Hydrogen and organic synthesis from wastewater with nano photocatalysts: a synergy
Abstract
Nano-photocatalysts exhibit significant potential for diverse applications, including the degradation of organic pollutants, hydrogen generation through water splitting, organic synthesis, and photoelectrochemical conversion. Recently, there has been growing interest in the efficient utilization of wastewater and liquid organic waste as resources for organic synthesis and hydrogen production. This approach offers a promising solution to both energy and environmental challenges by enabling hydrogen and organic synthesis from wastewater using nanophotocatalysts. Dual-functional nanophotocatalysts demonstrated significant potential for efficient catalysis processes. This review provides a comprehensive analysis of various nano-photocatalysts, their synthesis processes, and the underlying photocatalytic mechanisms that drive the synergistic effects, leading to enhanced efficiency. Furthermore, the resulting photocatalytic products and their implications are discussed in detail. Key challenges associated with this emerging technology are identified, along with future research directions to advance its development. By highlighting recent advancements in the use of nano-photocatalysts for hydrogen generation and organic compound synthesis from wastewater and liquid organic waste, this review serves as a valuable resource to guide ongoing and future research efforts in this field.
Keywords
INTRODUCTION
In recent decades, global awareness of the escalating energy demands and environmental challenges associated with fossil fuel consumption has increased significantly. Gong et al. reported that these growing concerns have fueled increased global awareness over the past few decades. Currently, the global energy supply primarily relies on fossil fuels, including coal, oil, and natural gas. However, these fossil fuel reserves are rapidly depleting, and global energy demand is projected to double by 2025 because of rapid industrialization and population growth[1,2]. Furthermore, George et al. reported that fossil fuel consumption generates toxic emissions, posing significant environmental threats. To address these challenges, significant advancements in materials science and engineering have been made to develop efficient energy-conversion technologies and environmental preservation strategies[3,4]. Bard et al. reported that among the various methods investigated, semiconductor-based photocatalysis has emerged as a promising technology with a significant interdisciplinary interest in environmentally friendly energy solutions. This technology has significant potential for harnessing clean and sustainable solar energy for a wide range of energy and environmental applications[5-15]. Chen et al. reported that the direct conversion of solar energy into energy fuels and chemical energy is a promising green and sustainable approach to addressing the impending energy and environmental crises[16-23]. Qi et al. reported that these processes, known as photocatalysis, utilize suitable semiconductors and solar light to drive a variety of catalytic reactions, including the selective synthesis of organic compounds from liquid organic wastewater[24-30] and water splitting to produce H2[31-42].
The groundbreaking 1972 paper by Fujishima and Honda marked a significant milestone in the field of photocatalytic water splitting using TiO2 electrodes under ultraviolet (UV) light[43]. Since then, numerous studies have reported significant progress in the fabrication of highly efficient semiconductor-based photocatalysts[44-51]. According to a literature review by Alam et al., numerous photocatalysts have been developed that are active under both visible and UV light, including Ag3PO4/Mn-ZnO[52], ZnO/MoO3[53], Al and Fe co-doped ZnO[54], Ag2O/Fe–TiO2[55], Fe2O3 doped TiO2[56], ZnO/CuO/MoO3[57], Bi2WO6 modified by N-doped g-C3N4[58], Bi2WO6 with Bi-metal and Bi2O3[59], BiVO4/TiS2[60], BiVO4[61], Ta2O5[62,63], TaON[64],
Figure 1. A number of publications related to (A) photocatalytic hydrogen production/evolution and (B) photocatalytic hydrogen production/evolution with synergistic organic synthesis from April 1, 2018, to May 19, 2024. Data were retrieved from ScienceDirect using relevant keywords.
Photocatalytic hydrogen generation coupled with synergistic organic synthesis from wastewater/liquid organic waste enables the control of reaction parameters and catalytic materials to meet specific requirements for organic synthesis or product preparation. Considering bi-functional synergy, this approach suggests a bi-directional selectivity between the photocatalyst and the waterborne contaminant. Enhanced hydrogen production coupled with simultaneous organic synthesis requires a suitable environment, the presence of a pollutant in water, and an effective catalyst. Essentially, the environmental medium, pollutants, and catalyst must be well-matched[24,77-79]. However, to the best of our knowledge, there is currently no concise summary of photocatalysis for H2 production and synergistic organic synthesis from wastewater. Therefore, providing a timely and concise overview of the latest advancements in dual-functional photocatalytic systems is crucial.
In conclusion, this mini-review provides an overview of recent research on photocatalytic hydrogen production and organic synthesis from wastewater or liquid organic waste. This study highlights recent advancements in nanophotocatalyst material composition, microstructures, synthesized compounds, pollutant properties, reaction products, and co-existing materials in systems. In addition, the necessary techniques from both the catalyst and pollutant perspectives are discussed. Finally, this review summarizes the key challenges and prospects for future research directions in this promising field.
FUNDAMENTAL PRINCIPLES OF PHOTOCATALYTIC MECHANISMS
Overall water splitting
Titanium dioxide (TiO2), the first photocatalyst reported by Fujishima and Honda in 1972, has been extensively studied for its ability to induce light-driven water splitting[43,78,80]. Since then, TiO2 and other semiconducting materials have been the focus of numerous photocatalysis studies[81,82]. Figure 2A illustrates the principles of overall water splitting (OWS) into H2 and O2 using a single semiconducting material in solution[83,84]. Firstly, according to the thermodynamics of this reaction, the conduction band (CB) minimum of the semiconductor must be more negatively potentialized than the H+/H2 energy level [-0.41 V vs. normal hydrogen electrode (NHE)], and the valence band (VB) maximum must be more positively potentialized than the O2/H2O energy level (0.82 V vs. NHE) for the two redox half-reactions to occur simultaneously[85]. Consequently, the semiconductor bandgap must be at least 1.23 eV for OWS. However, in practical applications, a bandgap larger than 1.7 eV is typically required because of the significant overpotential associated with the slowest kinetics of the water oxidation and proton reduction reactions. Secondly, the coupling of 2e- with 2H+ is involved in the H2 evolution reaction (HER), which occurs more efficiently when semiconductor photocatalysts are coupled with HER cocatalysts[86]. The main challenge lies in the release of diatomic O2, which requires the cleavage of four O–H bonds and subsequent production of O=O bonds. Typically, this process proceeds at reasonable rates only at high potentials[87]. Furthermore, O2 is a significant electron acceptor that opposes the HER half-reaction and produces reactive oxygen species (ROS), which can damage the system when water oxidation or O2 reduction occurs excessively[88]. Consequently, the conditions for initiating OWS are strict; failure to meet even one of the requirements prevents the reaction from occurring.
Figure 2. Diagram illustrating (A) H2 production via water splitting reaction and (B) the simultaneous coupling of H2 generation with selective organic synthesis over semiconductor-based photocatalysts[24]. Copyright 2021, American Chemical Society.
Photocatalytic hydrogen production with synergistic organic synthesis
Photocatalytic hydrogen production with synergistic organic synthesis from wastewater or separate photocatalytic organic synthesis requires several essential steps. These processes include (i) light absorption, (ii) formation and separation of electron-hole pairs, and (iii) surface reactions such as redox processes[78,89-91]. As illustrated in Figure 2B, the band gap represents the region between the VB and CB, where the density of state (DoS) is zero. The bandgap energy of semiconductors is smaller than that of insulators and is well-matched with the energy of photons in the solar spectrum. This enables photons to stimulate electron transitions within the semiconductor, generating electron-hole pairs that further drive photocatalytic reactions[92]. In addition, as illustrated in Figure 2B, the appropriate use of organic substrates, such as alcohols, hydrocarbons, ethers, and amines, as proton sources are essential for the synergistic reactions of hydrogen evolution and organic synthesis. Specifically, the X–H bonds (where X = C, N, O, and S) of organic reactants are activated and cleaved by holes with oxidizing ability. This process generates an intermediate radical and a proton. The protons subsequently combine with photoexcited electrons in a reduction reaction to produce hydrogen. Intermediate radicals tend to recombine or reform C–C or C–X bonds, resulting in the formation of chemicals or other value-added oxidation products (Ox)[93-95]. Figure 3 illustrates that visible light constitutes approximately 46% of sunlight, which is significantly greater than the 5% UV light. Visible light is also more readily absorbed and utilized than infrared light, which comprises approximately 49% of sunlight. Therefore, photocatalytic materials must improve their ability to absorb both visible and near-infrared light[96-98]. For effective photocatalytic processes, the photogenerated carriers must possess a sufficiently long lifetime to migrate to the catalyst surface, which is commonly referred to as the reaction active site. A key aspect of the photocatalytic implementation of this process is minimizing the recombination rate of the photogenerated electrons and holes. Following the migration of photogenerated carriers to the reaction sites, surface redox reactions occur. Here, photogenerated electrons reduce the number of active protons to produce hydrogen gas, while photogenerated holes or other strongly oxidative species gradually decompose synthetic materials into various intermediates or organic compounds, along with water. In these reactions, the photocatalytic mechanism, nature of the synthetic materials, and any co-existing substances all play critical roles in determining the overall efficiency and outcome of the process[31,78].
Figure 3. Standard solar energy distribution and solar radiation spectrum[98]. Copyright 2021, Wiley-VCH GmbH.
Concurrent organic synthesis and hydrogen production
Any photocatalyst with an appropriate band structure can achieve the desired hydrogen evolution and organic oxidation. For dual-functionality, the photocatalyst must possess a sufficiently positive VB to drive the target organic oxidation and a CB that is more negative than the hydrogen production potential (-0.41 V vs. NHE; pH = neutral). As illustrated in Figure 4, materials such as TiO2, ZnO, Cu2O, CdS, and g-C3N4 possess sufficiently positive VB potential to oxidize most typical organic compounds, including alcohols, thiols, hydrocarbons, amines, and heterocycles[29,30,99]. The oxidation products of organic compounds are also determined by the VB of semiconductors. A semiconductor with an excessively positive VB, often indicating an excessively strong oxidation capacity, is not suitable for target organic synthesis. For example, TiO2 and ZnO, which possess wide band gaps, are commonly used to oxidize alcohols into aldehydes, ketones, or even mineralized compounds such as CO, CO2, and H2O[100,101]. In contrast, alcohol oxidation reactions often yield products involving carbon-carbon (C-C) linkages when visible light-active photocatalysts with narrow band gaps, such as CdS, are used[102,103]. Techniques such as doping, defect engineering, and crystal facet modifications enable the tuning of semiconductor band structures to optimize their photocatalytic performance[99,104]. This indicates that the band structures of photocatalysts can be tailored to promote specific organic oxidation reactions. However, a detailed discussion of these techniques is not explored further here because they have been comprehensively addressed in previous studies focused on hydrogen evolution and other single-function processes[92,105]. The energy levels of distinct semiconductor photocatalysts and the redox potential of different reactions in water-based solutions are shown in Figure 4[106].
Figure 4. Energy levels of distinct semiconductor photocatalysts and the redox potential of different reactions in water-based solutions[106]. Copyright 2022, Elsevier B.V. All rights reserved.
The primary objective of this review is to provide a comprehensive overview of recent advancements in the synergistic integration of high-value organic molecule synthesis and photocatalytic hydrogen production. To enhance this synergy, each subsection is organized based on specific photocatalysts, introducing several novel photocatalysts and innovative promotional strategies. The subsections address the characteristics of the photocatalysts, methods for catalyst modification, and factors influencing the activity and selectivity of these photocatalytic systems.
Experimental photoreaction systems
The experimental photoreaction system for hydrogen production and organic synthesis was conducted under an argon atmosphere using a xenon lamp (PLS-SXE300) as the irradiation source at an intensity of 200 mW/cm. The reaction mixture, comprising 50 mL of aqueous solution, 5 mg of photocatalyst, and
Difficulties and challenges in hydrogen production with synergistic organic synthesis
Although extensive research has been conducted on photocatalytic organic synthesis and hydrogen production from wastewater, synergistic catalysis remains relatively understudied. The primary challenge in achieving dual-function photocatalysis systems is that conventional photocatalytic hydrogen production typically occurs in an anaerobic environment. Under such conditions, oxygen captures the electrons generated by photosynthesis, which are essential for proton reduction, thereby influencing the subsequent hydrogen production reaction.
Photocatalytic organic synthesis from wastewater can be conducted in both aerobic and anaerobic environments. However, aerobic conditions may lead to unwanted side reactions, oxidation, or reduction of specific organic molecules when they interact with oxygen. To protect these sensitive molecules and enhance the selectivity of the desired transformations, anaerobic environments are often preferred. Anaerobic conditions can be achieved through oxygen-scavenging techniques or by purging reaction vessels with inert gases such as argon or nitrogen[107]. Conversely, many organic synthesis reactions can proceed in the presence of oxygen in an anaerobic environment. Many synthetic processes in laboratories and industries operate under aerobic conditions. In several transformations, oxygen serves as an oxidant, co-reactant, or oxygen atom source. For instance, molecular oxygen is typically required for oxidation reactions, epoxidation, hydroxylation, and oxidative coupling reactions. The synthesis of oxygen-containing functional groups, such as alcohols, ketones, and carboxylic acids, is often favored under aerobic conditions[108].
In summary, organic synthesis can be performed in either anaerobic or aerobic environments, depending on the reaction type, substrate and intermediate reactivity, and desired products. To achieve efficient and selective outcomes, researchers and synthetic chemists carefully evaluate these factors when designing synthetic pathways and selecting appropriate reaction conditions. Integrating the two procedures requires not only an anaerobic environment, appropriate reaction conditions, and suitable reaction vessels but also ensures that the efficiency of organic synthesis and hydrogen production is maintained without mutual interference. However, the optimal conditions for such integrated systems require further investigation[109].
Dual-function photocatalysis encounters challenges similar to those of traditional mono-functional photocatalysis, although under more demanding conditions. Materials with large bandgaps are more stable but exhibit limited visible-light absorption. Conversely, narrow-bandgap materials, although effective light absorbers, often suffer from low energy consumption and instability, thereby compromising the overall photocatalytic performance. This significantly reduces the efficiency of solar energy conversion[24,78,110]. As shown in Figure 5[111], simultaneous oxygen-hydrogen production and organic synthesis can be achieved by carefully tuning various parameters, including catalyst type, dosage, and composition, as well as the microstructure, pollutant type and concentration, system interactions, pH, reaction temperature, and light intensity. The effects of the composites, additional catalyst components, and microstructures are particularly significant at the catalyst level. This section explores these aspects to reveal the underlying mechanisms that enable and enhance these processes.
Figure 5. Effective aspects of synergistic photocatalytic reactions[111]. Copyright 2023, Elsevier B.V. All rights reserved.
SYNTHESIS METHODS FOR NANO PHOTOCATALYSTS
The synthesis of nanophotocatalysts is crucial for modifying their properties and enhancing their catalytic performance. This section reviews both conventional and advanced synthesis methods, including sol-gel, solvothermal/hydrothermal, and precursor techniques, which are widely employed to control particle morphology, composition, and functionality. These approaches are fundamental for optimizing the photocatalytic properties of materials.
Although physical synthesis methods are cost-effective, versatile, and allow for large-scale nanoparticle production, they often lack precise control over morphology and can introduce contaminants, which can adversely affect photocatalytic efficiency[112]. Chemical methods such as sol-gel and hydrothermal synthesis offer superior control over the size, shape, and surface properties of nano-photocatalysts, significantly enhancing their catalytic activity. These methods also enable synthesis in both aqueous and non-aqueous media, broadening their applications in biomedical and environmental fields[113,114]. However, challenges such as precursor toxicity and agglomeration can impact the material’s properties and performance[115]. The following chemical and physical methods are used for particle synthesis, as illustrated.
Sol-gel method
Sol-gel methods are synthetic techniques used to produce nanophotocatalysts. This chemical technique involves a combination of chemical and physical processes, including drying, calcination, dehydration, hydrolysis, and gelation. The term “sol-gel” refers to the transition of the solution into a “gel” during synthesis, characterized by an increase in viscosity, which is a distinctive feature of the method. Subsequent treatments facilitate the conversion of the gel into solid oxides[116].
Metal alkoxides and alkoxysilanes are commonly used as precursors in this method. These colloids appear bulky when mixed with liquid, whereas the particles appear clear. The resulting gel exists in a continuous liquid phase. Compared to other techniques, sol-gel methods offer advantages such as cost-effectiveness, better product size, controlled morphology, low processing temperatures, high purity, and improved homogeneity. Both crystalline and noncrystalline materials can be synthesized using sol-gel techniques[117]. Figure 6 provides a visual representation of the proposed method[118].
Figure 6. Schematic illustration of the sol-gel method[118].
Hydrothermal method
Among the various synthesis methods, the hydrothermal approach has been widely used for the production of nanophotocatalysts. The method is based on the solubility of many oxides in alkali-metal solutions. In this technique, water is used as a solvent due to its ability to dissolve minerals under high-pressure conditions, facilitating the formation of single crystals. Although effective, this synthesis method is time-consuming. An autoclave is used for crystal growth, where a nutrient is dissolved in water to facilitate the process. A schematic representation of the hydrothermal method used to fabricate metal oxide nanomaterials is shown in Figure 7[119].
Figure 7. Hydrothermal methods for the fabrication of metal oxide nanomaterial[119]. Copyright 2021 Published by Elsevier B.V.
This method is also used for material recrystallization. A temperature gradient is maintained across the growth chamber, with the cooler end promoting crystal growth and the hotter end dissolving the nutrient supply. Hydrothermal methods also enable the monitoring of grain size and morphology. Their additional advantages include the ability to form crystalline phases and control the composition to grow high-quality crystals. However, autoclaves are expensive, and real-time crystal growth monitoring is not feasible[120].
Solvothermal method
Solvothermal synthesis is a widely used method for producing nanophotocatalysts and other chemical compounds. This process is similar to hydrothermal synthesis, but the primary difference lies in the type of precursor used. While the hydrothermal method uses an aqueous solution (water), it uses a non-aqueous solution, such as alcohol or amine, as the precursor. The reaction proceeds within this solvent at temperatures exceeding its boiling point, leading to the formation of single crystals under high temperatures in a non-aqueous solution within the autoclave. This technique enables the formation of particles with precise and controlled shapes, improved morphologies, well-defined crystallinities, and phase distributions. The major advantage of solvothermal synthesis is its ability to combine the benefits of hydrothermal and sol-gel methods[121,122]. The solvothermal method is analogous to the hydrothermal approach; thus, the same schematic representation shown in Figure 7 can be applied.
Ceramic method
The ceramic method is widely used to synthesize mixed metal oxides, nano-photocatalysts, and various metals, along with phosphides, metal sulfates, and nitrides of different metals. It involves high-temperature processing, often achieved through resistance heating, which can influence the size, shape, and crystallographic properties of the resulting product. Powders of the desired material’s oxides or other compounds are ground at these elevated temperatures, typically after pelletization. A diagrammatic representation of the ceramic synthesis process is shown in Figure 8[123].
Figure 8. Flowchart of the ceramic synthesis process[123].
However, this method has several disadvantages. It requires extremely high temperatures and lacks a monitoring procedure to determine when the reaction is complete, often relying on trial and error. Achieving a homogenous product composition is challenging, and after the reaction is complete, unreacted materials often remain as impurities and byproducts that cannot be separated from the desired product, compromising its purity and phase identification[124,125].
Co-precipitation method
The co-precipitation method is a widely adopted synthesis technique because of its simplicity, cost-effectiveness, and versatility. This time-consuming method involves the formation of “mixed precipitates”, consisting of two or more insoluble compounds that can be easily removed from the solution. Different classes of nanophotocatalysts have been synthesized through co-precipitation methods, including[126]:
· Mixed metal oxide nanoparticles such as ZnO, NiO, and CdO.
· Doped metal oxides.
· Ternary ferrites with the structure M-Fe2O4, where M represents a divalent metal ion.
· Mixed ferrites with two metals, having the composition A1-xBxFe2O4.
Divalent metal cations, such as nitrates, sulfates, and chlorides, are mostly inorganic salts that are readily soluble in water. Precipitating agents, usually acidic or basic compounds such as NaOH, NH3, citric acid, and polyethylene glycol (PEG), are used to extract the byproduct from the solution and form the desired nanophotocatalysts precipitates. The precipitates are subsequently calcined in a muffle furnace to form spinel nanocomposites. During synthesis, the pH is maintained between 9 and 11 to facilitate the formation of high-quality crystals. Figure 9 shows a schematic representation of this process[127].
Figure 9. Schematic diagram of the co-precipitation technique[127].
The crystal growth of these particles is influenced by factors such as temperature, pH of the material and synthesis solution, composition, and salt concentration. After precipitation, the solid was allowed to settle and washed repeatedly with ethanol and distilled water. This washing process must be performed carefully to prevent particle agglomeration, which can adversely affect the morphology and structural properties of the nanophotocatalysts[128]. This can be minimized by maintaining a neat and clean working environment. After washing, the solid material is dried at the melting temperature for several hours and then finely ground to form a powder. The powder is then calcined at temperatures ranging from 550 to 800 °C, depending on the material. If necessary, a sintering process can be conducted, depending on the nature of the prepared material. The calcination temperature and duration are crucial factors affecting the particle size and surface morphology of the final product.
The co-precipitation method offers several advantages, including[129]:
· Lower temperature requirements than those of other techniques
· Cost-effectiveness
· Formation of fine, uniform particles with reduced agglomeration
· Homogenous and single-phase crystal formation
However, the chemical co-precipitation method is limited when dealing with materials exhibiting dissimilar solubility in water, including:
· Commonly occurring supersaturated solutions
· Different precipitation rates of reactants
Many researchers have adopted the co-precipitation method to synthesize nanoparticles of various material classes, making it one of the most common techniques for preparing nanophotocatalysts.
Biosynthesis method
A biosynthetic method is a biological approach used to synthesize materials from various organisms, including algae, fungi, bacteria, and plants, for applications in medicine.
Recent advancements have led to the development of green synthesis methods for producing nanoparticles with the desired particle size and morphology. These methods employ stabilizing, reducing, and capping agents derived from biological sources such as plants, bacteria, fungi, and algae. By using these natural agents, it is possible to synthesize materials without relying on expensive, toxic, or harsh chemical precursors, making this approach environmentally friendly and sustainable. This approach is a simple and cost-effective synthesis route. Figure 10 shows a schematic representation of the biosynthesis of nanoparticles[130]. Currently, various types of particles, including sulfides, metals, metallic oxides, and ferrite, are synthesized using biosynthesis method. The samples prepared through biosynthesis have diverse applications, including DNA analysis, magnetic resonance imaging (MRI), biosensors, cancer treatment, gene therapy, material separation science, and drug delivery[131].
Figure 10. Graphic illustration of the plant-mediated biosynthesis of nanoparticles[130].
Spray pyrolysis method
In spray pyrolysis methods, micro-sized liquid droplets of a precursor material mixture are converted into solid particles through heat treatment. The process typically involves the following steps, as illustrated in Figure 11[132]:
· Generation of micro-sized droplets of precursor materials
· Solvent evaporation
· Solute condensation
· Reaction and decomposition of solutes
· Sintering of solid particles
Figure 11. Representation of spray pyrolysis procedure and its apparatus[132].
Precursor method
In the precursor method, mixtures of metals and easily decomposable metal compounds are prepared. Upon heating, these metals form the desired oxides. The method allows the production of high-purity oxide materials with fine particles and a homogeneous composition and facilitates the preparation of nanophotocatalysts from water-soluble salt solutions of the corresponding materials. It is employed to produce single-phase nanophotocatalyst materials with large surface areas, high purity, low porosity, and molecular-level homogeneity, as shown in Figure 12[133].
Solid-state reaction method
The solid-state reaction (SSR) technique is widely used to synthesize polycrystalline bulk materials, particularly manganites and ferrites. This method uses a range of starting materials, including sulfates, nitrates, oxides, and carbonates. The solid precursors are heated at elevated temperatures to facilitate the desired reaction at an appropriate rate, as solids typically do not react at ambient conditions. The high-temperature environment minimizes concerns of agglomeration or structural alterations during synthesis[134].
The SSR method produces a stable product through chemical reactions between solid precursors in the absence of a solvent at high temperatures. A primary advantage of this route is that the obtained product is in solid form and exhibits uncontaminated structural properties that are influenced by the sintering temperature. Additionally, this method is environmentally friendly and nontoxic, as it does not generate unwanted waste or byproducts[135].
A significant limitation of the SSR method is its inability to produce large single crystals. Additionally, it is energy-intensive and time-consuming, and it has low efficiency. The requirement for high temperatures and specialized furnaces further adds to the drawback of this method, as illustrated in Figure 13[135,136].
Figure 13. Graphic illustration presenting the diverse steps of the SSR method[136]. SSR: Solid-state reaction.
NANO PHOTOCATALYSTS FOR HYDROGEN PRODUCTION AND ORGANIC SYNTHESIS
Hydrogen is emerging as a promising clean and versatile energy source, attracting significant attention for its potential to replace fossil fuels in diverse applications such as fuel cells, energy storage, and transportation systems. Various types of nano-photocatalysts, including metal oxides, metal sulfides, carbon-based materials, and hybrid structures, have been effectively employed in hydrogen production and organic synthesis from wastewater and alcohol. The proposed approach offers a promising solution for treating wastewater while simultaneously generating sustainable energy. The use of nanostructured photocatalysts enhances the efficiency and selectivity of water-splitting processes. Recent advancements in nanophotocatalyst research and development have led to significant performance improvements, including reduced charge recombination losses, effective charge separation, and increased light absorption[137,138]. To enhance the catalytic activity of photocatalysts used in hydrogen evolution processes, various strategies have been employed, such as surface modification, heterostructuring, and doping[139]. These approaches aim to modify the electronic band structure and surface properties of catalysts. In addition to hydrogen production, nanophotocatalysts have attracted significant attention for their applications in organic synthesis. They facilitate the production of fine chemicals, pharmaceuticals, and biofuels from renewable energy sources. Photocatalytic organic transformations, such as oxidative coupling reactions, C-H functionalization, and C–C bond formation, enable the synthesis of complex organic molecules in an environmentally friendly and sustainable manner[102,140]. Nanostructured photocatalysts exhibit remarkable catalytic performance with excellent selectivity and moderate reaction conditions in various organic synthesis reactions. The precise control over reaction pathways and product distribution enabled by the regulated photocatalytic activation of organic materials facilitates the formation of valuable molecules with minimal environmental impact.
However, a comprehensive review of the literature on this topic is lacking. This overview summarizes the current state of the art based on a few recent publications. In recent years, there has been a significant surge in interest in photocatalytic reactions for hydrogen production and organic synthesis. This is largely due to the unsustainable nature of traditional industrial methods for the synthesis of hydrogen and organic compounds, which have significant resource and environmental implications, as detailed in the literature and Table 1. Shang et al. demonstrated the potential of a 1D/2D CdS/BiVO4 binary nanocomposite synthesized through electrostatic self-assembly for organic synthesis and hydrogen production. This composite effectively catalyzed C-C coupling with benzaldehyde as the substrate[141]. Similarly, Luo et al. investigated a NiS/CdS nanorod composite for simultaneous H2 production and benzaldehyde oxidation. The incorporation of NiS into CdS nanorods significantly enhanced electron-hole pair separation and overall stability, leading to improved photocatalytic performance[142].
Summary of some reported research papers on photocatalytic H2 production and organic synthesis
Photocatalyst | Synthesis method | Morphology | Light source | Hydrogen production | Synthesized organic products | Ref. |
MgO | Colloidal chemical | Porous type | Solar | 320 μmol·g-1·h-1 | Formaldehyde | [144] |
Pt/TiO2 | Sol-gel | Nano-film | UV-Vis | 4.675 mmol·h-1 | Formic acid | [145] |
N-doped TiO2 and Au-doped TiO2 | Deposition precipitation | Nanoparticles | Visible | 200 nmol·g-1·h-1 | Methyl formate | [146] |
2D SnS/g-C3N4 | Hydrothermal | Nanosheets | Visible | 1.27 mmol·g-1·h-1 | Liquid chemicals | [147] |
defective TiO2 | Facile NaBH4 reduction | Bulk crystal structure | UV and Visible | 0.08 mmol·h-1 | Liquid acetaldehyde | [149] |
Ni-MMT/TiO2 | Sol-gel | MMT layers on the spherical shape of TiO2 | UV | 3,470 μmol·g-1·h-1 | Hydrocarbons | [150] |
Au@TiO2 (Ti3+) | Sol-gel | Nanoparticles and nanorods | Visible | ~7,093 μmol·g-1·h-1 | CH4 | [151] |
CdS/MOF | Hydrothermal | Block shaped | Visible | 2,334 μmol·g-1·h-1 | Benzaldehyde | [152] |
Co/CdS | Photo-deposition | Nanorods | Visible | 442 μmol·g-1·h-1 | Benzaldehyde | [153] |
AgNPs/CdS | Microwave-assisted | Rod-like linear structure | Visible | 1,720.9 μmol g-1 h−1 | Acetaldehyde | [155] |
Pd/HNb3O8-H2 | Impregnation and thermal treatment | porous structure | Visible | 3.17 mmol·g-1·h-1 | Benzaldehyde | [104] |
MOF-derived Co9S8/CdS | Hydrothermal | Pore type structure | Visible | 61,924 μmol·g-1 in 6 h | Benzaldehyde | [157] |
P and S-doped g-C3N4 | Co-precipitation | Mesoporous spheres | Visible | 3.76 μmol·g-1·h-1 | Benzaldehyde | [158] |
PdSA+C/TiO2-VO | Pyrolysis | Rectangular blocks and clusters | UV-visible | 5,800 μmol·g-1·h-1 | N-benzylidenebenzylamine | [99] |
MoS2/ZnIn2S4 | Facile photochemical reduction | NFs | Visible | 3.88 mmol·g-1·h-1 | Benzaldehyde | [95] |
Pang et al. successfully synthesized single-layer MoS2, WS2, and ReS2 nanosheets using a one-pot colloidal wet-chemical method. Different molecular surfactants were used to facilitate the growth of single-layer 2D morphologies. These nanosheets were subsequently utilized for H2 fuel production through the decomposition of methanol into formaldehyde, derived from hydrogen-stored liquid waste containing methanol. MoS2 exhibited the optimum photocatalytic efficiency, achieving approximately 617 μmol·g-1·h-1 under normal pressure and room temperature conditions. This approach offers a promising pathway for generating solar H2 fuel by storing methanol, which can be easily produced through chemical re-bonding facilitated by 2D single-layer photocatalysts[143].
Liu et al. developed a novel facile colloidal chemical method to synthesize MgO for H2 production from liquid methanol at room temperature. The formation of COx-free H2 is also attributed to the photodecomposition of methanol into formaldehyde, facilitated by the surface electronic states of distinct monodispersed, porous MgO nanocrystals. The photocatalytic H2 production performance of the MgO photocatalyst from methanol improved over time, reaching 320 μmol·g-1·h-1 after 2 days. The energy band gap and charge carrier recombination decreased as the size of the MgO nanoparticles increased to
Cui et al. fabricated a Pt/TiO2 nano-film using the sol-gel method. In a continuous-flow reactor, the Pt/TiO2 nano-film demonstrated photocatalytic decomposition of methanol aqueous solution into H2 and formic acid. The study investigated the effects of several variables, including the methanol concentration, reaction duration, and space velocity, on the photocatalytic process. Notably, the photocatalytic activity of the Pt/TiO2 nano-film exceeds that of pristine TiO2[145].
Gazsi et al. synthesized N- and Au-doped TiO2 using the deposition-precipitation method. In all cases, the addition of H2O or O2 to the alcohol reduced CO production; in the case of methanol, CO was entirely removed. The photocatalytic decomposition of methanol and ethanol into H2 and methyl formate was significantly enhanced by Au-doped TiO2 compared with N-doped TiO2 under visible light[146].
Uddin et al. synthesized 2D SnS/g-C3N4 heterojunction photocatalysts using a hydrothermal method. The photocatalysts enhanced H2 production and organic or liquid chemical synthesis from liquid organic waste, such as methanol, ethanol, and isopropanol, without the emission of COx. The process achieved approximately 95% recoverability, and the hydrogen generation efficiency reached 1.27 mmol·g-1·h-1. Experimental and product analyses revealed that process reduction occurs through a series of redox processes, including disproportionation and combination. Each alcohol demonstrated a higher hydrogen production efficiency than previous reports, along with excellent reversibility (> 95%) over extended periods of operation (up to 360 h). In this study, the use of 2D SnS/g-C3N4 heterostructures for phototransforming light alcohols exemplifies a selective reaction, as illustrated in Figure 14[147].
Figure 14. (A) Powder XRD and (B) HRTEM images of both g-C3N4 and SnS; (C) Selected area electron diffraction results of SnS and SnS/g-C3N4; (D) EDS elemental maps of SnS/g-C3N4 heterojunction; (E) HRTEM images of SnS/g-C3N4; (F) STEM with an EDS elemental map showing the co-existence of Sn, N, C, and S in the SnS/g-C3N4 heterostructure; (G) Photo-oxidation of isopropanol, ethanol, and methanol along with experimental output ratios[147]. Copyright 2021, Elsevier B.V. All rights reserved. XRD: X-ray diffraction; HRTEM: high-resolution transmission electron microscopy; EDS: energy dispersive spectroscopy; STEM: scanning transmission electron microscopy.
Xie et al. prepared MoS2 and CdS for the conversion of methanol into hydrogen and ethylene glycol (EG) through controlled C-C coupling, a highly desirable and promising goal. This work introduced a novel visible-light-driven catalytic C–H activation approach for EG synthesis that preserves the hydroxyl group within the same molecule while offering an alternative, non-petroleum-based method[84].
Tan et al. developed bifunctionally modified carbon nitride incorporating interior cyano and surface carboxyl groups through a two-step process. This modification resulted in a photocatalyst with 205 times more active sites and an enhanced photoexcited charge carrier separation efficiency compared with pristine carbon nitride. The modified photocatalyst exhibited superior hydrogen and formaldehyde production performance in methanol-containing wastewater under visible light illumination[148].
Zhang et al. developed a simple NaBH4 reduction method to fabricate defective TiO2 photocatalysts for the simultaneous production of H2 gas and organic compounds such as acetaldehyde from methanol, ethanol, and isopropanol-containing wastewater. The modified TiO2 exhibited enhanced light absorption and charge separation, leading to improved photocatalytic activity. This resulted in a hydrogen production rate of
Figure 15. Hydrogen purity achieved using pristine and defective TiO2 catalysts with various reactants[149]. Copyright 2023, Author(s). Published by Elsevier B.V.
Figure 16. (A) Schematic representation of a spiral photoreforming reaction with a reaction bottle, automated gas sampling system, gas pump, liquid pump, and UV fluorescent lamp[149]. Copyright 2023, Author(s). Published by Elsevier B.V; (B) Diagrammatic representation of the experimental setup for a gas-phase photoreactor’s photocatalytic H2 generation from an ethanol-water mixture when exposed to UV light[150]. Copyright 2017, Hydrogen Energy Publications LLC. Published by Elsevier Ltd. All rights reserved. UV: Ultraviolet.
Muhammad Tahir synthesized Ni and montmorillonite (MMT)-loaded TiO2 (Ni-MMT/TiO2) nanocomposites using the sol-gel method. These nanocomposites exhibited significant photocatalytic activity for H2 production and the generation of C1-C3 or CH4/C2H6/C3H6 hydrocarbons from ethanol-water mixture under UV light irradiation. The 3 wt% Ni-10 wt% MMT/TiO2 nanocomposite exhibited the highest H2 production rate of 3,470 μmol·g-1·h-1 with a selectivity of 99%. This performance is 2.62 times greater than that of Ni-doped TiO2, 6.48 times higher than MMT/TiO2, and 6.45 orders of magnitude higher than that of pure TiO2. To assess the lifespan and reusability of the composite catalysts, stability tests were conducted over multiple cycles. Based on the experimental results, a reaction mechanism was proposed for the photocatalytic synthesis of H2 and CH4 from an ethanol-water mixture in a gas phase system, as depicted in Figure 17A and B. The energy level diagram of Ni-MMT/TiO2 photocatalysts for H2 production and the schematic representation of the photocatalytic H2 and hydrocarbon production over the MMT-Ni/TiO2 sample, as depicted in Figure 17C and D[150].
Figure 17. (A) Stability analysis of 3% Ni-10% MMT/TiO2 catalysts for photocatalytic H2 production and (B) CH4 production from ethanol-water reforming; (C) Energy level illustration of Ni-MMT/TiO2 photocatalysts for H2 production; (D) Schematic representation of photocatalytic H2 and hydrocarbon production over MMT-Ni/TiO2 samples[150]. Copyright 2017, Hydrogen Energy Publications LLC. Published by Elsevier Ltd. All rights reserved. MMT: Montmorillonite.
Zhang et al. synthesized TiO2-supported Au catalysts [Au@TiO2 (Ti3+)] using the sol-gel method, varying the concentration of the NaBH4 reducing agent to optimize the production of H2 and CH4 through ethanol photoreforming. Increasing the NaBH4 concentration led to a higher Ti3+ defect concentration, consequently boosting the number of oxygen vacancies (VOs) and the amount of electron-rich Au in TiO2. The catalytic activity of the catalysts improved with concentration of defect sites. The Au@TiO2 catalyst with the highest defect concentration exhibited exceptional performance during ethanol photoreforming, producing high-quality H2 at approximately 7,093 μmol·gcat-1·h-1 and CH4 via C–C bond cleavage of ethanol, without CO2 emission. Additionally, the catalyst improved charge carrier generation by enhancing visible light absorption, which accelerated the proton reduction rate to H2. The adsorption and activation of ethanol on the catalyst surface further contributed to the improved H2 production rate, as depicted in Figure 18[151].
Figure 18. The mechanism of ethanol photoreforming by Au@TiO2 photocatalysts into H2 and CH4[151]. Copyright 2019, American Chemical Society.
Li et al. synthesized CdS/metal-organic framework (MOF) composites, specifically CdS/MIL-53(Fe), using the hydrothermal method. These composites demonstrated efficient photocatalytic production of H2
Figure 19. (A) Photocatalytic H2 production and benzaldehyde; (B) Photocatalytic efficiency of dissimilar catalysts with 0.5 mmol benzyl alcohol, CH3CN5 mL, λ > 420 nm; (C) Graphic illustration of CdS/MIL-53(Fe) for the e-h transfer mechanism (Z-scheme)[152]. Copyright 2021, Elsevier B.V. All rights reserved.
Jiang et al. prepared Co/CdS photocatalysts by using an in situ photodeposition method for the simultaneous evolution of hydrogen and benzaldehyde under visible light. The benzaldehyde production yield reached 94.4%. The photocatalytic mechanism highlights the crucial role of benzyl alcohol oxidation in the selective production of benzaldehyde. Notably, the hydrogen evolution via photocatalytic dehydrogenation of benzyl alcohol reached a record-high quantum efficiency, with an average actual quantum content of 63.2% at 420 nm in a 50 vol% benzyl alcohol-acetonitrile solution, demonstrating optimal hydrogen evolution performance. This study provides a promising approach for developing highly efficient photosynthesis systems that do not require sacrificial electron donors or noble metal cocatalysts[153].
Tayyab et al. synthesized cadmium sulfide nanowires with vanadium carbide as a cocatalyst (VC/CdS) using the calcination method and superficial electrostatic self-assembly. This study demonstrated the use of VC/CdS photocatalysts for the simultaneous production of hydrogen and highly valued organic compounds in a single aqueous system or liquid organic waste. The VC/CS-15 composite exhibited the highest performance, significantly enhancing the selective oxidation of benzyl alcohol to benzaldehyde and simultaneous H2 generation. Under visible light irradiation (λ > 420 nm) for 2 h, the maximum hydrogen evolution rate reached 20.5 mmol·g-1·h-1, with a selectivity of over 99% for benzaldehyde synthesis. This performance is more than 661 times higher than that of pure CdS nanowires. The enhanced photocatalytic activity is attributed to VC, which lowers the zeta potential, attracts benzyl alcohol, creates active sites for hydrogen generation, and prolongs electron-hole pair separation, thereby increasing the overall efficiency of the photocatalytic process[154].
Zhang et al. synthesized silver nanoparticles (AgNPs)/CdS hybrid photocatalysts using a microwave-assisted method followed by the photodeposition method in the presence of methanol under vacuum conditions. The prepared photocatalyst was used to split ethanol into H2 and acetaldehyde under visible light at room temperature without producing Cox. The incorporation of AgNPs enhanced the stability and activity of the photocatalysts, enabling the use of light radiation above 650 nm. This increased electron-hole generation and decrease in charge carrier recombination expands the potential applications of photocatalysis. The AgNPs/CdS hybrid photocatalyst demonstrated over 40 times greater efficiency in ethanol dehydrogenation into H2 and acetaldehyde than pristine CdS, making it a highly favorable material for low-carbon, green chemistry, and sustainable development[155].
Fu et al. synthesized ZnSn(OH)6 nanocubes (ZHS) using a solvothermal technique to reform an aqueous ethanol solution to H2 and CH4. Various characterization techniques, including transmission electron microscopy, scanning electron microscopy, N2 sorption [Brunauer–Emmett–Teller (BET) surface area], X-ray diffraction, and UV-visible diffuse reflectance spectroscopy, were utilized to analyze the samples. Among the different samples, the ZHS-20 photocatalysts exhibited the highest photocatalytic activity. However, further enhancement was achieved by loading Pt onto the ZHS-20 surface. The Pt-loaded ZHS-20 catalyst demonstrated superior photocatalytic performance, significantly improving the production of H2 and CH4 from the ethanol-water mixture[156].
Li et al. fabricated Pd/HNb3O8-H2 photocatalysts using a thermal treatment method involving the integration of Pd2+ into HNb3O8 in a H2/Ar gas atmosphere. The presence of Pd facilitates the formation of surface VOs in HNb3O8, creating dual active sites for the concurrent production of hydrogen and benzaldehyde. The photoelectrochemical and spectroscopy investigations highlight the advantages of the Pd/HNb3O8-H2 photocatalyst in improving the separation and migration of photoinduced charge carriers. This catalyst demonstrated exceptional performance in the co-production of H2 and benzaldehyde from benzyl-alcohol solutions in water, achieving impressive rates of 3.17 and 2.76 mmol·g-1·h-1, respectively. By integrating experimental data and theoretical studies, the authors investigated the cooperative mechanism between Pd and surface VOs on HNb3O8, which significantly enhances the photocatalytic activity for hydrogen evolution coupled with the selective oxidation of benzyl alcohol, as illustrated in Figure 20[104].
Figure 20. (A) Photocatalytic chemical reaction yielding benzaldehyde and H2 simultaneously. Output rates of benzaldehyde and H2 over (B) various photocatalysts fabricated by several treatments; (C) photocatalysts through thermal treatment in numerous atmospheres; (D) optimized photocatalysts at different times prepared by thermal treatment; and (E) various M/HNb3O8-H2 (M = Pt, Ni, Pd, Au) photocatalysts acquired by thermal treatment[104]. Copyright 2021, Elsevier B.V. All rights reserved.
Liu et al. synthesized a Z-scheme MOF-derived Co9S8/CdS heterostructure using the hydrothermal method for H2 production and benzaldehyde synthesis. This MOF-derived Co9S8/CdS photocatalyst exhibited superior H2 production activity, reaching 61,924 μmol·g-1 in 6 h and a maximum benzaldehyde output, which was 21 and 16 times greater than that of bare CdS and Co9S8/CdS composite, respectively. Notably, the efficiency of the proposed system surpassed that of most CdS-based photocatalytic systems that rely on high concentrations of sacrificial agents. This study demonstrated a novel approach to constructing a Z-scheme heterostructure from a multivariate crystalline framework, significantly enhancing the co-production of valuable chemicals such as benzaldehyde and solar fuel without the need for sacrificial agents[157].
Zhang et al. fabricated non-metals such as phosphorus (P) and sulfur (S)-doped g-C3N4 hierarchical mesoporous spheres using the co-precipitation method for photocatalytic H2 and benzaldehyde production. Through experimental analysis and density functional theory (DFT) calculations, the study identified promising doping sites and their positive effects on electronic structure. The optimized S-doped g-C3N4 photocatalysts exhibited a 13.2-fold enhancement in H2 production compared to bare g-C3N4 under visible light irradiation. Additionally, S-doped g-C3N4 demonstrated outstanding dual-function photocatalytic performance, producing H2 and benzaldehyde from the oxidation of benzyl alcohol at rates of 3.76 and
Lin et al. prepared nickel-modified ZnIn2S4 (Ni:ZIS) by in situ photodeposition treatment for highly efficient photocatalytic H2 evolution and selective oxidation of benzyl alcohol to benzaldehyde, as illustrated in the schematic mechanism in Figure 21. The incorporation of Ni into ZnIn2S4 facilitates photogenerated charge transfer by promoting α-H abstraction, thereby enhancing H2 and benzaldehyde photoactivity under visible light irradiation. Further electron paramagnetic resonance (EPR) spectral analysis and quenching experiments confirmed that a radical mechanism centered on carbon catalyzes the photocatalytic benzyl alcohol conversion activity[159].
Figure 21. Schematic mechanism illustrating the photoredox-catalyzed transformation of benzyl alcohol and the evolution of H2 over Ni:ZIS in a N2 atmosphere using visible light (λ > 420 nm)[159]. Copyright 2020, Elsevier B.V. All rights reserved.
Hao et al. developed a ZnS/NixSy composite with dual functions for H2 evolution and benzaldehyde production via the selective oxidation of benzyl alcohols in anaerobic water under UV light. The composite facilitates a transition from hole-predominant oxidation on the ZnS surface to electron-initiating dehydrogenation of alcohols on NixSy nanoparticles. The NixSy nanoparticles exhibit a remarkable ability to abstract H atoms from alcohol and desorb the produced benzaldehyde, as confirmed by hydroxyl radical detection and various active species trapping experiments. In addition, compared with the equivalent acetonitrile system, the ZnS-NixSy composites demonstrated higher conversion efficiency in benzyl alcohol aqueous solution. This study highlights the potential of using NixSy nanoparticles as dehydrogenation units and for hydrogen evolution, thereby improving the performance of wide-band-gap catalysts for discriminating benzyl alcohol transformations coupled with hydrogen evolution in green aqueous solutions[160].
Wang et al. fabricated Pd-doped TiO2 with VOs (PdSA+C/TiO2-VO) using a facile pyrolysis method for the production of H2 and the oxidation of benzylamine into N-benzylidenebenzylamine. The PdSA+C/TiO2-VO photocatalyst, which contains Pd single atoms (SAs), clusters (C), and VOs, demonstrated superior performance compared with PdSA/TiO2-VO and TiO2-VO. The PdSA+C/TiO2-VO demonstrated remarkable production rates of H2 and N-benzylidenebenzylamine which were 52.7 and 1.5 times higher, respectively, than TiO2-VO, as illustrated in Figure 22. Theoretical and experimental studies have elucidated the synergistic mechanism of Pd SAs, clusters, and VOs on TiO2 in enhancing photocatalytic performance. Pd clusters facilitate efficient charge separation and provide optimal active sites for H2 evolution, whereas Pd SAs contribute to the formation and stabilization of VOs through the creation of a Pd + O + Ti3+ atomic interface[99].
Figure 22. Photocatalytic synergistic H2 production and N-benzylidene benzylamine. (A) Graphical illustration of the photocatalytic reaction; (B) evolution of H2 using various photocatalysts; (C) different amounts of H2 and N-benzylidenebenzylamine using various photocatalysts; (D) H2 evolution with different dosages of H2O and benzylamine over PdSA+C/TiO2-VO photocatalyst; and (E) production rates of N-benzylidenebenzylamine and H2 over the PdSA+C/TiO2-VO photocatalyst at varying dosages of H2O and benzylamine[99]. Copyright 2020, Wiley-VCH GmbH.
Chen et al. fabricated MoS2/ZnIn2S4 photocatalysts using a facile photochemical reduction method for the conversion of benzyl alcohol into hydrogen and valuable organic compounds such as benzaldehyde under visible light irradiation. The optimized 1%MoS2/ZnIn2S4 composite exhibited enhanced visible light absorption and photocatalytic activity, with benzyl alcohol conversion and H2 evolution rates of 3.69 and 3.88 mmol·g-1·h-1, respectively, which were six times higher than those of bare ZnIn2S4. The MoS2 cocatalyst accelerated the kinetics of H2 formation for collaborative production reactions by significantly enhancing charge separation and reducing the overpotential of the HER. The molecular suitability of MoS2 as a high-performance cocatalyst was further confirmed by examining the photoreduction of Cr (VI) and 4-nitroaniline (4-NA). A carbon-centered radical mechanism was identified for the conversion of benzyl alcohol by the MoS2/ZnIn2S4 photocatalyst[86].
Qi et al. fabricated a CdS/SiO2 composite using a grinding method by loading CdS quantum dots (QDs) onto spherical SiO2 to enable H2 evolution and the dehydrogenative C-C coupling of benzyl alcohol into hydrobenzoin. The CdS/SiO2 composite demonstrated enhanced stability and improved photoredox-catalytic activity, effectively facilitating the C-C coupling of benzyl alcohol to form hydrobenzoin and the evolution of H2, as depicted in Figure 23A-C[102].
Figure 23. (A) Scavenger test experiments of the optimized CdS/SiO2 photocatalyst with different scavengers at a constant concentration (24 mM) over a 12-h reaction period; (B) In situ, EPR spectra of CdS and CdS/SiO2 composites in CH3CN solution saturated with argon (Ar) in the presence of a DMPO scavenger; (C) Graphic diagram of simultaneous photocatalytic production of H2 and hydrobenzoin[102]. Copyright 2020, American Chemical Society. EPR: Electron paramagnetic resonance; DMPO: 5, 5-dimethyl-1-pyrroline N-oxide.
CONCLUSION
In conclusion, this review provides a comprehensive overview of the latest advancements in photocatalytic hydrogen production and simultaneous chemical synthesis from wastewater or liquid organic waste. The fundamental concepts of cooperative H2 production and photocatalytic selective organic synthesis were briefly reviewed, followed by an analysis of the critical requirements for semiconductors used in dual-function photocatalysis systems. Subsequently, examples of innovative materials and approaches used in this field to address current challenges and unlock the full potential of nanophotocatalysts were presented. Each example describes the associated photocatalytic reaction or mechanism. There are opportunities and challenges in the advancement of such dual-function photocatalysis. To achieve more effective solar light utilization and synergistic photocatalytic activity, the primary focus of catalyst preparation is the modification of its components and microstructure to improve light absorption, charge separation and migration, and redox capacity of the base catalyst. Adopting a binary analysis perspective, this study focuses on the following key points in order of importance:
(i) The necessity for dual-directional optimization in photocatalytic hydrogen production with synergistic organic synthesis.
(ii) The efficiency of photocatalytic hydrogen production is not always compromised by replacing sacrificial agents. Instead, properly integrated organic synthesis from wastewater or liquid organic waste can exert a multiplier effect.
(iii) The significant potential for advancements and the feasibility analysis of photocatalytic hydrogen production with synergistic organic synthesis.
More effort is required to modify catalysts for hydrogen production and organic synthesis from wastewater or liquid organic waste because this technology remains in its early stages of development and faces significant challenges for broader applications.
DECLARATIONS
Acknowledgments
The authors are thankful to the Australian National University (ANU).
Authors’ contributions
Investigation, writing - original draft: Hussain, M. K.
Investigation, analysis: Liu, R.
Analysis: Tanveer, M.; Khalid, N. R.
Conceptualization, supervision: Yin, Z.
Availability of data and materials
Not applicable.
Financial support and sponsorship
The authors acknowledge the financial support from the Australian Research Council (FT230100059, DP240100687, IH220100012).
Conflicts of interest
All authors declared that there are no conflicts of interest.
Ethical approval and consent to participate
Not applicable.
Consent for publication
Not applicable.
Copyright
© The Author(s) 2025.
REFERENCES
1. Gong, Y.; Wang, J.; Wei, Z.; Zhang, P.; Li, H.; Wang, Y. Combination of carbon nitride and carbon nanotubes: synergistic catalysts for energy conversion. ChemSusChem 2014, 7, 2303-9.
2. Dai, L.; Chang, D. W.; Baek, J. B.; Lu, W. Carbon nanomaterials for advanced energy conversion and storage. Small 2012, 8, 1130-66.
3. George, C.; Ammann, M.; D’Anna, B.; Donaldson, D. J.; Nizkorodov, S. A. Heterogeneous photochemistry in the atmosphere. Chem. Rev. 2015, 115, 4218-58.
4. Hussain, M. K.; Khalid, N.; Tanveer, M.; Kebaili, I.; Alrobei, H. Fabrication of CuO/MoO3 p-n heterojunction for enhanced dyes degradation and hydrogen production from water splitting. Int. J. Hydrogen. Energy. 2022, 47, 15491-504.
5. Bard, A. J.; Fox, M. A. Artificial photosynthesis: solar splitting of water to hydrogen and oxygen. Acc. Chem. Res. 1995, 28, 141-5.
6. Yang, J.; Wang, D.; Han, H.; Li, C. Roles of cocatalysts in photocatalysis and photoelectrocatalysis. Acc. Chem. Res. 2013, 46, 1900-9.
7. Tu, W.; Zhou, Y.; Zou, Z. Versatile graphene-promoting photocatalytic performance of semiconductors: basic principles, synthesis, solar energy conversion, and environmental applications. Adv. Funct. Mater. 2013, 23, 4996-5008.
8. Fan, W.; Zhang, Q.; Wang, Y. Semiconductor-based nanocomposites for photocatalytic H2 production and CO2 conversion. Phys. Chem. Chem. Phys. 2013, 15, 2632-49.
9. Wondraczek, L.; Tyystjärvi, E.; Méndez-Ramos, J.; Müller, F. A.; Zhang, Q. Shifting the sun: solar spectral conversion and extrinsic sensitization in natural and artificial photosynthesis. Adv. Sci. 2015, 2, 1500218.
10. Kapilashrami, M.; Zhang, Y.; Liu, Y. S.; Hagfeldt, A.; Guo, J. Probing the optical property and electronic structure of TiO2 nanomaterials for renewable energy applications. Chem. Rev. 2014, 114, 9662-707.
11. Kubacka, A.; Fernández-García, M.; Colón, G. Advanced nanoarchitectures for solar photocatalytic applications. Chem. Rev. 2012, 112, 1555-614.
12. Pan, J.; Wang, B.; Shen, S.; Chen, L.; Yin, S. Introducing bidirectional axial coordination into BiVO4@metal phthalocyanine core–shell photoanodes for efficient water oxidation. Angew. Chem. Int. Ed. Engl. 2023, 135, e202307246.
13. Li, H.; Zhou, Y.; Tu, W.; Ye, J.; Zou, Z. State-of-the-art progress in diverse heterostructured photocatalysts toward promoting photocatalytic performance. Adv. Funct. Mater. 2015, 25, 998-1013.
14. Zhang, H.; Liu, G.; Shi, L.; Liu, H.; Wang, T.; Ye, J. Engineering coordination polymers for photocatalysis. Nano. Energy. 2016, 22, 149-68.
15. Hussain, M. K.; Khalid, N.; Tanveer, M.; et al. In-situ fabrication of MoO3 hexagonal flowers decorated with Co3O4 microrods with enhanced photocatalytic activity and stability under visible light irradiation. Mater. Chem. Phys. 2023, 302, 127652.
16. Chen, G.; Waterhouse, G. I. N.; Shi, R.; et al. From solar energy to fuels: recent advances in light-driven C1 chemistry. Angew. Chem. Int. Ed. Engl. 2019, 58, 17528-51.
17. Mustafa, A.; Lougou, B. G.; Shuai, Y.; Wang, Z.; Tan, H. Current technology development for CO2 utilization into solar fuels and chemicals: a review. J. Energy. Chem. 2020, 49, 96-123.
18. Qi, J.; Zhang, W.; Cao, R. Solar-to-hydrogen energy conversion based on water splitting. Adv. Energy. Mater. 2018, 8, 1701620.
19. Aslam, U.; Rao, V. G.; Chavez, S.; Linic, S. Catalytic conversion of solar to chemical energy on plasmonic metal nanostructures. Nat. Catal. 2018, 1, 656-65.
20. Wang, Q.; Pornrungroj, C.; Linley, S.; Reisner, E. Strategies to improve light utilization in solar fuel synthesis. Nat. Energy. 2022, 7, 13-24.
21. Agosti, A.; Nakibli, Y.; Amirav, L.; Bergamini, G. Photosynthetic H2 generation and organic transformations with CdSe@CdS-Pt nanorods for highly efficient solar-to-chemical energy conversion. Nano. Energy. 2020, 70, 104510.
22. Xu, X.; Su, C.; Shao, Z. Fundamental understanding and application of Ba0.5Sr0.5Co0.8Fe0.2O3-δ perovskite in energy storage and conversion: past, present, and future. Energy. Fuels. 2021, 35, 13585-609.
23. Zhou, B.; Zhou, P.; Dong, W.; Mi, Z. Gallium nitride-based artificial photosynthesis integrated devices for solar hydrogen generation and carbon dioxide reduction. In: Varghese OK, Souza FL, editors. Conversion of water and CO2 to fuels using solar energy. Wiley; 2024. pp. 309-39.
24. Qi, M. Y.; Conte, M.; Anpo, M.; Tang, Z. R.; Xu, Y. J. Cooperative coupling of oxidative organic synthesis and hydrogen production over semiconductor-based photocatalysts. Chem. Rev. 2021, 121, 13051-85.
25. Franchi, D.; Amara, Z. Applications of sensitized semiconductors as heterogeneous visible-light photocatalysts in organic synthesis. ACS. Sustainable. Chem. Eng. 2020, 8, 15405-29.
26. Gisbertz, S.; Pieber, B. Heterogeneous photocatalysis in organic synthesis. ChemPhotoChem 2020, 4, 456-75.
27. Molinari, R.; Lavorato, C.; Argurio, P. Recent progress of photocatalytic membrane reactors in water treatment and in synthesis of organic compounds. a review. Catal. Today. 2017, 281, 144-64.
28. Friedmann, D.; Hakki, A.; Kim, H.; Choi, W.; Bahnemann, D. Heterogeneous photocatalytic organic synthesis: state-of-the-art and future perspectives. Green. Chem. 2016, 18, 5391-411.
29. Dai, X.; Xie, M.; Meng, S.; Fu, X.; Chen, S. Coupled systems for selective oxidation of aromatic alcohols to aldehydes and reduction of nitrobenzene into aniline using CdS/g-C3N4 photocatalyst under visible light irradiation. Appl. Catal. B. Environ. 2014, 158-9, 382-90.
30. Huang, H.; Jin, Y.; Chai, Z.; et al. Surface charge-induced activation of Ni-loaded CdS for efficient and robust photocatalytic dehydrogenation of methanol. Appl. Catal. B. Environ. 2019, 257, 117869.
31. Bie, C.; Wang, L.; Yu, J. Challenges for photocatalytic overall water splitting. Chem 2022, 8, 1567-74.
32. Rahman, M. Z.; Raziq, F.; Zhang, H.; Gascon, J. Key strategies for enhancing H2 production in transition metal oxide based photocatalysts. Angew. Chem. Int. Ed. Engl. 2023, 135, e202305385.
33. Nishioka, S.; Osterloh, F. E.; Wang, X.; Mallouk, T. E.; Maeda, K. Photocatalytic water splitting. Nat. Rev. Methods. Primers. 2023, 3, 226.
34. Ismael, M. A review and recent advances in solar-to-hydrogen energy conversion based on photocatalytic water splitting over doped-TiO2 nanoparticles. Solar. Energy. 2020, 211, 522-46.
35. Wang, Z.; Li, C.; Domen, K. Recent developments in heterogeneous photocatalysts for solar-driven overall water splitting. Chem. Soc. Rev. 2019, 48, 2109-25.
36. Miseki, Y.; Sayama, K. Photocatalytic water splitting for solar hydrogen production using the carbonate effect and the Z-scheme reaction. Adv. Energy. Mater. 2019, 9, 1801294.
37. Wang, Y.; Silveri, F.; Bayazit, M. K.; et al. Bandgap engineering of organic semiconductors for highly efficient photocatalytic water splitting. Adv. Energy. Mater. 2018, 8, 1801084.
38. Maeda, K.; Teramura, K.; Lu, D.; et al. Photocatalyst releasing hydrogen from water. Nature 2006, 440, 295.
39. Yuan, Y. J.; Lu, H. W.; Yu, Z. T.; Zou, Z. G. Noble-metal-free molybdenum disulfide cocatalyst for photocatalytic hydrogen production. ChemSusChem 2015, 8, 4113-27.
40. Moniz, S. J. A.; Shevlin, S. A.; Martin, D. J.; Guo, Z.; Tang, J. Visible-light driven heterojunction photocatalysts for water splitting - a critical review. Energy. Environ. Sci. 2015, 8, 731-59.
41. Lu, Q.; Yu, Y.; Ma, Q.; Chen, B.; Zhang, H. 2D transition-metal-dichalcogenide-nanosheet-based composites for photocatalytic and electrocatalytic hydrogen evolution reactions. Adv. Mater. 2016, 28, 1917-33.
42. Zhang, N.; Qu, Y.; Pan, K.; Wang, G.; Li, Y. Synthesis of pure phase Mg1.2Ti1.8O5 and MgTiO3 nanocrystals for photocatalytic hydrogen production. Nano. Res. 2016, 9, 726-34.
43. Fujishima, A.; Honda, K. Electrochemical photolysis of water at a semiconductor electrode. Nature 1972, 238, 37-8.
44. Yang, X.; Singh, D.; Ahuja, R. Recent advancements and future prospects in ultrathin 2D semiconductor-based photocatalysts for water splitting. Catalysts 2020, 10, 1111.
45. Zhong, S.; Xi, Y.; Wu, S.; Liu, Q.; Zhao, L.; Bai, S. Hybrid cocatalysts in semiconductor-based photocatalysis and photoelectrocatalysis. J. Mater. Chem. A. 2020, 8, 14863-94.
46. Zhang, Y.; Xia, B.; Ran, J.; Davey, K.; Qiao, S. Z. Atomic-level reactive sites for semiconductor-based photocatalytic CO2 reduction. Adv. Energy. Mater. 2020, 10, 1903879.
47. Chen, S.; Huang, D.; Xu, P.; et al. Semiconductor-based photocatalysts for photocatalytic and photoelectrochemical water splitting: will we stop with photocorrosion? J. Mater. Chem. A. 2020, 8, 2286-322.
48. Tahir, M.; Asiri, A. M.; Nawaz, T. A perspective on the fabrication of heterogeneous photocatalysts for enhanced hydrogen production. Int. J. Hydrogen. Energy. 2020, 45, 24544-57.
49. Wang, L.; Sasaki, T. Titanium oxide nanosheets: graphene analogues with versatile functionalities. Chem. Rev. 2014, 114, 9455-86.
50. Bai, S.; Jiang, W.; Li, Z.; Xiong, Y. Surface and interface engineering in photocatalysis. ChemNanoMat 2015, 1, 223-39.
51. Martin, D. J.; Liu, G.; Moniz, S. J.; et al. Efficient visible driven photocatalyst, silver phosphate: performance, understanding and perspective. Chem. Soc. Rev. 2015, 44, 7808-28.
52. Alam, M.; Azam, H.; Khalid, N.; et al. Enhanced photocatalytic performance of Ag3PO4/Mn-ZnO nanocomposite for the degradation of tetracycline hydrochloride. Crystals 2022, 12, 1156.
53. Hussain, M. K.; Khalid, N.; Tanveer, M.; et al. Facile fabrication of Z-scheme ZnO/MoO3 heterojunction as an excellent visible-light responsive photocatalyst for the degradation of rhodamine B and alizarin yellow dyes. Opt. Mater. 2024, 148, 114794.
54. Khalid, N.; Hammad, A.; Tahir, M.; et al. Enhanced photocatalytic activity of Al and Fe co-doped ZnO nanorods for methylene blue degradation. Ceram. Int. 2019, 45, 21430-5.
55. Khalid, N. R.; Hussain, M. K.; Murtaza, G.; Ikram, M.; Ahmad, M.; Hammad, A. A novel Ag2O/Fe–TiO2 photocatalyst for CO2 conversion into methane under visible light. J. Inorg. Organomet. Polym. 2019, 29, 1288-96.
56. Banisharif, A.; Khodadadi, A. A.; Mortazavi, Y.; et al. Highly active Fe2O3-doped TiO2 photocatalyst for degradation of trichloroethylene in air under UV and visible light irradiation: experimental and computational studies. Appl. Catal. B. Environ. 2015, 165, 209-21.
57. Hussain, M. K.; Khalid, N.; Tahir, M.; Tanveer, M.; Iqbal, T.; Liaqat, M. Enhanced visible light-driven photocatalytic activity and stability of novel ternary ZnO/CuO/MoO3 nanorods for the degradation of rhodamine B and alizarin yellow. Mater. Sci. Semicond. Process. 2023, 155, 107261.
58. Zhu, D.; Zhou, Q. Novel Bi2WO6 modified by N-doped graphitic carbon nitride photocatalyst for efficient photocatalytic degradation of phenol under visible light. Appl. Catal. B. Environ. 2020, 268, 118426.
59. He, W.; Sun, Y.; Jiang, G.; Huang, H.; Zhang, X.; Dong, F. Activation of amorphous Bi2WO6 with synchronous Bi metal and Bi2O3 coupling: photocatalysis mechanism and reaction pathway. Appl. Catal. B. Environ. 2018, 232, 340-7.
60. Tanveer, M.; Cheema, H. H.; Nabi, G.; Ali, A. R.; Hussain, M. K.; Qadeer, M. A novel composite (BiVO4/TiS2) presenting an excellent Z-scheme photocatalytic degradation for Rhodamine B dye under the visible light irradiation. J. Lumin. 2024, 271, 120585.
61. Tayebi, M.; Lee, B. Recent advances in BiVO4 semiconductor materials for hydrogen production using photoelectrochemical water splitting. Renew. Sustain. Energy. Rev. 2019, 111, 332-43.
62. Gurylev, V. A review on the development and advancement of Ta2O5 as a promising photocatalyst. Mater. Today. Sustain. 2022, 18, 100131.
63. Liu, W.; Liao, M.; Huang, S.; Reyes, Y. I. A.; Tiffany, C. H.; Perng, T. Formation and characterization of gray Ta2O5 and its enhanced photocatalytic hydrogen generation activity. Int. J. Hydrogen. Energy. 2020, 45, 16560-8.
64. Arunachalam, P.; Nagai, K.; Amer, M. S.; Ghanem, M. A.; Ramalingam, R. J.; Al-Mayouf, A. M. Recent developments in the use of heterogeneous semiconductor photocatalyst based materials for a visible-light-induced water-splitting system - a brief review. Catalysts 2021, 11, 160.
65. Qadeer M, Khalid Hussain M, Tanveer M, Munawar S, Nabi G, Henaish A. Sol-gel extended hydrothermal synthesis of BiFeO3 nano-beads for excellent photocatalytic and photo-electrochemical properties under natural light irradiation. Inorg. Chem. Commun. 2023, 158, 111617.
66. Zhao, Y.; Liu, W.; Liu, P.; et al. In situ photodeposition of Au nanoparticle plasma: enhanced defect-state g-C3N4 photocatalytic hydrogen evolution. Cryst. Growth. Des. 2024, 24, 5794-805.
67. Cheng, L.; Xiang, Q.; Liao, Y.; Zhang, H. CdS-based photocatalysts. Energy. Environ. Sci. 2018, 11, 1362-91.
68. Sun, Y.; Cheng, H.; Gao, S.; et al. Freestanding tin disulfide single-layers realizing efficient visible-light water splitting. Angew. Chem. Int. Ed. Engl. 2012, 124, 8857-61.
69. Wang, B.; Wang, Y.; Lei, Y.; et al. Mesoporous silicon carbide nanofibers with in situ embedded carbon for co-catalyst free photocatalytic hydrogen production. Nano. Res. 2016, 9, 886-98.
70. Li, B.; Hu, Y.; Shen, Z.; et al. Photocatalysis driven by near-infrared light: materials design and engineering for environmentally friendly photoreactions. ACS. EST. Eng. 2021, 1, 947-64.
71. Tan, L.; Ong, W.; Chai, S.; Goh, B. T.; Mohamed, A. R. Visible-light-active oxygen-rich TiO2 decorated 2D graphene oxide with enhanced photocatalytic activity toward carbon dioxide reduction. Appl. Catal. B. Environ. 2015, 179, 160-70.
72. Tan, L.; Ong, W.; Chai, S.; Mohamed, A. R. Visible-light-activated oxygen-rich TiO2 as next generation photocatalyst: importance of annealing temperature on the photoactivity toward reduction of carbon dioxide. Chem. Eng. J. 2016, 283, 1254-63.
73. Rashid, R.; Shafiq, I.; Gilani, M. R. H. S.; et al. Advancements in TiO2-based photocatalysis for environmental remediation: strategies for enhancing visible-light-driven activity. Chemosphere 2024, 349, 140703.
74. Jiang, A.; Guo, H.; Yu, S.; et al. Dual charge-accepting engineering modified AgIn5S8/CdS quantum dots for efficient photocatalytic hydrogen evolution overall H2S splitting. Appl. Catal. B. Environ. 2023, 332, 122747.
75. Jeon, T. H.; Koo, M. S.; Kim, H.; Choi, W. Dual-functional photocatalytic and photoelectrocatalytic systems for energy- and resource-recovering water treatment. ACS. Catal. 2018, 8, 11542-63.
77. Dashtian, K.; Shahsavarifar, S.; Usman, M.; et al. A comprehensive review on advances in polyoxometalate based materials for electrochemical water splitting. Coord. Chem. Rev. 2024, 504, 215644.
78. Kampouri, S.; Stylianou, K. C. Dual-functional photocatalysis for simultaneous hydrogen production and oxidation of organic substances. ACS. Catal. 2019, 9, 4247-70.
79. Sun, W.; Zheng, Y.; Zhu, J. A “win-win” photocatalysis: coupling hydrogen production with the synthesis of high value-added organic chemicals. Mater. Today. Sustain. 2023, 23, 100465.
80. Schrauzer, G. N.; Guth, T. D. Photolysis of water and photoreduction of nitrogen on titanium dioxide. J. Am. Chem. Soc. 1977, 99, 7189-93.
81. Mills, A.; Le, H. S. An overview of semiconductor photocatalysis. J. Photochem. Photobiol. A. Chem. 1997, 108, 1-35.
82. Kampouri, S.; Ireland, C. P.; Valizadeh, B.; et al. Mixed-phase MOF-derived titanium dioxide for photocatalytic hydrogen evolution: the impact of the templated morphology. ACS. Appl. Energy. Mater. 2018, 1, 6541-8.
83. Wang, Q.; Domen, K. Particulate photocatalysts for light-driven water splitting: mechanisms, challenges, and design strategies. Chem. Rev. 2020, 120, 919-85.
84. Xie, S.; Shen, Z.; Deng, J.; et al. Visible light-driven C-H activation and C-C coupling of methanol into ethylene glycol. Nat. Commun. 2018, 9, 1181.
85. Chen, S.; Takata, T.; Domen, K. Particulate photocatalysts for overall water splitting. Nat. Rev. Mater. 2017, 2, 17050.
86. Chen, Z. H.; Li, Y. H.; Qi, M. Y.; Tang, Z. R.; Xu, Y. J. Benzyl alcohol oxidation and hydrogen generation over MoS2/ZnIn2S4 composite photocatalyst. Res. Chem. Intermed. 2022, 48, 1-12.
87. Liu, H.; Xu, C.; Li, D.; Jiang, H. Photocatalytic hydrogen production coupled with selective benzylamine oxidation over MOF composites. Angew. Chem. Int. Ed. Engl. 2018, 130, 5477-81.
88. Luo, N.; Montini, T.; Zhang, J.; et al. Visible-light-driven coproduction of diesel precursors and hydrogen from lignocellulose-derived methylfurans. Nat. Energy. 2019, 4, 575-84.
89. Zhang, X.; Liu, T.; Zhao, F.; Zhang, N.; Wang, Y. In-situ-formed Cd and Ag2S decorated CdS photocatalyst with boosted charge carrier spatial separation for enhancing UV-vis-NIR photocatalytic hydrogen evolution. Appl. Catal. B. Environ. 2021, 298, 120620.
90. Naseri, A.; Asghari, S. G.; Samadi, M.; Yousefi, M.; Ebrahimi, M.; Moshfegh, A. Z. Recent advances on dual-functional photocatalytic systems for combined removal of hazardous water pollutants and energy generation. Res. Chem. Intermed. 2022, 48, 911-33.
91. Zhu, T.; Ye, X.; Zhang, Q.; Hui, Z.; Wang, X.; Chen, S. Efficient utilization of photogenerated electrons and holes for photocatalytic redox reactions using visible light-driven Au/ZnIn2S4 hybrid. J. Hazard. Mater. 2019, 367, 277-85.
92. Zhang, S.; Wang, K.; Li, F.; Ho, S. Structure-mechanism relationship for enhancing photocatalytic H2 production. Int. J. Hydrogen. Energy. 2022, 47, 37517-30.
93. Chen, X.; Shen, S.; Guo, L.; Mao, S. S. Semiconductor-based photocatalytic hydrogen generation. Chem. Rev. 2010, 110, 6503-70.
94. Rahman, M. Z.; Edvinsson, T.; Gascon, J. Hole utilization in solar hydrogen production. Nat. Rev. Chem. 2022, 6, 243-58.
95. Ran, J.; Zhang, J.; Yu, J.; Jaroniec, M.; Qiao, S. Z. Earth-abundant cocatalysts for semiconductor-based photocatalytic water splitting. Chem. Soc. Rev. 2014, 43, 7787-812.
96. Zhou, P.; Navid, I. A.; Ma, Y.; et al. Solar-to-hydrogen efficiency of more than 9% in photocatalytic water splitting. Nature 2023, 613, 66-70.
97. Liu, S.; Lin, P.; Wu, M.; et al. Organic dyes with multi-branched structures for highly efficient photocatalytic hydrogen evolution under visible-light irradiation. Appl. Catal. B. Environ. 2022, 309, 121257.
98. Yang, Y.; Tan, H.; Cheng, B.; Fan, J.; Yu, J.; Ho, W. Near-infrared-responsive photocatalysts. Small. Methods. 2021, 5, e2001042.
99. Wang, T.; Tao, X.; Li, X.; Zhang, K.; Liu, S.; Li, B. Synergistic Pd single atoms, clusters, and oxygen vacancies on TiO2 for photocatalytic hydrogen evolution coupled with selective organic oxidation. Small 2021, 17, e2006255.
100. Rusinque, B.; Escobedo, S.; de, L. H. Hydrogen production via Pd-TiO2 photocatalytic water splitting under near-UV and visible light: analysis of the reaction mechanism. Catalysts 2021, 11, 405.
101. Lyubina, T. P.; Markovskaya, D. V.; Kozlova, E. A.; Parmon, V. N. Photocatalytic hydrogen evolution from aqueous solutions of glycerol under visible light irradiation. Int. J. Hydrogen. Energy. 2013, 38, 14172-9.
102. Qi, M.; Li, Y.; Anpo, M.; Tang, Z.; Xu, Y. Efficient photoredox-mediated C–C coupling organic synthesis and hydrogen production over engineered semiconductor quantum dots. ACS. Catal. 2020, 10, 14327-35.
103. Jia, Q.; Zhang, S.; Jia, X.; Dong, X.; Gao, Z.; Gu, Q. Photocatalytic coupled redox cycle for two organic transformations over Pd/carbon nitride composites. Catal. Sci. Technol. 2019, 9, 5077-89.
104. Li, X.; Wang, T.; Zheng, Z.; Yang, Q.; Li, C.; Li, B. Pd modified defective HNb3O8 with dual active sites for photocatalytic coproduction of hydrogen fuel and value-added chemicals. Appl. Catal. B. Environ. 2021, 296, 120381.
105. Pomilla, F.; García-lópez, E.; Marcì, G.; Palmisano, L.; Parrino, F. Heterogeneous photocatalytic materials for sustainable formation of high-value chemicals in green solvents. Mater. Today. Sustain. 2021, 13, 100071.
106. Changotra, R.; Ray, A. K.; He, Q. Establishing a water-to-energy platform via dual-functional photocatalytic and photoelectrocatalytic systems: a comparative and perspective review. Adv. Colloid. Interface. Sci. 2022, 309, 102793.
107. Liu, J.; Guðmundsson, A.; Bäckvall, J. E. Efficient aerobic oxidation of organic molecules by multistep electron transfer. Angew. Chem. Int. Ed. Engl. 2021, 60, 15686-704.
108. Klibanov, A. M. Asymmetric enzymatic oxidoreductions in organic solvents. Curr. Opin. Biotechnol. 2003, 14, 427-31.
109. Yadav, M.; Joshi, C.; Paritosh, K.; et al. Organic waste conversion through anaerobic digestion: a critical insight into the metabolic pathways and microbial interactions. Metab. Eng. 2022, 69, 323-37.
110. Caudillo-Flores, U.; Fuentes-Moyado, S.; Fernández-García, M.; Kubacka, A. Effect of niobium on the performance of Pd-TiO2 photocatalysts for hydrogen production. Catal. Today. 2023, 419, 114147.
111. Yan, Z.; Yin, K.; Xu, M.; et al. Photocatalysis for synergistic water remediation and H2 production: a review. Chem. Eng. J. 2023, 472, 145066.
112. Khatami, M.; Iravani, S. Green and eco-friendly synthesis of nanophotocatalysts: an overview. Comments. Inorg. Chem. 2021, 41, 133-87.
113. Li, X.; Wang, L.; Fan, Y.; Feng, Q.; Cui, F.; Zhang, S. Biocompatibility and toxicity of nanoparticles and nanotubes. J. Nanomater. 2012, 2012, 548389.
114. Saravanan, A.; Kumar, P. S.; Hemavathy, R. V.; et al. A review on synthesis methods and recent applications of nanomaterial in wastewater treatment: challenges and future perspectives. Chemosphere 2022, 307, 135713.
115. Kalirajan, C.; Dukle, A.; Nathanael, A. J.; Oh, T. H.; Manivasagam, G. A critical review on polymeric biomaterials for biomedical applications. Polymers 2021, 13, 3015.
116. Bokov, D.; Turki, J. A.; Chupradit, S.; et al. Nanomaterial by sol-gel method: synthesis and application. Adv. Mater. Sci. Eng. 2021, 2021, 5102014.
117. Esposito, S. “Traditional” sol-gel chemistry as a powerful tool for the preparation of supported metal and metal oxide catalysts. Materials 2019, 12, 668.
118. Navas, D.; Fuentes, S.; Castro-Alvarez, A.; Chavez-Angel, E. Review on sol-gel synthesis of perovskite and oxide nanomaterials. Gels 2021, 7, 275.
119. Komarneni, S.; Roy, R.; Li, Q. Microwave-hydrothermal synthesis of ceramic powders. Mater. Res. Bull. 1992, 27, 1393-405.
120. Hussain, M. K.; Khalid, N. Surfactant-assisted synthesis of MoO3 nanorods and its application in photocatalytic degradation of different dyes in aqueous environment. J. Mol. Liq. 2022, 346, 117871.
121. Fu, Q.; Cao, C.; Zhu, H. A solvothermal synthetic route to prepare polycrystalline carbon nitride. Chem. Phys. Lett. 1999, 314, 223-6.
122. Walton, R. I. Subcritical solvothermal synthesis of condensed inorganic materials. Chem. Soc. Rev. 2002, 31, 230-8.
123. Khater, G. A.; Nabawy, B. S.; El-Kheshen, A. A.; Abdel, L. M. A.; Farag, M. M. Use of arc furnace slag and ceramic sludge for the production of lightweight and highly porous ceramic materials. Materials 2022, 15, 1112.
125. Otitoju T, Ugochukwu Okoye P, Chen G, Li Y, Onyeka Okoye M, Li S. Advanced ceramic components: materials, fabrication, and applications. J. Ind. Eng. Chem. 2020, 85, 34-65.
126. Schwarz, J. A.; Contescu, C.; Contescu, A. Methods for preparation of catalytic materials. Chem. Rev. 1995, 95, 477-510.
127. Shukla, A.; Singh, S. C.; Bhardwaj, A.; et al. Calcination temperature induced structural, optical and magnetic transformations in titanium ferrite nanoparticles. Reactions 2022, 3, 224-32.
128. Bogdanović, X.; Hinrichs, W. Influence of temperature during crystallization setup on precipitate formation and crystal shape of a metalloendopeptidase. Acta. Crystallogr. Sect. F. Struct. Biol. Cryst. Commun. 2011, 67, 421-3.
129. Theiss, F. L.; Ayoko, G. A.; Frost, R. L. Synthesis of layered double hydroxides containing Mg2+, Zn2+, Ca2+ and Al3+ layer cations by co-precipitation methods - a review. Appl. Surf. Sci. 2016, 383, 200-13.
130. Dikshit, P.; Kumar, J.; Das, A.; et al. Green synthesis of metallic nanoparticles: applications and limitations. Catalysts 2021, 11, 902.
131. Li, X.; Xu, H.; Chen, Z.; Chen, G. Biosynthesis of nanoparticles by microorganisms and their applications. J. Nanomater. 2011, 2011, 1-16.
132. Dridi, S.; Bitri, N.; Mahjoubi, S.; Chaabouni, F.; Ly, I. One-step spray of Cu2NiSnS4 thin films as absorber materials for photovoltaic applications. J. Mater. Sci. Mater. Electron. 2020, 31, 7193-9.
133. Mazzotta, A.; Carlotti, M.; Mattoli, V. Conformable on-skin devices for thermo-electro-tactile stimulation: materials, design, and fabrication. Mater. Adv. 2021, 2, 1787-820.
134. Iguchi, S.; Teramura, K.; Hosokawa, S.; Tanaka, T. A ZnTa2O6 photocatalyst synthesized via solid state reaction for conversion of CO2 into CO in water. Catal. Sci. Technol. 2016, 6, 4978-85.
135. Bouddouch, A.; Amaterz, E.; Bakiz, B.; et al. Phase transformation, photocatalytic and photoluminescent properties of BiPO4 catalysts prepared by solid-state reaction: degradation of rhodamine B. Minerals 2021, 11, 1007.
136. Mazumdar, S. C.; Datta, S.; Alam, F. Structural, magnetic and transport properties of Gd and Cu Co-doped BiFeO3 multiferroics. J. Appl. Mathemat. Phys. 2022, 10, 2026-39.
137. Zhao, W.; Luo, L.; Cong, M.; et al. Nanoscale covalent organic frameworks for enhanced photocatalytic hydrogen production. Nat. Commun. 2024, 15, 6482.
138. Khalid, N. R.; Arshad, A.; Tahir, M. B.; Hussain, M. K. Fabrication of p–n heterojunction Ag2O@Ce2O nanocomposites make enables to improve photocatalytic activity under visible light. Appl. Nanosci. 2021, 11, 199-206.
139. Choudhary, R. K.; Kumaraswamy, G. N.; Baitha, R.; et al. Synthesis of BNiO3 nanocomposites for photocatalytic hydrogen production applications. J. Inst. Eng. India. Ser. D.2024.
140. Thakur, A.; Manisha; Kumar, I.; Sharma, U. Visible light-induced functionalization of C−H bonds: opening of new avenues in organic synthesis. Asian. J. Org. Chem. 2022, 11, e202100804.
141. Shang, F. K.; Qi, M. Y.; Tan, C. L.; Tang, Z. R.; Xu, Y. J. Nanoscale assembly of CdS/BiVO4 hybrids for coupling selective fine chemical synthesis and hydrogen production under visible light. ACS. Phys. Chem. Au. 2022, 2, 216-24.
142. Luo, J.; Wang, M.; Chen, L.; Shi, J. Efficient benzaldehyde photosynthesis coupling photocatalytic hydrogen evolution. J. Energy. Chem. 2022, 66, 52-60.
143. Pang, Y.; Uddin, M. N.; Chen, W.; et al. Colloidal single-layer photocatalysts for methanol-storable solar H2 fuel. Adv. Mater. 2019, 31, e1905540.
144. Liu, Z.; Yin, Z.; Cox, C.; et al. Room temperature stable COx-free H2 production from methanol with magnesium oxide nanophotocatalysts. Sci. Adv. 2016, 2, e1501425.
145. Cui, W.; Feng, L.; Xu, C.; Lü, S.; Qiu, F. Hydrogen production by photocatalytic decomposition of methanol gas on Pt/TiO2 nano-film. Catal. Commun. 2004, 5, 533-6.
146. Gazsi, A.; Schubert, G.; Bánsági, T.; Solymosi, F. Photocatalytic decompositions of methanol and ethanol on Au supported by pure or N-doped TiO2. J. Photoch. Photobio. A. 2013, 271, 45-55.
147. Uddin, N.; Langley, J.; Zhang, C.; et al. Zero-emission multivalorization of light alcohols with self-separable pure H2 fuel. Appl. Catal. B. Environ. 2021, 292, 120212.
148. Tan, H.; Kong, P.; Liu, M.; Gu, X.; Zheng, Z. Enhanced photocatalytic hydrogen production from aqueous-phase methanol reforming over cyano-carboxylic bifunctionally-modified carbon nitride. Chem. Commun. 2019, 55, 12503-6.
149. Zhang, J.; Toe, C. Y.; Kumar, P.; Scott, J.; Amal, R. Engineering defects in TiO2 for the simultaneous production of hydrogen and organic products. Appl. Catal. B. Environ. 2023, 333, 122765.
150. Tahir, M. Ni/MMT-promoted TiO2 nanocatalyst for dynamic photocatalytic H2 and hydrocarbons production from ethanol-water mixture under UV-light. Int. J. Hydrogen. Energy. 2017, 42, 28309-26.
151. Zhang, X.; Luo, L.; Yun, R.; Pu, M.; Zhang, B.; Xiang, X. Increasing the activity and selectivity of TiO2-supported Au catalysts for renewable hydrogen generation from ethanol photoreforming by engineering Ti3+ defects. ACS. Sustainable. Chem. Eng. 2019, 7, 13856-64.
152. Li, P.; Yan, X.; Gao, S.; Cao, R. Boosting photocatalytic hydrogen production coupled with benzyl alcohol oxidation over CdS/metal–organic framework composites. Chem. Eng. J. 2021, 421, 129870.
153. Jiang, D.; Chen, X.; Zhang, Z.; et al. Highly efficient simultaneous hydrogen evolution and benzaldehyde production using cadmium sulfide nanorods decorated with small cobalt nanoparticles under visible light. J. Catal. 2018, 357, 147-53.
154. Tayyab, M.; Liu, Y.; Min, S.; et al. Simultaneous hydrogen production with the selective oxidation of benzyl alcohol to benzaldehyde by a noble-metal-free photocatalyst VC/CdS nanowires. Chin. J. Catal. 2022, 43, 1165-75.
155. Zhang, Q.; Du, C.; Zhao, Q.; Zhou, C.; Yang, S. Visible light-driven the splitting of ethanol into hydrogen and acetaldehyde catalyzed by fibrous AgNPs/CdS hybrids at room temperature. J. Taiwan. Inst. Chem. E. 2019, 102, 182-9.
156. Fu, X.; Leung, D. Y.; Wang, X.; Xue, W.; Fu, X. Photocatalytic reforming of ethanol to H2 and CH4 over ZnSn(OH)6 nanocubes. Int. J. Hydrogen. Energy. 2011, 36, 1524-30.
157. Liu, M.; Qiao, L.; Dong, B.; et al. Photocatalytic coproduction of H2 and industrial chemical over MOF-derived direct Z-scheme heterostructure. Appl. Catal. B. Environ. 2020, 273, 119066.
158. Zhang, F.; Li, J.; Wang, H.; et al. Realizing synergistic effect of electronic modulation and nanostructure engineering over graphitic carbon nitride for highly efficient visible-light H2 production coupled with benzyl alcohol oxidation. Appl. Catal. B. Environ. 2020, 269, 118772.
159. Lin, Q.; Li, Y.; Qi, M.; et al. Photoredox dual reaction for selective alcohol oxidation and hydrogen evolution over nickel surface-modified ZnIn2S4. Appl. Catal. B. Environ. 2020, 271, 118946.
Cite This Article

How to Cite
Download Citation
Export Citation File:
Type of Import
Tips on Downloading Citation
Citation Manager File Format
Type of Import
Direct Import: When the Direct Import option is selected (the default state), a dialogue box will give you the option to Save or Open the downloaded citation data. Choosing Open will either launch your citation manager or give you a choice of applications with which to use the metadata. The Save option saves the file locally for later use.
Indirect Import: When the Indirect Import option is selected, the metadata is displayed and may be copied and pasted as needed.
About This Article
Copyright
Author Biographies
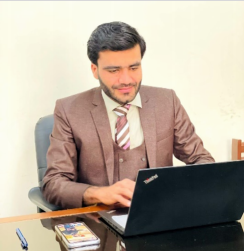
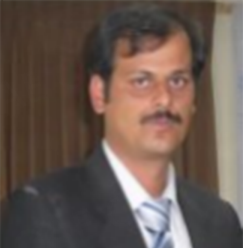
Dr. Riaz worked as an Assistant Professor of Physics at the University of Gujrat, Gujrat, Pakistan, from April 1, 2015, to September 6, 2021. He then joined the University of Okara, Pakistan, on September 6, 2021, as an Associate Professor of Physics, where he continues to serve in this prestigious institution.
Dr. Riaz has published more than 189 international research papers, with an h-Index of 53 and approximately 7866 citations (source: Google Scholar). Additionally, he has edited seven book chapters in well-recognized books published by Springer and Elsevier. His book, “Photocatalysis and Nanomaterials in Chemistry: Mechanistic & Experimental Approaches”, was recently published by Springer Nature.
Dr. Riaz has completed two SRGP research projects of HEC as PI and Co-PI. He also completed one NRPU, HEC project worth Rs. 4.57 million in 2020 at the University of Gujrat, Gujrat. Dr. Riaz received research productivity awards from the Pakistan Council of Science and Technology in 2013, 2014, 2015 and 2016.
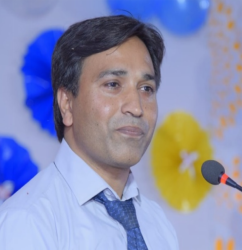
After completing his PhD, Dr. Tanveer served at Punjab University, Lahore, Pakistan, for one year (2015-2016) before joining the University of Lahore, Pakistan. At UOL, he served as the HOD of Physics at UOL Gujrat Campus for three years (2016-2019). On October 16, 2019, he joined the University of Gujrat (UOG), Pakistan, as an Assistant Professor on the Tenure Track System (TTS), where he has served for five years. He has recently been selected as the HOD of Physics at UOG Mandi Campus, Pakistan.
Dr. Tanveer has published more than 110 research manuscripts in well-reputed peer-reviewed journals, with an accumulated impact factor of 350. He has been recognized as one of the top 2% scientists globally in 2024, as listed by Stanford University and Elsevier.
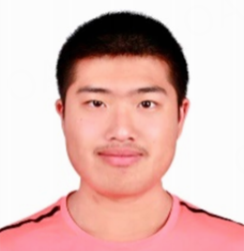
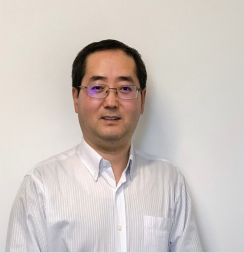
Data & Comments
Data
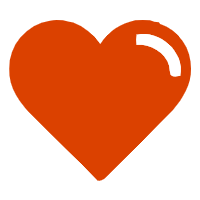
Comments
Comments must be written in English. Spam, offensive content, impersonation, and private information will not be permitted. If any comment is reported and identified as inappropriate content by OAE staff, the comment will be removed without notice. If you have any queries or need any help, please contact us at [email protected].