. Chem. 2021, 51, 451-7.
46. Chen, Z.; Li, D.; Xiao, G.; He, Y.; Xu, Y. Microwave-assisted hydrothermal synthesis of marigold-like ZnIn2S4 microspheres and their visible light photocatalytic activity. J. Solid. State. Chem. 2012, 186, 247-54.
47. Mahadik, M. A.; Patil, R. P.; Chae, W.; Hwi, L. H.; Cho, M.; Suk, J. J. Microwave-assisted rapid synthesis of Cu2S:ZnIn2S4 marigold-like nanoflower heterojunctions and enhanced visible light photocatalytic hydrogen production via Pt sensitization. J. Ind. Eng. Chem. 2022, 108, 203-14.
48. Chang, Y. C.; Bi, J. N.; Pan, K. Y.; Chiao, Y. C. Microwave-assisted synthesis of SnO2@ZnIn2S4 composites for highly efficient photocatalytic hydrogen evolution. Materials 2024, 17, 2367.
49. Mishra, M.; Huang, Y. C.; Wang, P. H.; Liu, S. P.; Lee, T. R.; Lee, T. C. Tuning the crystallinity and coverage of SiO2-ZnIn2S4 core-shell nanoparticles for efficient hydrogen generation. ACS. Appl. Mater. Interfaces. 2021, 13, 4043-50.
50. Sun, M.; Zhao, X.; Zeng, Q.; et al. Facile synthesis of hierarchical ZnIn2S4/CdIn2S4 microspheres with enhanced visible light driven photocatalytic activity. Appl. Surf. Sci. 2017, 407, 328-36.
51. Bedala, K. K.; Gonugunta, P.; Soleimani, M.; et al. Facile synthesis of ZnIn2S4/Cu2O hierarchical heterostructures for enhanced selectivity and sensitivity of NH3 gas at room temperature. Appl. Surf. Sci. 2023, 640, 158315.
52. Xu, Z.; Shi, W.; Shi, Y.; et al. Carbon dots as solid-state electron mediator and electron acceptor in S-scheme heterojunction for boosted photocatalytic hydrogen evolution. Appl. Surf. Sci. 2022, 595, 153482.
53. Chen, W.; Yan, R.; Zhu, J.; Huang, G.; Chen, Z. Highly efficient visible-light-driven photocatalytic hydrogen evolution by all-solid-state Z-scheme CdS/QDs/ZnIn2S4 architectures with MoS2 quantum dots as solid-state electron mediator. Appl. Surf. Sci. 2020, 504, 144406.
54. Yang, R.; Mei, L.; Fan, Y.; et al. ZnIn2S4-based photocatalysts for energy and environmental applications. Small. Methods. 2021, 5, 2100887.
55. Fang, W.; Liu, J.; Zhang, Y.; et al. Alkaline induced indium gradient distribution in ZnmIn2S3+m/In(OH)3 heterojunction for improved photocatalytic H2 generation. Appl. Surf. Sci. 2020, 530, 147241.
56. Pan, Y.; Yuan, X.; Jiang, L.; et al. Recent advances in synthesis, modification and photocatalytic applications of micro/nano-structured zinc indium sulfide. Chem. Eng. J. 2018, 354, 407-31.
57. Wang, S.; Wang, Y.; Zhang, S. L.; Zang, S. Q.; Lou, X. W. D. Supporting ultrathin ZnIn2S4 nanosheets on Co/N-doped graphitic carbon nanocages for efficient photocatalytic H2 generation. Adv. Mater. 2019, 31, 1903404.
58. Huang, L.; Han, B.; Huang, X.; et al. Ultrathin 2D/2D ZnIn2S4/MoS2 hybrids for boosted photocatalytic hydrogen evolution under visible light. J. Alloys. Compd. 2019, 798, 553-9.
59. Chen, Y.; Huang, R.; Chen, D.; et al. Exploring the different photocatalytic performance for dye degradations over hexagonal ZnIn2S4 microspheres and cubic ZnIn2S4 nanoparticles. ACS. Appl. Mater. Interfaces. 2012, 4, 2273-9.
60. Uddin, A.; Muhmood, T.; Guo, Z.; Gu, J.; Chen, H.; Jiang, F. Hydrothermal synthesis of 3D/2D heterojunctions of ZnIn2S4/oxygen doped g-C3N4 nanosheet for visible light driven photocatalysis of 2,4-dichlorophenoxyacetic acid degradation. J. Alloys. Compd. 2020, 845, 156206.
61. Xu, L.; Deng, X.; Li, Z. Photocatalytic splitting of thiols to produce disulfides and hydrogen over PtS/ZnIn2S4 nanocomposites under visible light. Appl. Catal. B. Environ. 2018, 234, 50-5.
62. Li, M.; Ke, S.; Yang, X.; Shen, L.; Yang, M. Q. S-scheme homojunction of 0D cubic/2D hexagonal ZnIn2S4 for efficient photocatalytic reduction of nitroarenes. J. Colloid. Interface. Sci. 2024, 674, 547-59.
63. Wang, S.; Guan, B. Y.; Lou, X. W. D. Construction of ZnIn2S4-In2O3 hierarchical tubular heterostructures for efficient CO2 photoreduction. J. Am. Chem. Soc. 2018, 140, 5037-40.
64. Zuo, G.; Wang, Y.; Teo, W. L.; Xian, Q.; Zhao, Y. Direct Z-scheme TiO2-ZnIn2S4 nanoflowers for cocatalyst-free photocatalytic water splitting. Appl. Catal. B. Environ. 2021, 291, 120126.
65. Chen, Y.; Wang, M.; He, B.; Zou, R.; Wu, Q. Facile design and fabrication of RP/ZnIn2S4 composite photocatalysts with efficient removal of antibiotics under visible-light irradiation. J. Alloys. Compd. 2023, 968, 171972.
66. Tu, B.; Che, R.; Wang, F.; Li, Y.; Li, J.; Qiu, J. New insights into the enhancement of TiO2/ZnIn2S4 heterojunction via cerium doping. Appl. Surf. Sci. 2023, 629, 157451.
67. Zhou, F.; Zhang, Y.; Wu, J.; et al. Utilizing Er-doped ZnIn2S4 for efficient photocatalytic CO2 conversion. Appl. Catal. B. Environ. 2024, 341, 123347.
68. Khosya, M.; Faraz, M.; Khare, N. Enhanced photoelectrochemical water splitting in ternary layered chalcogenide ZnIn2S4 coupled with MWCNT. Nano. Trends. 2023, 4, 100018.
69. Zhou, M.; Liu, Z.; Song, Q.; Li, X.; Chen, B.; Liu, Z. Hybrid 0D/2D edamame shaped ZnIn2S4 photoanode modified by Co-Pi and Pt for charge management towards efficient photoelectrochemical water splitting. Appl. Catal. B. Environ. 2019, 244, 188-96.
70. Zhang, S.; Du, P.; Xiao, H.; et al. Fast interfacial carrier dynamics modulated by bidirectional charge transport channels in ZnIn2S4-based composite photoanodes probed by scanning photoelectrochemical microscopy. Angew. Chem. Int. Ed. 2024, 63, e202315763.
71. Li, J.; Wang, C.; Guo, Z.; Ruan, M. Piezoelectric effect promoted photoelectrochemical water splitting ability of ZnIn2S4 photoanode with highly exposed active (110) facets. ChemCatChem 2024, 16, e202301318.
72. Li, S.; Meng, L.; Tian, W.; Li, L. Engineering interfacial band bending over ZnIn2S4/SnS2 by interface chemical bond for efficient solar-driven photoelectrochemical water splitting. Adv. Energy. Mater. 2022, 12, 2200629.
73. Lv, G.; Long, L.; Wu, X.; et al. Realizing highly efficient photoelectrochemical performance for vertically aligned 2D ZnIn2S4 array photoanode via controlled facet and phase modulation. Appl. Surf. Sci. 2023, 609, 155335.
74. Khosya, M.; Kumar, D.; Faraz, M.; Khare, N. Visible light active ZnIn2S4/g-C3N4 heterostructure nanocomposite photoelectrode for efficient photoelectrochemical water splitting activity. Adv. Powder. Technol. 2023, 34, 104051.
75. Hao, Z.; Wang, R.; Zhang, L.; et al. Sufficient energy band utilization profited from spatially discrete heterogeneous interfaces to induce efficient photoelectrochemical water splitting for ZnIn2S4 photoanode. Surf. Interfaces. 2024, 51, 104667.
76. Wang, L.; Zheng, M.; Lai, L.; et al. Immobilization of prussian blue nanoparticles onto Au-modified ZnIn2S4 photoanode for efficient photoelectrochemical water splitting. Eur. J. Inorg. Chem. 2024, 27, e202400007.
77. Fan, B.; Chen, Z.; Liu, Q.; Zhang, Z.; Fang, X. One-pot hydrothermal synthesis of Ni-doped ZnIn2S4 nanostructured film photoelectrodes with enhanced photoelectrochemical performance. Appl. Surf. Sci. 2016, 370, 252-9.
78. Qian, H.; Liu, Z.; Guo, Z.; Ruan, M.; Ma, J. Hexagonal phase/cubic phase homogeneous ZnIn2S4 n-n junction photoanode for efficient photoelectrochemical water splitting. J. Alloys. Compd. 2020, 830, 154639.
79. Qian, H.; Liu, Z.; Ya, J.; Xin, Y.; Ma, J.; Wu, X. Construction homojunction and co-catalyst in ZnIn2S4 photoelectrode by Co ion doping for efficient photoelectrochemical water splitting. J. Alloys. Compd. 2021, 867, 159028.
80. Wu, Y.; Yao, S.; Lv, G.; et al. Construction of p-n junctions in single-unit-cell ZnIn2S4 nanosheet arrays toward promoted photoelectrochemical performance. J. Catal. 2021, 401, 262-70.
81. Xu, W.; Gao, W.; Meng, L.; Tian, W.; Li, L. Incorporation of sulfate anions and sulfur vacancies in ZnIn2S4 photoanode for enhanced photoelectrochemical water splitting. Adv. Energy. Mater. 2021, 11, 2101181.
82. Wu, K.; Yao, C.; Wu, P.; et al. Highly efficient hydrogen production performance of g-C3N4 quantum dot-sensitized WO3/Ni-ZnIn2S4 nanosheets. Appl. Phys. A. 2022, 128, 6055.
83. Fan, H.; Jin, Y.; Liu, K.; Liu, W. One-step MOF-templated strategy to fabrication of Ce-doped ZnIn2S4 tetrakaidecahedron hollow nanocages as an efficient photocatalyst for hydrogen evolution. Adv. Sci. 2022, 9, 2104579.
84. Zhou, D.; Xue, X.; Wang, X.; et al. Ni, In co-doped ZnIn2S4 for efficient hydrogen evolution: modulating charge flow and balancing H adsorption/desorption. Appl. Catal. B. Environ. 2022, 310, 121337.
85. Dong, W.; Zhou, S.; Ma, Y.; et al. N-doped C-coated MoO2/ZnIn2S4 heterojunction for efficient photocatalytic hydrogen production. Rare. Met. 2023, 42, 1195-204.
86. Shi, X.; Dai, C.; Wang, X.; et al. Facile construction TiO2/ZnIn2S4/Zn0.4Ca0.6In2S4 ternary hetero-structure photo-anode with enhanced photo-electrochemical water-splitting performance. Surf. Interfaces. 2021, 26, 101323.
87. Hu, Z.; Wang, R.; Han, C.; Chen, R. Plasmon-induced hole-depletion layer on p-n heterojunction for highly efficient photoelectrochemical water splitting. J. Colloid. Interface. Sci. 2022, 628, 946-54.
88. Zhao, Y.; Linghu, X.; Shu, Y.; et al. Classification and catalytic mechanisms of heterojunction photocatalysts and the application of titanium dioxide (TiO2)-based heterojunctions in environmental remediation. J. Environ. Chem. Eng. 2022, 10, 108077.
89. Yue, Y.; Zou, J. Oxygen vacancy-suppression strengthened the internal electric field in ZnIn2S4/BiVO4 S-scheme heterojunction to boost photocatalytic removal of aqueous pollutants. J. Environ. Chem. Eng. 2024, 12, 113473.
90. Wang, S.; Zhang, D.; Pu, X.; Zhang, L.; Zhang, D.; Jiang, J. Photothermal-enhanced S-scheme heterojunction of hollow core-shell FeNi2S4@ZnIn2S4 toward photocatalytic hydrogen evolution. Small 2024, 20, 2311504.
91. Liu, D.; Jiang, L.; Chen, D.; et al. Twin S-scheme g-C3N4/CuFe2O4/ZnIn2S4 heterojunction with a self-supporting three-phase system for photocatalytic CO2 reduction: mechanism insight and DFT calculations. ACS. Catal. 2024, 14, 5326-43.
92. Wang, H.; Ning, Y.; Tang, Q.; et al. Ultrathin 2D/2D ZnIn2S4/La2Ti2O7 nanosheets with a Z-scheme heterojunction for enhanced photocatalytic hydrogen evolution. Dalton. Trans. 2024, 53, 13491-502.
93. Xu, W.; Tian, W.; Meng, L.; Cao, F.; Li, L. Interfacial chemical bond-modulated Z-scheme charge transfer for efficient photoelectrochemical water splitting. Adv. Energy. Mater. 2021, 11, 2003500.
94. Li, J.; Wu, C.; Li, J.; Dong, B.; Zhao, L.; Wang, S. 1D/2D TiO2/ZnIn2S4 S-scheme heterojunction photocatalyst for efficient hydrogen evolution. Chin. J. Catal. 2022, 43, 339-49.
95. Yue, Y.; Zou, J. Boosting interfacial charge separation for ZnIn2S4 homojunction by lattice matching effect to enhance photocatalytic performance. J. Alloys. Compd. 2023, 966, 171659.
96. Wang, H.; Li, M.; You, Z.; Chen, Y.; Liu, Y. An innovative Zn3In2S6/ZnIn2S4 homojunction photocatalyst with enhanced interfacial charge transfer for the highly efficient degradation of tetracycline under visible radiation. J. Environ. Manage. 2024, 365, 121605.
97. Jiang, Z.; Li, K.; Cai, X.; et al. Enhanced performance of FeOOH/ZnIn2S4/Au nanosheet arrays for visible light water splitting. J. Mater. Sci. Mater. Electron. 2022, 33, 6070-81.
98. Li, C.; Liu, X.; Ding, G.; et al. Interior and surface synergistic modifications modulate the SnNb2O6/Ni-doped ZnIn2S4 S-scheme heterojunction for efficient photocatalytic H2 evolution. Inorg. Chem. 2022, 61, 4681-9.
99. Wang, G.; Sun, X.; Xia, C.; Li, H.; Dong, B.; Cao, L. Sulfur poisoning-resistant TiO2/Cu-doped ZnIn2S4 photoanode for achieving efficient sulfur oxidation. Colloids. Surf. A. Physicochem. Eng. Aspects. 2024, 689, 133656.
100. Peng, Y.; Guo, X.; Xu, S.; et al. Surface modulation of MoS2/O-ZnIn2S4 to boost photocatalytic H2 evolution. J. Energy. Chem. 2022, 75, 276-84.
101. Zhao, H.; Yao, Y.; Cai, M.; et al. Synergistic selenium doping and colloidal quantum dots decoration over ZnIn2S4 enabling high-efficiency photoelectrochemical hydrogen peroxide production. Chem. Eng. J. 2024, 491, 151925.
102. Jeong, Y. J.; Tan, R.; Nam, S.; et al. Rapid surface reconstruction of In2S3 photoanode via flame treatment for enhanced photoelectrochemical performance. Adv. Mater. 2024, 2403164.
103. Gao, L.; Cui, X.; Sewell, C. D.; Li, J.; Lin, Z. Recent advances in activating surface reconstruction for the high-efficiency oxygen evolution reaction. Chem. Soc. Rev. 2021, 50, 8428-69.
Cite This Article

How to Cite
Download Citation
Export Citation File:
Type of Import
Tips on Downloading Citation
Citation Manager File Format
Type of Import
Direct Import: When the Direct Import option is selected (the default state), a dialogue box will give you the option to Save or Open the downloaded citation data. Choosing Open will either launch your citation manager or give you a choice of applications with which to use the metadata. The Save option saves the file locally for later use.
Indirect Import: When the Indirect Import option is selected, the metadata is displayed and may be copied and pasted as needed.
About This Article
Copyright
Data & Comments
Data
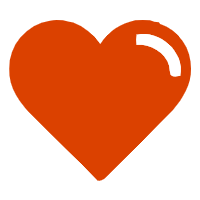
Comments
Comments must be written in English. Spam, offensive content, impersonation, and private information will not be permitted. If any comment is reported and identified as inappropriate content by OAE staff, the comment will be removed without notice. If you have any queries or need any help, please contact us at [email protected].