Recent processing of interaction mechanisms of single metallic atom/clusters in energy electrocatalysis
Abstract
Understanding the interactions between single metallic atom/clusters (SMACs) has been taken to an unprecedented level, due to the delicate conditions required to produce exotic phenomena in electrode materials, such as thermocatalysis, electrocatalysis, and energy storage devices. Recently, state-of-the-art synthesis methods, such as one-step pyrolysis and multistep pyrolysis, have been developed for SMACs. Herein the interactions between SMACs such as synergetic, charge redistribution effects, and mutual assistance effects, are studied. SMACs have the advantage of maximum utilization of atoms and scattered active sites compared to single metal atoms, and they also have flexible and tunable atom clusters. SMACs have been widely developed and have shown excellent catalytic performance in electrocatalysis. Herein, the self-interaction between SMACs and their catalytic mechanisms are systematically described. The challenges in current synthesis strategies, catalytic mechanisms, and industrial applications of SMACs are analyzed, and a possible synthesis method for SMACs is proposed.
Keywords
INTRODUCTION
Driven by the dual-carbon strategy, electrochemical energy is the source of power for promoting and realizing modernization as well as for the steady growth of the economy in China[1-3]. To mitigate environmental problems and the energy crisis, electrochemical catalytic reactions, such as hydrogen evolution reaction (HER)[4-6], oxygen evolution reaction (OER)[7], oxygen reduction reaction (ORR)[8], carbon dioxide reduction reaction (CO2RR)[9-11], nitrogen reduction reaction (NRR)[12], and nitrate reduction reaction (NITRR)[13] have attracted great attention. Understanding the catalyst synthesis and reaction mechanism is vital for the development of electrochemistry. Catalytic reaction mechanisms in electrochemical energy engineering have recently attracted research attention[14]. Inhomogeneous catalysis has contributed to catalyst diversity and the development of several industries that require multiple complex chemical catalytic processes, such as the energy and environmental industries[15]. Multiphase catalysts also play a key role in environmental pollution problem-solving, green chemistry, and energy storage processes[16]. Despite tremendous developments in the research of catalysts, many challenges still need to be addressed. Insufficient catalytic activity limits the efficiency of industrial production[17]. Catalytic selectivity is also an important indicator for reducing molecular separation costs[18]. Poor catalyst lifetime, i.e., stability, leads to environmental pollution and significantly increases the cost, which can directly hinder its large-scale application[19-21].
In heterogeneous catalysis, the interaction between gas-phase reactants and solid-phase catalysts occurs at the active sites on the solid surface[22-24]. Increasing the number of active sites on the catalyst surface is a direct way to improve catalytic efficiency[25]. Single metallic atom (SMA) site catalysts, characterized by maximum atom utilization and isolated active sites, have recently been extensively researched. It should be noted that the single metallic atom sites discussed in this review do not only refer to the atoms that coordinate with heteroatoms (such as carbon, nitrogen, sulfur, and oxygen), but also include the sites that form mono-atomic alloy catalysts with other metallic elements. The ratio of surface metal atoms to total metal atoms of these catalysts can theoretically be increased to 100%, corresponding to that of fully exposed cluster catalysts[26-28]. Over the past few decades, various methods have been proposed to modify SMA catalyst structures at the atomic level to achieve high metal site density, suitable d-band centers, and optimal binding strength to reactant intermediates[29]. Although catalysts with fully exposed active sites have excellent catalytic activity, their synthesis and stability cannot be controlled during catalysis. Metal atoms agglomerate due to high-temperature pyrolysis during SMA synthesis[30]. Thus, the synthesis of SMA requires precise control of the pyrolysis temperature after the metal atoms are agglomerated, which leads to the atomic recovery rate decreasing dramatically. In addition, SMA catalysts are extremely sensitive to the environment and detach from metal sites by acidic electrolytes, which can degrade their catalytic activity. In fact, the pH of the catalytic environment can greatly affect the catalytic activity. ORR, for example, is limited by proton-coupled electron transfer at pH below 10.5. At higher pH values, the reaction occurs in the outer Helmholtz plane (OHP), which is conducive to the production of H2O2[31,32].
Unlike SMA catalysts, interactions in single metallic atom/clusters (SMACs) are crucial for enhancing the catalytic activity of materials[33,34]. Here, we define the block interacting with the single atomic site as cluster. In the traditional definition of cluster, the size often determines the catalytic performance to a large extent. In order to cover a wide range of interaction mechanisms, clusters mentioned in this paper are not limited to subnanometer or nanometer sizes. Thus, the electronic structure and chemical state of catalytic sites on the catalyst surface can be modified using SMACs[35]. To ensure low active-site density, metal sub-nano clusters are loaded, which change the electronic states of the active sites and the electronic structure of the surface[36,37]. However, this interaction mechanism has not been discussed in detail and is referred to as the synergistic effect. This synergistic effect exists between active sites and intermediates, which optimize their electronic structure and binding behavior, respectively [Scheme 1].
The synthesis, structural modification, performance implications, and specific applications of cluster or SMA catalysts have been reported previously reported[38,39]. Herein, the advantages and disadvantages, fabrication methods, interactions, and catalytic mechanisms of SMACs are described. We classify the catalytic mechanisms of SMACs into synergistic effects, charge redistribution effects, and mutual assistance. The charge redistribution effect includes charge transfer, strong electronic coupling effect, and spin state adjustment. SMACs interactions are studied to understand their catalytic effects. Finally, the challenges and prospects of SMACs in industrial applications are discussed. The results of this study will pave the way for the research of SMACs as energy electrocatalysts.
SYNTHETIC STRATEGY OF SINGLE METALLIC ATOM/CLUSTERS ELECTROCATALYST
SMACs have unique structural characteristics that enable their interaction with atomically dispersed metals, coordination atoms on the carriers such as N[40], O[41], S[42], and P[43], and atomic clusters on the carrier, thereby playing a vital role in enhancing catalytic activity and stability. In addition to carbon, SiO2[44] and P[45] can be used as the carrier[46-51]. In SMA catalysts, single atomic sites are often artificially generated to form clusters during pyrolysis, which affects their catalytic activity. However, the catalytic process can be modified using metal clusters, thereby enhancing the catalytic performance of SMACs.
SMACs are synthesized via several methods such as one-step pyrolysis[52], multi-stage pyrolysis[53], including atomic layer deposition (ALD)[54], and pyrolytic-liquid phase loading strategy[55]. Most of these methods use metal precursors (e.g., metal nitrates[56], metal chlorides[57], and metal-organic frameworks (MOFs)[58]) as starting materials. For instance, Xia et al. synthesized the sulfurization of Fe-N-C catalyst containing
Figure 1. Synthesis scheme. (A) FeNC-S-FexC/Fe catalysts. Reproduced with permission from Ref.[59], © WILEY-VCH Verlag GmbH & Co. KGaA, Weinheim 2018.(B) Ir@NBD-C catalysts. Reproduced with permission from Ref.[63], © WILEY-VCH Verlag
Unlike transition metals, noble metals are often loaded onto different carriers via deposition. ALD is a widely used synthesis technique used to effectively obtain the SMA sites of noble metals on carrier materials. Liu et al. obtained monatomic catalysts using multiple ALD techniques to deposit Ni on the SiO2 substrate[44]. The quasi Pd1Ni single-atom surface alloy forming after multiple deposition cycles. In similar research, Liu et al. used Ag as the substrate to deposit palladium diacetylacetonate and trimethylgallium atomic layer under an oxygen environment to obtain Pd SAM catalysts[44]. Thus, these methods can be used for the successful synthesis of SMACs. SMACs can also be synthesized by liquid deposition of pyrolyzed carriers. Xiao et al. prepared SMA via one-step pyrolysis and evenly dispersed them in water. To this,
In addition to the common SMACs synthesis strategies described above, there are many other approaches. For example, Zhang et al. deposited Pt onto MoC using the incipient wetness impregnation method, and then defects in MoC acted as anchor sites to effectively trap Pt atoms[66]. Qiao et al. synthesized Pt1/FeOx catalysts via CO oxidation using Pt[67]. The strong electron transfer between Pt and FeOX makes atomically dispersed Pt a catalytic site for promoting CO oxidation. Similarly, noble metal atoms can effectively bind to defects to form atomically dispersed noble-metal active sites. This synthesis strategy applies to not only metal-based materials, but also nonmetallic substrates. Scheme 2 shows these synthesis methods, among which pyrolysis is widely used. Pyrolysis is the most used method, which has strong universality. However, due to the limitation of metal agglomeration, the heating rate and temperature must be accurately controlled. To this end, multistep pyrolysis or noble metal loading must be used for controlling the catalyst morphology after pyrolysis. ALD can accurately control the atomic layer thickness and the number of exposed SMA sites at low temperatures but at the expense of high synthesis cost, which limits its wide applicability.
INTERACTION MECHANISM OF SINGLE METALLIC ATOM/CLUSTERS ELECTROCATALYSTS
Synergetic effect of single metallic atom/clusters catalysts interactions
The design and fabrication of heterogeneous solid catalysts containing multiple components have attracted research attention[68-71]. Appropriate combination of different elements significantly improves the catalytic performance, which is considerably higher than that of the individual elements. The application of conventional SAM in electrocatalytic energy reactions is limited by their ability to optimize the Gibbs free energy (ΔG) for a single reaction, which affects the electrocatalytic energy reaction kinetics. This challenge can be overcome by the synergistic effect of single-metal active sites and cluster sites, which reduces the energy barrier in multiple reaction steps[72]. In this paper, the synergetic effect is divided into “tandem catalysis” and “concerted catalysis”. Tandem catalysis, as the name suggests, is similar to the series of electrical appliances in a circuit in which the reaction intermediates proceed through the different steps of the catalytic process at different reaction points. Different from tandem catalysis, collaborative catalysis occurs as a result of simultaneous action of multiple components without multiple active sites.
Tandem catalysis
Multi-site catalytic are used for tandem catalysis. In multi-catalytic processes, different catalytic sites are only effective for specific catalytic steps. Using this characteristic, different catalysts can be combined to synthesize catalysts to perform different catalytic steps. Liu et al. fabricated a series of electrodes based on Rh as a single active site and clusters supported on Cu nanowires (Rh@Cu NWs) via the synergistic effect[73], resulting in high catalytic activity. The hydrogen adsorbed on Rh sites is transferred to vicinal NO intermediate species on Cu sites, promoting hydrogenation reaction and ammonia formation [Figure 2A]. To demonstrate the “division of labor” between Rh and Cu sites during catalysis, the formation of hydrogen radicals during HER was monitored by electron paramagnetic resonance (EPR) using dimethyl-1-pyrroline-N-oxide (DMPO) as the radical trapping reagent. Comparison of the EPRs of electrolytes with and without NO3- reveals that H-DMPO is formed in the electrolyte without NO3-, and the same trend is observed for HER performance [Figure 2B]. The H-DMPO signal did not change for the electrolyte containing NO3- and sample Rh nanoparticles (NPs), confirming that Rh NPs are barely active toward nitrate reduction reaction, whereas H* in Rh@Cu-0.6% and Cu NWs are consumed quickly. On the other hand, in situ infrared spectroscopy (IR) results show only a weak characteristic peak corresponding to -NH2 from -0.1 V vs. RHE when pure Cu NWs catalyze NITRR [Figure 2C]. An obvious characteristic peak corresponding to -NH2 is detected from 0.2 V vs. RHE when Rh@Cu-0.6% catalyzes NITRR. This corroborates the EPR results, Rh shows a near-optimal ΔGH*. Thus, the addition of Rh greatly improves H* adsorption on the electrode surface, which can promote the hydrogenation of NO* at the nearby Cu site at a lower application potential. The synergistic effect of Rh and Cu sites was theoretically verified using the density functional theory (DFT) calculation of the stepwise deoxygenation reaction and hydrogenation process, *NO3→*NO2→*N and *NO→*NOH→*NH2OH→*NH3, in the NITRR process [Figure 2D]. The addition of Rh considerably reduces the amount of intermediate *NOH formed, which is the rate-determining step (RDS) of NITRR.
Figure 2. (A) Schematic diagram of the Rh@Cu-0.6% nitrate reduction reaction. (B) Electron paramagnetic resonance (EPR) spectra of the solutions obtained after 3 min of electrocatalysis at -0.1 V vs.. RHE by Cu NWs, Rh@Cu-0.6% and Rh NPs loading on carbon cloth in 0.1 M Na2SO4 electrolyte with/without 0.1 M KNO3 under argon. (C) Electrochemical in situ infrared spectroscopy (IR) of Rh@Cu-0.6% and Cu NWs with different potentials at 0.1 M Na2SO4 electrolyte with 0.1 M KNO3. (D) Gibbs free energy diagram of various intermediates generated during electrocatalytic NITRR over the pure Cu NWs and Rh@Cu-0.6%, it is assumed that all Rh in
SMACs show great potential as electrocatalysts for fundamental reactions, such as ORR and OER, in proton-exchange membrane fuel cells (PEMFCs). Ding et al. reported the synthesis of a bi-functional oxygen electrocatalyst by combining FeSA and NiCo NPs in carbon nanotubes (CNTs) for fabricating efficient and long-life rechargeable ZABs[74]. Similar to PEMFCs, ZABs use ORR and OER. The WT contour plot of FePc||CNTs||NiCo/CP is similar to that of FePc, implying that the N coordination of Fe atoms exists as a single atom. In addition, the FT k3-weighted EXAFS and Fe K-edge XANES spectra of FePc||CNTs||NiCo/CP demonstrated that Fe in the sample forms a Fe-N bond instead of a Fe-Fe bond, indicating the existence of Fe atom site [Figure 2E]. Electrochemical performance tests of ZABs revealed ORR and OER as the two central reactions, with the ORR half-wave potential (E1/2@ORR) of 0.902 V, which is significantly higher than that of the Pt/C catalyst (0.842 V), and the OER potential (Ej = 10) of 1.588 V at a current density of 10 mA cm-2, which is considerably better than that of the Ir/C catalyst (1.608 V). The excellent oxygen electrocatalytic properties are due to the presence of FeSA and NiCo hydroxide sites, which accelerate the ORR and OER, respectively.
Guo et al. reported that the initial Pt1.5Ni NC undergoes a dealloying process driven by ammonia and heat, as the nickel atoms are continuously separated from the substrate to form a stable Pt surface Pt1.5Ni1-x alloy[75]. Subsequently, these Ni atoms will be captured by adjacent defects on the carbon substrate, resulting in many NiSA sites that are closely distributed around the de-alloyed Pt1.5Ni1-x. The morphologies of PtNi alloys at different stages can be observed via morphological characterization in addition to the valence states and coordination environments of Ni and Pt. The white line intensity of the Ni K-edge gradually increased and the absorption threshold position shifted toward Ni3+, indicating that Ni was further oxidized. The half-wave potential (E1/2) of Pt1.5Ni1-x/Ni-N-C in 0.1 M HClO4 solution is 0.967 V, which is higher than that of the commercial Pt/C (0.887 V). Figure 2F shows the synergistic effect of different sites within the Pt1.5Ni1-x/Ni-N-C, indicating superior ORR performance. The yellow arrow in the figure shows the reaction pathway A, where ORR occurs via a four-electron transfer pathway at the Pt site to yield H2O. A two-electron transfer reaction occurs at the Ni-N-C site to produce hydrogen peroxide (path C). However, for the NiSA sites near the Pt1.5Ni1-x, the resulting OOH* is directly adsorbed by the Pt site, undergoing further reaction to form
Concerted catalysis
Using the Ru-alginate metal-organic supramolecular conversion method, Zhang et al. synthesized an electrocatalyst containing both Ru single atoms (SAs) and NPs anchored on defective carbon (RuSA+NP/DC)[76]. XAS spectra of RuSA+NP/DC, metallic Ru, and RuO2 proved that RuSA+NP/DC contains Ru-Ru metallic bonds and Ru-C bonds. Differential charge density distributions revealed that the charge is transferred from the Ru atom to the H2O molecule [Figure 3A]. This charge transfer significantly elongates the H-O bond of the adsorbed water to 0.983 Å, implying that Ru NPs have an important positive promoting effect on hydrolytic splitting. H* adsorption energies of different Ru catalysts were calculated. The volcano plot
Figure 3. (A) Differential charge density distributions of active Ru atom for RuNP@DC-1 and adsorbed H2O. (B) Volcano plot of theoretical ηHERvs. ΔEH*. (C) Kinetic barrier of H2O dissociation for different models. Reproduced with permission from Ref.[76],
Yang et al. designed a novel catalyst containing Ir NPs and atomic Ir sites (IrNP@IrSA-N-C), which could effectively address the CO poisoning problem of the catalyst[78]. The EXAFS signals of IrNP@IrSA-N-C show the existence of IrNP and IrSA [Figure 3E and F]. The atomic Ir site, a good CO oxidation site, efficiently removes CO molecules adsorbed on IrNP in a short time. Thus, IrNP retains part of the active site, which is conducive to promoting H2 oxidation. The interaction between IrNP and IrSA centers enhances the H2 oxidation activity of the catalyst and the electrooxidation activity of CO in PEMFCs [Figure 3G].
Charge redistribution effect of single metallic atom/clusters electrocatalysts
The redistribution of charge on the catalyst surface is the design idea for most catalysts, particularly SMACs. Charge redistribution can change the charge density of active sites and thus change the charge distribution around the Fermi level[79-82]. The d-band center of the active core metal can also be changed to optimize the reaction path and activation energy. Moreover, the reaction process can be optimized by changing the adsorption-desorption strength of the reaction intermediates through strong electron coupling between different active sites. The spin state of the active site can be modified by charge redistribution to further accelerate energy electrocatalysis[83].
Charge transfer
Xia et al. showed the fabrication of Fe-N-C graphitic layer-encapsulating Fe3C species within carbon nanosheets (Fe-N-C/Fe3C@HCNs) via one-step pyrolysis[60]. The SMA active sites can be clearly observed by morphological characterization. In addition, the NEXAFS shows that the coordination forms of Fe in the catalyst system are Fe-N, Fe-Fe, and Fe-C, respectively, which proves the existence of the Fe-N-C site and Fe3C. Figure 4A and B show the density of state (DOS) illustrations of Fe-N-C/Fe3C and Fe-N-C. Compared with Fe-N-C, the electron states near the Fermi level of Fe-N-C/Fe3C increase significantly under pre- and post-treatment conditions. This indicates that the electron mobility in Fe-N-C/Fe3C is improved, which significantly reduces the charge transfer resistance. Figure 4C shows the schematic after long-life cycles, which can be proved to be structurally complete without collapse through the image of an electron microscope.
Figure 4. (A and B) DOS illustrations of the Fe-N-C/Fe3C, Fe-N-C. The dashed line at 0 eV indicates the Fermi level (the inset is a charge density difference diagram). (C) The schematic illustration of Na+ storage mechanism of Fe-N-C/Fe3C@HCNs, and structure changes during discharge/charge process. Reproduced with permission from Ref.[60], © Tsinghua University Press 2021.
For SMACs, the size of clusters will considerably affect the degree of electron transfer and the catalytic performance. Huang et al. synthesized Fe-based metal-organic frameworks (Fe-MOFs) with nanocube structures using the co-precipitating method[84]. The Fe-MOFs were then coated by polydopamine via the self-polymerization of the dopamine monomer in alkaline conditions. Then, two-step pyrolysis was performed under ammonia and Ar gas flows successively to increase the N content and enhance carbonization, respectively. The cubic morphologies of Fe atomic cluster functionalizing dispersed metal single-atoms (Fe-ACSA@NC) and Fe NPs functionalizing dispersed metal single-atoms (Fe-NPSA@NC) were confirmed via structural characterization. Using XPS spectra and Fourier transform k3-weighted Fe K-edge EXAFS spectra analysis of Fe, the coordination modes of Fe in the sample were found to be Fe-Fe and Fe-N, corresponding to Fe nanoclusters and Fe-N-C sites, respectively. By comparing the differential charge density plots of Fe-ACSA@NC and Fe-NPSA@NC, it was found that the decoration of nano-sized Fe clusters induced electron redistribution around the Fe-N active site [Figure 4D and E]. The electrons around the metal Fe sites were transferred to the adjacent carbon matrix, making the Fe sites more positive and weakening the OH* adsorption energy. Analysis of the relationship between the Bader charge and OH* adsorption energy [Figure 4F] revealed that the OH* adsorption energy has a linear relationship with the Bader charge; Fe-NPSA@NC with a Bader charge of 0.64 V shows the strongest OH* adsorption energy. In contrast, Fe-ACSA@NC with a higher Bader charge showed the weakest OH* binding tendency, promoting the desorption of OH* and thus preserving the active site for subsequent reactions.
Similar to the above work, Chen et al. prepared a spindle-like Fe/FexC@Fe-N-Cs [Figure 4G][85]. The Gibbs free-energy diagrams of the four-electrons pathway of ORR on the Fe-N4-Fe10 models are shown in Figure 4H. When the external voltage is 0 (U = 0), the free energy of both models appears downhill, indicating that the reaction is exothermic and spontaneous. The Fe-N4-Fe10 model exhibits a lower overpotential (ƞ = 0.27 V) in the RDS than that of the Fe-N4 structure, which has a more efficient ORR activity on Fe-N4-Fe10. Fe-N4-Fe10 has a higher half-wave potential (E1/2 = 0.81 V), implying that its ORR activity is higher [Figure 4I]. Due to the presence of Fe10, a considerable amount of electron is transferred, which significantly affects the ORR process (especially OOH* production), the catalytic effect occurs due to this activity.
Strong electron coupling
In SMACs, the presence of clusters directly affects the distribution of electrons on the catalyst surface. Charge distribution occurs throughout the d-band center of the catalyst, and its effect on the catalytic process cannot be ignored. Yao et al. used F127 as a surfactant for the self-assembly of 3-aminophenol and hexamethylenetetramine in a solution. They then conducted annealing carbonization to obtain Ru SA coupling with ultrafine nanoclusters on hierarchical porous N-doped carbon (NMC-RuSA+NC)[86]. DFT and experimental results reveal the existence of a strong electron coupling effect between Ru SA and nanoclusters. As shown in Figure 5A, by comparing the positions of the d-band centers of RuN4,
Figure 5. (A) Projected density of states (PDOS) of Ru on the above three Ru systems and the schematic illustration between the
Spin-state regulation
Regulation of spin states has recently played a vital role in the development of catalysts[89]. Reasonable spin states can greatly improve catalytic activity[90-93]. Wei et al. synthesized an unprecedented ORR catalyst consisting of Pd nanoclusters (PdNC) and Fe single-atoms (Fe-N-C/PdNC) via the dual-confinement effect of ZIF-8[94]. The XAS spectra show that the valence state of Fe species in Fe-N-C/PdNC and Fe-N-C is between FePc(II) and Fe2O3. The D1, D2 and D3 peaks in 57Fe Mossbauer spectra belong to low spin (LS), media spin (MS), and high spin (HS), respectively. Relative to Fe-N-C, the D2 peak in Fe-N-C/PbNC increased from 19.64% to 40.32%. The strong interaction between FeSA and PdNC effectively modifies the electronic structure of Fe, the dz2 orbit is perpendicular to the Fe-N-C plane, and the original empty dz2 orbit of LS Fe (II) becomes a partially occupied orbit in the MS structure, which can effectively adjust the orbital overlap with the oxygen-containing intermediate, such that Fe-N-C/PdNC has high catalytic activity. With PdNC loading, the electron transfer from Fe SAs to PdNC leads to the redistribution of Fe 3d-orbital electrons [Figure 6A]. Further details of different orbitals show that this mainly originates from the increasing spin state of Fe dz2 orbital [Figure 6B]. Fe-N-C/PdNC exhibits a high E1/2 of 0.94 V in 0.1 M KOH, indicating that Fe-N-C/PdNC has a better ORR performance [Figure 6C]. As shown in Figure 6D, spin density diagrams of Fe-N-C and Fe-N-C/PdNC revealed the reason of its enhanced ORR activity. Fe sites with higher spin-states in
Figure 6. (A) The charge density difference of Fe-N-C/PdNC (top: top view; bottom: front view. The yellow and cyan regions represent electron accumulation and depletion, respectively). (B) Projected DOS diagrams of Fe-N-C and Fe-N-C/PdNC. (C) ORR polarization curves measured on RDE in O2-saturated 0.1 M HClO4 electrolyte for different catalysts. (D) Spin density diagrams of Fe-N-C and Fe-N-C/PdNC (the isosurface is 0.1 a.u.). (E) Tafel plots of catalysts. Reproduced with permission from Ref.[94], © Elsevier Ltd. 2022. (F) Calculated relationship between μB and ΔEads (O2) of FeN3O and FeN3O-O-Ti. (G) The PDOS of Fe 3d orbital for FeN3O and FeN3O-O-Ti and (H) The free energy changes for ORR. The blue dotted lines denote the d-band centers, while the gray dotted line denotes the Fermi level. Reproduced with permission from Ref.[95], © WILEY-VCH Verlag GmbH & Co. KGaA, Weinheim 2022.
To regulate the spin-states of the active center of the catalyst, Liu et al. developed an axial Fe-O-Ti ligand-regulated spin-state transition strategy to improve the ORR activity of the Fe sites[95]. By bridging Fe SAs to Ti3C2Tx, FeN3O-O-Ti was obtained. Compared with FeN3O, the formation of FeN3O-O-Ti significantly increased the amount of charge transfer. Figure 6F shows the increase in the magnetic moment of the Fe site from 1.51 to 3.52 μB, proving that the spin state of the site was from LS to MS, which greatly improved the adsorption energy with O2 (ΔEads). In contrast, compared with the calculated PDOS of the Fe 3d orbital [Figure 6G], the d-band center of FeN3O-O-Ti was further away from the Fermi level. Thus, the adsorption energy of the oxygenated intermediate could be appropriately adjusted, which is crucial for the ORR process. This conclusion is also supported by the free-energy calculations for the ORR process. FeN3O must overcome a large energy barrier to form OOH* [Figure 6H].
Electronic metal-support interaction
The electronic metal-support interaction (EMSI) mechanism is based on the metal-support interaction (MSI)[96-98]. As the support height increases, the EMSI decreases and leads to a significant difference between the atoms at the top and bottom of the nanoclusters (known as tip effect, TE)[99-103]. The local electric field from the tip promotes mass transfer. In addition, the tip often plays an important role in the catalytic reaction due to the synergistic effect of the enhanced electric field effect and the local electric field effect. This results in a suitable adsorption strength of the reversible intermediate. EMSI can be used as a tool to regulate the electronic structure of the metal active site. EMSI is usually so strong that the efficiency of the active site is determined by the metal center and adjacent atoms. It has a flexible electronic environment and a high electron transfer rate; however, it has high adsorption energy and energy barrier.
The size of the cluster greatly affects the charge distribution on the catalyst surface. The smaller the cluster size, the more significant the charge redistribution in the matrix[104]. Therefore, the surface charge distribution of the cluster must be maintained within an appropriate range of reaction by controlling the cluster size. Yang et al. designed an IrO2 sub-nano cluster catalyst supported by titanium carbide NPs
Figure 7. (A) The crystal orbital Hamilton population of the interaction between the Ir atoms of different heights and the Ti atoms on the surface of the Irn-TiC. (B) The electron localization function of the Ir1-TiC. (C) The charge density difference of the Ir1-TiC. (D) Synthesis schematic of Mo SAs on a 1T-CrS2 nanoflake by L-MBE. Reproduced with permission from Ref.[105], © WILEY-VCH Verlag GmbH & Co. KGaA, Weinheim 2022. (E) Schematic of electric field distribution of Mo SAs@1T-CrS2 nanoflake. (F) The calculated free-energy diagram of HER at the equilibrium potential for Mo SAs@1T-CrS2, 1T-CrS2 and Pt. Reproduced with permission from Ref.[106],
Similarly, when the cluster size is fixed, the atomic cluster will be regulated by the charge redistribution from the cluster. Zhou et al. developed Mo SAs@1T-CrS2 nanoflake through the simple synthesis of laser-molecular beam epitaxy (L-MBE) technology[106]. As shown in Figure 7D, the Mo atom can be successfully anchored to the metal substrate of 1T-CrS2 nanosheet by S atom under the resulting Mo plasma, which can induce TE by local electric field. Fourier transform of the k3-weighted Mo K-edge of the EXAFS spectra can prove that the coordination form for Mo is Mo-S. Mo SAs@1T-CrS2 shows excellent electrocatalytic performance and ultra-high stability, which is superior to 1T-CrS2. The schematic of electric field distribution of Mo SAs@1T-CrS2 nanoflake is shown in Figure 7E. This figure shows that the charge density around Mo atom increases, enhancing the coupling of H*. By comparing the calculated free-energy diagram of HER at the equilibrium potential for Mo SAs@1T-CrS2, 1T-CrS2, and Pt, it can be found that the ΔGH* of Mo SAs@1T-CrS2 is lower than that of 1T-CrS2 [Figure 7F]. Thus, it is theoretically proved that atomic Mo on the 1T-CrS2 substrate plane is the active sites of efficient HER.
In summary, most of the research on changes in charge transfer, spin state, or EMSI caused by electric charge distribution comes from theoretical calculations. It is worth noting that SMACs, as a relatively complex catalytic system, may contain many more active sites than the structures calculated by researchers. Therefore, it is limited to prove the active site and the catalytic reaction process at the active site only by calculations. To support the theoretical calculation results, a more accurate characterization of the charge transfer/electron coupling/spin states is needed as experimental evidence. For example, the number of unpaired electrons of the material can be detected by electron paramagnetic resonance (EPR), and the magnetic moment of the material can be detected by vibrating sample magnetometer (VSM) to evaluate the change of spin state, etc. This is also another requirement for the characterization of materials.
Mutual assistance of single metallic atom/clusters electrocatalysts
SMACs contain multiple reaction sites; however, not all sites are involved in the catalytic process[107]. This does not mean that the presence of these phases is meaningless; on the contrary, it may be crucial for the actual catalytic sites. The interaction between SMA and metal clusters can regulate the electronic and geometric structure of the active sites, change the adsorption energy of intermediates, and ultimately affect the reaction pathway. It is one of the advanced design strategies for synthesizing high-performance catalysts, combining the advantages of different catalytic sites and exploiting the characteristics of each metal active site to improve the catalytic efficiency. Researchers have recently developed catalysts containing different catalytic actions, providing a platform for understanding the mutual assistance within catalysts at a molecular level[14].
Single atom assisted cluster site
SMA carriers consist of multiple elements. The coordination environment at SMA sites is diverse and difficult to control, making it difficult to achieve specific bonding reactions (e.g., C-C coupling). As shown in Figure 8A, Ou et al. catalyzed C-C coupling by loading Au SAs on a red phosphorus (RP) catalyst
Figure 8. (A) Interface-assisted catalytic C-C Coupling of Au single-atoms over red phosphorus. Photocatalytic reduction of CO2 to ethane. (B) Wavelet-transformed k3-weighted EXAFS spectra of samples. (C) Schematic illustration of Au1/RP for C-C coupling. Reproduced with permission from Ref.[43], © American Chemical Society 2022. (D) Optimized structures of Pt/Fe-N-C, Pt/N-C, and Pt/C, along with projected crystal orbital Hamilton population analysis. Reproduced with permission from Ref.[65], © American Chemical Society 2022. (E) Charge transfer process in Os-OsSe2. (F) The DOS of Os, OsSe2, and Os-OsSe2. Reproduced with permission from Ref.[4], © WILEY-VCH Verlag GmbH & Co. KGaA, Weinheim 2022.
Work function (WF), as a parameter reflecting electron escape ability, is widely used to reveal the electron transfer process in heterogeneous catalysts. Chen et al. constructed Os-OsSe2 heterostructures with molten salt-assisted synthesis strategy, in which the WF determined the built-in electric field and the direction of charge transfer [Figure 8E][4]. As the cheapest metal in the Pt group, Os has an extremely strong binding energy to H*; thus, it cannot be used as a catalyst for HER. The built-in electric field drives the transfer of electric charges from Os to OsSe2 due to the differences in WFs on both sides of the Os-OsSe2 heterogeneous structure. The resulting interfacial equilibrium significantly weakened the adsorption strength of Os to H*. Os-OsSe2 had the highest density of states near the Fermi level, as shown in Figure 8F. Similarly, SMACs charge transfer resulting from differences in WFs is the internal driving mechanism of SMACs catalysts.
Cluster assisted single atom site
So far, Fe-N-C is one of the widely used catalysts[108]. However, due to thermodynamic instability, Fe atoms tend to migrate and coalesce into NPs during pyrolysis[109]. This makes high loading of atomic Fe catalyst difficult. However, the presence of clusters does not only have negative effects on Fe-N-C catalyst[108].
Figure 9. (A) Free-energy path and (B) calculated overpotentials of the ORR for the Fe1@FeSA-N-C, Fe13@FeSA-N-C, FeNP@FeSA-N-C, and FeSA-N-C models. (C) LSV curves of FeAC@FeSA-N-C, FeSA-N-C, N-C, and Pt/C in O2-saturated 0.1 M KOH solution with a rotation rate of 1,600 rpm and a sweep rate of 10 mV s-1. Reproduced with permission from Ref.[110], © American Chemical Society 2019. (D) Fe-N radical distribution function profiles of the Fe-N4 moiety in the models of bare Fe-N4 and Fe-N4/Fe4-N6 at 25 and 80 °C. Wavy arrows are used to indicate the amplitude of Fe-N bond-length fluctuation. (E) Snapshots of Fe-N4 and Fe-N4/Fe4-N6 obtained from MD simulations at 80 °C. The initial configuration (left) and an intermediate state (right) are provided to show the elongation of the Fe-N bond as marked by the yellow box. (F) Time-resolved CO2 formation after exposure to continuous CO flow and (G) in situ DRIFTS of CO adsorption. Reproduced with permission from Ref.[112], © American Chemical Society 2022.
Achieving enhanced catalytic effect by inhibiting transition metal leaching as well as balancing stability and activity are crucial for practical applications of proton-exchange membrane fuel cells. Wan et al. used protonated N-doped carbon substrates to produce moderate coordination strengths for metallic elements during heat treatment, thereby introducing Fe clusters and thus achieving uniform dispersion of FeSA and FeNP[111]. Experimental and theoretical evidence shows strong electronic interactions between FeSA and FeNP due to the smooth electron transfer pathway and very short interaction distances. The RDS of ORR on
Xie et al. succeeded in enriching defective CeO2-Al2O3 carriers with different local coordination environments on the surface for atomic Pt (Pt1) and Pt atomic single-layer (PtASL) and Pt multilayer cluster (PtC) catalysts with 100% metal dispersion and precisely controlled local coordination environments using a surface defect enrichment strategy[112]. To determine the reactive oxygen species and better understand the advantages of embedded PtASL (PtASL-e), the in-situ DRIFT study at 100 °C to monitor the reaction of CO with oxygen in PtASL/CA and PtASL/CA-e catalysts [Figure 9F and G]. Compared with the intense CO formation peak observed on PtASL/CA, a lower CO formation peak was observed on PtASL/CA-e, indicating that less surface oxygen is present on PtASL/CA-e. The oxygen vacancies of CeO2-Al2O3 effectively promote the synthesis of Pt materials at different atomic levels, bringing positive effects for the subsequent adsorption and oxidation of CO.
CONCLUSION AND OUTLOOK
In most single metallic atom/clusters (SMACs) catalysts, single metallic atom (SMA) sites are the main active sites, while the presence of clusters often serves as a matrix. Precise regulation of the coordination environment of the single metallic atom and the change in electronic structure induced by the introduction of clusters, as well as appropriate regulation of SMACs interactions, are important ways to optimize the catalytic activity of SMACs. Although SMA catalysts have outstanding advantages in terms of improving atomic utilization and reducing cost, their catalytic activity and stability cannot be guaranteed if the structural stability of single atoms cannot be ensured. Therefore, SMAs and clusters are combined to obtain advanced catalysts with high catalytic activity and stability as well as improved atomic utilization.
In this review, SMACs based on their synthesis methods and catalytic reaction mechanisms. The mechanisms by which various SMACs play a role in the catalytic process and the influence of these mechanisms on their catalytic performance are discussed along with the synergistic effect of carriers and clusters in the catalytic process. Machine learning is gaining popularity in the field of catalysis. Artificial intelligence, which combines Big Data and machine learning for catalyst selection and design, has significantly accelerated the related research. A machine learning framework was used to improve computational speed and obtain catalysts with high catalytic activity. The as-obtained catalysts were matched with the experimental results to create an efficient catalyst design[113,114]. SMACs are being extensively researched. However, due to limitations in characterization techniques, their electronic structure and reaction dynamics cannot be monitored, which results in an insufficient understanding of their reaction kinetics. To understand the reaction mechanism and kinetics of SMACs and to synthesize SMACs with high catalytic activity, more accurate in-situ characterization techniques and computational simulations are required. Thus, many crucial challenges have to be addressed [Scheme 3].
Scheme 3. Proposed future directions towards advanced single metallic atom/clusters catalyst in energy electrocatalysis.
(I) Synthesize of SMACs with high efficiency and stability. The technology to synthesize SMACs is not yet mature and is more challenging than those used for the synthesis of SMAs and cluster catalysts. Computational models can be used for catalysis; however, using theoretical models due to their limited precision and controllability for material synthesis. Strategies that enable accurate synthesis of SMACs, such as ALD, are expensive and involve matrix selection; thus, they cannot be readily applied on a large scale. A universal and accurate synthesis strategy is required for the development of synthesis methods such as the loading of metal clusters with controllable sizes onto different SMA matrixes or the loading of single atoms with controllable site density onto different metal clusters. Notably, metal cluster loading and morphology-controllable synthesis are promising methods for catalyst development.
(II) Identification of truly active sites in SMACs and dynamic changes during catalysis as well as the analysis of the formation and disappearance of reaction intermediates: Advanced characterization methods are used to simultaneously determine the active sites and monitor catalyst transformation under working conditions. The combination of in situ and operando structural characterization (e.g., in-situ Mössbauer spectroscopy, online mass spectrometry, ultrafast spectroscopy, and operando XAS) is an important tool for understanding the activation and deactivation of catalyst sites. For example, in actual catalytic studies, the formation, transformation and disappearance of reaction intermediates in the system can be clearly understood by in-situ FTIR. At the same time, in-situ Raman can be used to characterize the change in catalyst composition at the same time. Combining the two results gives insight into the catalytic process. This is necessary to understand the mechanism of the reaction. Future research should focus on developing complete and functional in-situ and operando instrumentation for complex reaction systems.
(III) Artificial intelligence is of guiding significance to the design of catalysts. As mentioned above, the existence of big data can analyze current research hotspots, but it is still difficult for researchers to accurately choose these huge data. Through artificial intelligence training, the research hotspot can be sorted out and the structure of catalyst can be predicted. On this basis, combined with the interest of researchers, the catalyst is screened. In conclusion, using artificial intelligence for prediction and pre-selection is an interesting option for reducing researchers' workload and improving research efficiency.
(IV) Simulation of the complexity and dynamics of catalytic systems using computational modeling. Similar to machine learning, theoretical calculations can guide catalyst design, and the calculation process is not constrained by practical conditions, so impractical solutions can be ruled out in advance. However, theoretical calculation results may not be realized. On the one hand, the synthesis of prefabricated catalysts may fail due to limited resources; on the other hand, the validity of theoretical calculations can only be proven when the results are verified via structural, morphological, and physicochemical characterization techniques. Therefore, reasonable theoretical calculations can distinguish catalysts with different structures to determine reaction pathways and active reaction sites.
Understanding and developing multiatom cluster catalysts is fraught with challenges and opportunities. Combining SMAs and metal clusters is critical to the development of new-generation catalyst systems. SMACs are used not only in electrocatalysis but also in thermal and environmental catalysis. Its potential in pollution control, renewable energy, carbon neutrality, and other industries should help alleviate global energy and environmental problems. In the future, efficient SMACs will be developed to optimize industrial production processes, reduce production costs, and prevent pollution.
DECLARATIONS
Authors’ contributionsProposed the topic of this review: Zhang JN
Prepared the manuscript: Wei Y, Xia H
Collectively discussed and revised the manuscript: Wei Y, Xia H, Yan W, Zhang JN
Availability of data and materialsNot applicable.
Financial support and sponsorshipThis work was supported by the National Natural Science Foundation of China (U22A20107) and the Distinguished Young Scholars Innovation Team of Zhengzhou University (32320275). The Science and Technology R&D Program Joint Fund Project of Henan Provincial (222301420001), and Postgraduate Education Reform Project of Henan Province (2021SJGLX093Y).
Conflicts of interestAll authors declared that there are no conflicts of interest.
Ethical approval and consent to participateNot applicable.
Consent for publicationNot applicable.
Copyright© The Author(s) 2023.
REFERENCES
2. Pomerantseva E, Bonaccorso F, Feng X, Cui Y, Gogotsi Y. Energy storage: the future enabled by nanomaterials. Science 2019;366:eaan8285.
3. Xiao Y, Xu R, Xu L, Ding J, Huang J. Recent advances in anion-derived SEIs for fast-charging and stable lithium batteries. Energy Mater 2022;1:100013.
4. Chen D, Lu R, Yu R, et al. Work-function-induced interfacial built-in electric fields in Os-OsSe2 Heterostructures for Active Acidic and Alkaline Hydrogen Evolution. Angew Chem Int Ed 2022;61:e202208642.
5. Deng J, Ren P, Deng D, Bao X. Enhanced electron penetration through an ultrathin graphene layer for highly efficient catalysis of the hydrogen evolution reaction. Angew Chem Int Ed 2015;54:2100-4.
6. Zhu J, Guo Y, Liu F, et al. Regulative electronic states around ruthenium/ruthenium disulphide heterointerfaces for efficient water splitting in acidic media. Angew Chem Int Ed 2021;60:12328-34.
7. Pan F, Jin T, Yang W, et al. Theory-guided design of atomic Fe-Ni dual sites in N,P-co-doped C for boosting oxygen evolution reaction. Chem Catal 2021;1:734-45.
8. Liu S, Li C, Zachman MJ, et al. Atomically dispersed iron sites with a nitrogen-carbon coating as highly active and durable oxygen reduction catalysts for fuel cells. Nat Energy 2022;7:652-63.
9. Wang X, Fu N, Liu JC, et al. Atomic replacement of PtNi nanoalloys within Zn-ZIF-8 for the fabrication of a multisite CO2 reduction electrocatalyst. J Am Chem Soc 2022;144:23223-9.
10. Xue D, Xia H, Yan W, Zhang J, Mu S. Defect engineering on carbon-based catalysts for electrocatalytic CO2 reduction. Nanomicro Lett 2020;13:5.
11. Zhang Y, Gao P, Jiao F, et al. Chemistry of ketene transformation to gasoline catalyzed by H-SAPO-11. J Am Chem Soc 2022;144:18251-8.
12. Garrido-Barros P, Derosa J, Chalkley MJ, Peters JC. Tandem electrocatalytic N2 fixation via proton-coupled electron transfer. Nature 2022;609:71-6.
13. Li Z, Deng Z, Ouyang L, et al. CeO2 nanoparticles with oxygen vacancies decorated N-doped carbon nanorods: a highly efficient catalyst for nitrate electroreduction to ammonia. Nano Res 2022;15:8914-21.
14. Xia H, Zan L, Wei Y, et al. Catalytic effect of carbon-based electrode materials in energy storage devices. Sci China Mater 2022;65:3229-42.
15. Qing G, Ghazfar R, Jackowski ST, et al. Recent advances and challenges of electrocatalytic N2 reduction to ammonia. Chem Rev 2020;120:5437-516.
16. Zhou L, Chen L, Ding Z, et al. Enhancement of interfacial catalysis in a triphase reactor using oxygen nanocarriers. Nano Res 2021;14:172-6.
17. Liu M, Lei Z, Ke Q, et al. Regulation of hydrogen evolution performance of titanium oxide-carbon composites at high current density with a Ti-O hybrid orbital. Carbon Energy 2022;4:480-90.
18. Chen X, Qiao Z, Hou B, et al. Chiral metal-organic frameworks with tunable catalytic selectivity in asymmetric transfer hydrogenation reactions. Nano Res 2021;14:466-72.
19. Zhang X, Zhu X, Bo S, et al. Identifying and tailoring C-N coupling site for efficient urea synthesis over diatomic Fe-Ni catalyst. Nat Commun 2022;13:5337.
20. Wan X, Shui J. Exploring durable single-atom catalysts for proton exchange membrane fuel cells. ACS Energy Lett 2022;7:1696-705.
21. Hu J, Xu Q, Wang X, et al. Charge-transfer-regulated bimetal ferrocene-based organic frameworks for promoting electrocatalytic oxygen evolution. Carbon Energy 2023;5:e315.
22. Guo Y, Wang M, Zhu Q, Xiao D, Ma D. Ensemble effect for single-atom, small cluster and nanoparticle catalysts. Nat Catal 2022;5:766-76.
23. Cheng N, Stambula S, Wang D, et al. Platinum single-atom and cluster catalysis of the hydrogen evolution reaction. Nat Commun 2016;7:13638.
24. Kim J, Yoo JM, Lee HS, Sung Y, Hyeon T. Single-atom M-N-C catalysts for oxygen reduction electrocatalysis. Trends Chem 2021;3:779-94.
25. Tang X, Wei Y, Zhai W, et al. Carbon nanocage with maximum utilization of atomically dispersed iron as efficient oxygen electroreduction nanoreactor. Adv Mater 2023;35:e2208942.
26. Song LN, Zhang W, Wang Y, et al. Tuning lithium-peroxide formation and decomposition routes with single-atom catalysts for lithium-oxygen batteries. Nat Commun 2020;11:2191.
27. Han L, Cheng H, Liu W, et al. A single-atom library for guided monometallic and concentration-complex multimetallic designs. Nat Mater 2022;21:681-8.
28. Xia H, Qu G, Yin H, Zhang J. Atomically dispersed metal active centers as a chemically tunable platform for energy storage devices. J Mater Chem A 2020;8:15358-72.
29. Tian Z, Zhang Y, Zhu J, Li Q, Liu T, Antonietti M. A reanalysis of the diverse sodium species in carbon anodes for sodium ion batteries: a thermodynamic view. Adv Energy Mater 2021;11:2102489.
30. Kumar K, Asset T, Li X, et al. Fe-N-C electrocatalysts’ durability: effects of single atoms’ mobility and clustering. ACS Catal 2021;11:484-94.
31. Patniboon T, Hansen HA. Acid-stable and active M-N-C catalysts for the oxygen reduction reaction: the role of local structure. ACS Catal 2021;11:13102-18.
32. Rojas-carbonell S, Artyushkova K, Serov A, Santoro C, Matanovic I, Atanassov P. Effect of pH on the activity of platinum group metal-free catalysts in oxygen reduction reaction. ACS Catal 2018;8:3041-53.
33. Wu Z, Shen J, Li C, et al. Mo2TiC2 MXene-supported Ru clusters for efficient photothermal reverse water-gas shift. ACS Nano 2022;17:1550-9.
34. Wang Y, Guo T, Tian Z, Bibi K, Zhang YZ, Alshareef HN. MXenes for energy harvesting. Adv Mater 2022;34:e2108560.
35. Liu H, Jiang L, Khan J, et al. Decorating single-atomic mn sites with FeMn clusters to boost oxygen reduction reaction. Angew Chem Int Ed 2023;62:e202214988.
36. Ye C, Zheng M, Li Z, et al. Electrical pulse induced one-step formation of atomically dispersed Pt on oxide clusters for ultra-low-temperature zinc-air battery. Angew Chem Int Ed 2022;61:e202213366.
37. Yang L, Li G, Ma R, et al. Nanocluster PtNiP supported on graphene as an efficient electrocatalyst for methanol oxidation reaction. Nano Res 2021;14:2853-60.
38. Yin H, Xia H, Zhao S, Li K, Zhang J, Mu S. Atomic level dispersed metal-nitrogen-carbon catalyst toward oxygen reduction reaction: synthesis strategies and chemical environmental regulation. Energy Environ Mater 2021;4:5-18.
39. Zhao S, Yin H, Xia H, et al. The assembling principle and strategies of high-density atomically dispersed catalysts. Chem Eng J 2021;417:127917.
40. Wu Z, Zhao Y, Xiao W, et al. Metallic-bonded Pt-Co for atomically dispersed Pt in the Co4N Matrix as an efficient electrocatalyst for hydrogen generation. ACS Nano 2022;16:18038-47.
41. Yang Y, Yang Q, Yang Y, et al. Enhancing water oxidation of Ru single atoms via oxygen-coordination bonding with NiFe layered double hydroxide. ACS Catal 2023;13:2771-9.
42. Chen J, Huang B, Cao R, et al. Steering local electronic configuration of Fe-N-C-based coupling catalysts via ligand engineering for efficient oxygen electroreduction. Adv Funct Mater 2023;33:2209315.
43. Ou H, Li G, Ren W, et al. Atomically dispersed Au-assisted C-C coupling on red phosphorus for CO2 photoreduction to C2H6. J Am Chem Soc 2022;144:22075-82.
44. Liu F, Xia Y, Xu W, et al. Integration of bimetallic electronic synergy with oxide site isolation improves the selective hydrogenation of acetylene. Angew Chem Int Ed 2021;60:19324-30.
45. Guo Y, Yuan P, Zhang J, et al. Co2P-CoN double active centers confined in N-doped carbon nanotube: heterostructural engineering for trifunctional catalysis toward HER, ORR, OER, and Zn-air batteries driven water splitting. Adv Funct Mater 2018;28:1805641.
46. Sun Q, Chen BWJ, Wang N, et al. Zeolite-encaged Pd-Mn nanocatalysts for CO2 hydrogenation and formic acid dehydrogenation. Angew Chem Int Ed 2020;59:20183-91.
47. Liang D, Wang Y, Chen M, et al. Dry reforming of methane for syngas production over attapulgite-derived MFI zeolite encapsulated bimetallic Ni-Co catalysts. Appl Catal B Environ 2023;322:122088.
48. Fang JY, Zheng QZ, Lou YY, et al. Ampere-level current density ammonia electrochemical synthesis using CuCo nanosheets simulating nitrite reductase bifunctional nature. Nat Commun 2022;13:7899.
49. Aitbekova A, Zhou C, Stone ML, et al. Templated encapsulation of platinum-based catalysts promotes high-temperature stability to 1,100 °C. Nat Mater 2022;21:1290-7.
50. Chi X, Li M, Di J, et al. A highly stable and flexible zeolite electrolyte solid-state Li-air battery. Nature 2021;592:551-7.
51. Zhao H, Yu R, Ma S, et al. The role of Cu1-O3 species in single-atom Cu/ZrO2 catalyst for CO2 hydrogenation. Nat Catal 2022;5:818-31.
52. Yuan C, Zeng P, Cheng C, et al. Boosting the rate performance of Li-S batteries via highly dispersed cobalt nanoparticles embedded into nitrogen-doped hierarchical porous carbon. CCS Chem 2022;4:2829-41.
53. Zhao Y, Pei Z, Lu XF, Luan D, Wang X, Lou XW. Rationally designed nitrogen-doped carbon macroporous fibers with loading of single cobalt sites for efficient aqueous Zn-CO2 batteries. Chem Catal 2022;2:1480-93.
54. Su Y, Hao J, Liu X, Yang Y. Progress of atomic layer deposition and molecular layer deposition in the development of all-solid-state lithium batteries. Batteries Supercaps 2023;6:e202200359.
55. Wen Z, Zhang S, Liu Z, et al. Size-engineered noble metal nanoclusters synthesized by impregnation for size-dependent catalysis. Sci China Mater 2023;66:1417-26.
56. Jiang L, Luo X, Wang D. A review on system and materials for aqueous flexible metal-air batteries. Carbon Energy 2023;5:e284.
57. Zhao Y, Shi Z, Zhu Y, et al. Mechanism for one-pot synthesis of 0D-2D carbon materials in the bubbles inside molten salts. Adv Funct Mater 2022;32:2202381.
58. Hou S, Zhang A, Zhou Q, et al. Designing heterostructured FeP-CoP for oxygen evolution reaction: interface engineering to enhance electrocatalytic performance. Nano Res 2023;16:6601-7.
59. Xia H, Zan L, Qu G, et al. Evolution of a solid electrolyte interphase enabled by FeN
60. Xia H, Yuan P, Zan L, et al. Probing the active sites of 2D nanosheets with Fe-N-C carbon shell encapsulated Fe
61. Zhao B, Huang X, Ding Y, Bi Y. Bias-free solar-driven syngas production: a Fe2O3 photoanode featuring single-atom cobalt integrated with a silver-palladium cathode. Angew Chem Int Ed 2023;62:e202213067.
62. Ma Z, Liu S, Tang N, et al. Coexistence of Fe nanoclusters boosting Fe single atoms to generate singlet oxygen for efficient aerobic oxidation of primary amines to imines. ACS Catal 2022;12:5595-604.
63. Xue D, Cheng J, Yuan P, et al. Boron-tethering and regulative electronic states around iridium species for hydrogen evolution. Adv Funct Mater 2022;32:2113191.
64. Yin Z, Yu J, Xie Z, et al. Hybrid catalyst coupling single-atom Ni and nanoscale cu for efficient CO2 electroreduction to ethylene. J Am Chem Soc 2022;144:20931-8.
65. Xiao F, Wang Y, Xu GL, et al. Fe-N-C boosts the stability of supported platinum nanoparticles for fuel cells. J Am Chem Soc 2022;144:20372-84.
66. Zhang X, Zhang M, Deng Y, et al. A stable low-temperature H2-production catalyst by crowding Pt on α-MoC. Nature 2021;589:396-401.
67. Qiao B, Wang A, Yang X, et al. Single-atom catalysis of CO oxidation using Pt1/FeO
68. Sun L, Reddu V, Wang X. Multi-atom cluster catalysts for efficient electrocatalysis. Chem Soc Rev 2022;51:8923-56.
69. Zhao YX, Wen JH, Li P, et al. A “pre-division metal clusters” strategy to mediate efficient dual-active sites ORR catalyst for ultralong rechargeable Zn-air battery. Angew Chem Int Ed 2023;62:e202216950.
70. Han J, Liu R, Lin Z, Zi W. Stereodivergent construction of Csp3-Csp3 bonds bearing vicinal stereocenters by synergistic palladium and phase-transfer catalysis. Angew Chem Int Ed 2023;62:e202215714.
71. Ye Y, Cao J, Oblinsky DG, et al. Using enzymes to tame nitrogen-centred radicals for enantioselective hydroamination. Nat Chem 2023;15:206-12.
72. Shi J. On the synergetic catalytic effect in heterogeneous nanocomposite catalysts. Chem Rev 2013;113:2139-81.
73. Liu H, Lang X, Zhu C, et al. Efficient electrochemical nitrate reduction to ammonia with copper-supported rhodium cluster and single-atom catalysts. Angew Chem Int Ed 2022;61:e202202556.
74. Ding S, He L, Fang L, et al. Carbon-nanotube-bridging strategy for integrating single Fe atoms and NiCo nanoparticles in a bifunctional oxygen electrocatalyst toward high-efficiency and long-life rechargeable zinc-air batteries. Adv Energy Mater 2022;12:2202984.
75. Guo W, Gao X, Zhu M, et al. A closely packed Pt1.5Ni1-
76. Zhang L, Jang H, Wang Y, et al. Exploring the dominant role of atomic- and nano-ruthenium as active sites for hydrogen evolution reaction in both acidic and alkaline media. Adv Sci 2021;8:e2004516.
77. Su P, Pei W, Wang X, et al. Exceptional electrochemical HER performance with enhanced electron transfer between Ru nanoparticles and single atoms dispersed on a carbon substrate. Angew Chem Int Ed 2021;60:16044-50.
78. Yang X, Wang Y, Wang X, et al. CO-tolerant PEMFC anodes enabled by synergistic catalysis between iridium single-atom sites and nanoparticles. Angew Chem Int Ed 2021;60:26177-83.
79. Guo S, Su J, Luo H, et al. Boosting photocatalytic hydrogen evolution reaction by the improved mass flow and energy flow process based on ultrasound waves. ACS Catal 2023;13:296-307.
80. Wei P, Chen Y, Zhou T, et al. Manipulation of charge-transfer kinetics via Ti3C2
81. Zhu J, Cao J, Cai G, et al. Non-trivial contribution of carbon hybridization in carbon-based substrates to electrocatalytic activities in Li-S batteries. Angew Chem Int Ed 2023;62:e202214351.
82. Wei H, Liu H, Yu L, et al. Alloying Pd with Cu boosts hydrogen production via room-temperature electrochemical water-gas shift reaction. Nano Energy 2022;102:107704.
83. Zhang L, Ren X, Zhao X, et al. Synergetic charge transfer and spin selection in CO oxidation at neighboring magnetic single-atom catalyst sites. Nano Lett 2022;22:3744-50.
84. Huang H, Yu D, Hu F, et al. Clusters induced electron redistribution to tune oxygen reduction activity of transition metal single-atom for metal-air batteries. Angew Chem Int Ed 2022;61:e202116068.
85. Chen M, Kong F, Yao H, et al. Dual metal-organic frameworks-derived Fe-atomic sites bounded to fine Fe/Fe
86. Yao H, Wang X, Li K, et al. Strong electronic coupling between ruthenium single atoms and ultrafine nanoclusters enables economical and effective hydrogen production. Appl Catal B Environ 2022;312:121378.
87. Bae S, Mahmood J, Jeon I, Baek J. Recent advances in ruthenium-based electrocatalysts for the hydrogen evolution reaction. Nanoscale Horiz 2020;5:43-56.
88. Hu Q, Li G, Huang X, et al. Electronic structure engineering of single atomic Ru by Ru nanoparticles to enable enhanced activity for alkaline water reduction. J Mater Chem A 2019;7:19531-8.
89. Yu Y, Xue D, Xia H, et al. Electron spin modulation engineering in oxygen-involved electrocatalysis. J Phys Condens Matter 2022;34:364002.
90. Tian Y, Cao H, Yang H, et al. Electron spin catalysis with graphene belts. Angew Chem Int Ed 2023;62:e202215295.
91. Chen W, Xia H, Guo K, et al. Atomically dispersed Fe-N4 sites and Fe3C particles catalyzing polysulfides conversion in Li-S batteries. Chem Res Chin Univ 2022;38:1232-8.
92. Qu G, Guo K, Dong J, et al. Tuning Fe-spin state of FeN4 structure by axial bonds as efficient catalyst in Li-S batteries. Energy Stor Mater 2023;55:490-7.
93. Yang G, Zhu J, Yuan P, et al. Regulating Fe-spin state by atomically dispersed Mn-N in Fe-N-C catalysts with high oxygen reduction activity. Nat Commun 2021;12:1734.
94. Wei X, Song S, Cai W, et al. Tuning the spin state of Fe single atoms by Pd nanoclusters enables robust oxygen reduction with dissociative pathway. Chem 2023;9:181-97.
95. Liu Y, Liu X, Lv Z, et al. Tuning the spin state of the iron center by bridge-bonded Fe-O-Ti ligands for enhanced oxygen reduction. Angew Chem Int Ed 2022;61:e202117617.
96. Yang J, Li WH, Tan S, et al. The electronic metal-support interaction directing the design of single atomic site catalysts: achieving high efficiency towards hydrogen evolution. Angew Chem Int Ed 2021;60:19085-91.
97. Yang J, Li W, Wang D, Li Y. Electronic metal-support interaction of single-atom catalysts and applications in electrocatalysis. Adv Mater 2020;32:e2003300.
98. Hu P, Huang Z, Amghouz Z, et al. Electronic metal-support interactions in single-atom catalysts. Angew Chem Int Ed 2014;53:3418-21.
99. Liu P, Chen B, Liang C, et al. Tip-enhanced electric field: a new mechanism promoting mass transfer in oxygen evolution reactions. Adv Mater 2021;33:e2007377.
100. Khan MU, Wang L, Liu Z, et al. Pt3Co octapods as superior catalysts of CO2 hydrogenation. Angew Chem Int Ed 2016;55:9548-52.
101. Yu J, Yin J, Li R, Ma Y, Fan Z. Interfacial electric field effect on electrochemical carbon dioxide reduction reaction. Chem Catal 2022;2:2229-52.
102. Liu W, Li X, Wang Y, et al. Multi-branched AgAuPt nanoparticles for efficient electrocatalytic hydrogen evolution: synergism of tip-enhanced electric field effect and local electric field effect. J Energy Chem 2023;81:339-48.
103. Wang L, Wang L, Zhang L, Liu H, Yang J. Perspective of
104. Yan H, Xiang H, Liu J, et al. The factors dictating properties of atomically precise metal nanocluster electrocatalysts. Small 2022;18:e2200812.
105. Yang J, Li WH, Xu K, Tan S, Wang D, Li Y. Regulating the tip effect on single-atom and cluster catalysts: forming reversible oxygen species with high efficiency in chlorine evolution reaction. Angew Chem Int Ed 2022;61:e202200366.
106. Zhou W, Jiang Z, Chen M, et al. Directly anchoring non-noble metal single atoms on 1T-TMDs with tip structure for efficient hydrogen evolution. Chem Eng J 2022;428:131210.
107. Xia H, Zan L, Yuan P, et al. Evolution of stabilized 1T-MoS2 by atomic-interface engineering of 2H-MoS2/Fe-N
108. Ma Q, Jin H, Zhu J, et al. Stabilizing Fe-N-C catalysts as model for oxygen reduction reaction. Adv Sci 2021;8:e2102209.
110. Ao X, Zhang W, Li Z, et al. Markedly enhanced oxygen reduction activity of single-atom Fe catalysts via integration with Fe nanoclusters. ACS Nano 2019;13:11853-62.
111. Wan X, Liu Q, Liu J, et al. Iron atom-cluster interactions increase activity and improve durability in Fe-N-C fuel cells. Nat Commun 2022;13:2963.
112. Xie S, Liu L, Lu Y, et al. Pt atomic single-layer catalyst embedded in defect-enriched ceria for efficient CO oxidation. J Am Chem Soc 2022;144:21255-66.
113. Chen L, Zhang X, Chen A, Yao S, Hu X, Zhou Z. Targeted design of advanced electrocatalysts by machine learning. Chin J Catal 2022;43:11-32.
Cite This Article

How to Cite
Wei, Y.; Xia H.; Yan W.; Zhang J. N. Recent processing of interaction mechanisms of single metallic atom/clusters in energy electrocatalysis. Energy Mater. 2023, 3, 300033. http://dx.doi.org/10.20517/energymater.2023.13
Download Citation
Export Citation File:
Type of Import
Tips on Downloading Citation
Citation Manager File Format
Type of Import
Direct Import: When the Direct Import option is selected (the default state), a dialogue box will give you the option to Save or Open the downloaded citation data. Choosing Open will either launch your citation manager or give you a choice of applications with which to use the metadata. The Save option saves the file locally for later use.
Indirect Import: When the Indirect Import option is selected, the metadata is displayed and may be copied and pasted as needed.
About This Article
Copyright
Data & Comments
Data
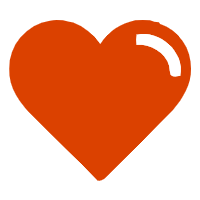
Comments
Comments must be written in English. Spam, offensive content, impersonation, and private information will not be permitted. If any comment is reported and identified as inappropriate content by OAE staff, the comment will be removed without notice. If you have any queries or need any help, please contact us at support@oaepublish.com.