In situ synthesis of nanosized ZSM-12 zeolite isomorphously substituted by gallium for the n-hexadecane hydroisomerization
Abstract
The ZSM-12 zeolite has attracted attention as the promising acid component of bifunctional catalysts for the
Keywords
INTRODUCTION
Owing to the reduction in non-renewable fossil energy and increasing environmental awareness, the development of high-quality alternative energy sources has attracted worldwide attention in recent decades[1-5]. Second-generation biodiesel has become a potential environmentally friendly fuel because of its abundant and renewable raw materials, which are free of olefins, aromatics, sulfides, and nitrides[6,7]. To modify the low-temperature flow property of second-generation biodiesel produced from Fischer-Tropsch synthesis wax or vegetable oil, the hydroisomerization of linear n-alkanes with long carbon chains has become an essential process[8-10]. Currently, the most widely employed catalysts for n-alkane hydroisomerization are bifunctional catalysts containing metal sites with dehydrogenation and hydrogenation functions and Brønsted acid sites with isomerization functions[11,12]. Accordingly, it is essential to develop effective bifunctional catalysts to improve the hydroisomerization performance of
ZSM-12 zeolite with a 12-membered-ring opening has become a promising acid support for providing Brønsted acid sites because of its one-dimensional channels and large opening size of main channels
In recent years, the synthesis of metal-incorporated zeolites containing Zn, Fe and Ga in the framework has been recognized as a promising method to decrease the crystal size and weaken the acidity of synthesized hierarchical zeolites simultaneously[23,24]. Because of the larger atomic radius, the substitution of Ga and Fe into the framework is more difficult, and the H+ dissociation of Ga-OH-Si and Fe-OH-Si groups is also weaker than that of the Al-OH-Si group. Accordingly, Ga- or Fe-containing zeolites generally have weaker Brønsted acidity than conventional silica aluminate zeolites[25,26]. To synthesize Ga-incorporated zeolite, the conventional strategies are ion exchange and wet impregnation methods. However, the Ga amount introduced into the ion exchange sites of the ZSM-12 zeolite is generally limited because of the high Si/Al ratios[27]. Additionally, for the preparation of Ga-incorporated zeolites by the incipient wet impregnation method, most of the Ga species are still located on the external surface or enriched at the micropores opening of the zeolite and finally become aggregated Ga oxides after the preparation of the bifunctional catalysts[28].
In addition to ion exchange and impregnation strategies, isomorphous substitution has been developed as a promising method for synthesizing Ga-incorporated zeolites due to its simple synthesis process and wide range of adjustable acidity[29,30]. Yang et al. synthesized Ga-substituted HZM-5 zeolites via a hydrothermal process by adding different amounts of Ga(NO3)3 to the initial gel and studied their catalytic performances for the in situ reforming of lignite pyrolysis volatiles to enrich light aromatics. The catalytic test results suggest that the benzene yield over Ga-substituted HZM-5 zeolite is almost two times greater than that over conventional HZSM-5 zeolite[31]. Liu et al. synthesized Ga-ZSM-22 zeolites as supports for the n-dodecane hydroisomerization. They found that the selectivity for iso-dodecanes increased from 47.01% to 78.19% after Ga substitution since the synthesized Ga-substituted ZSM-22 zeolites demonstrated reduced Brønsted acid strength and acid density[32]. Although the influences of Ga substitution on the catalytic performance of ZSM-5 and ZSM-22 zeolites have been investigated in the previously reported works, there have been few reports on Ga-modified ZSM-12 zeolites for the long-chain n-alkane hydroisomerization. Simultaneously, during the in situ isomorphic substitution process, the competition for the entrance into the zeolite framework between Ga and Al usually leads to the formation of abundant non-framework species that decrease the crystallinity or block micropores of synthesized zeolites, resulting in decrease of the selectivity for branched isomers. Therefore, the Ga isomorphic substitution of ZSM-12 zeolite remains challenging for the synthesis of highly effective hierarchical zeolites for hydroisomerization.
Herein, nanosized GaZSM-12 silicogalliate zeolite was synthesized in situ via a hydrothermal method. For comparison, [Ga,Al]ZSM-12 samples (Ga/Al molar ratio of 1:1) partially isomorphously substituted with gallium and ZSM-12 silica aluminate zeolite were also synthesized. Furthermore, bifunctional catalysts for
EXPERIMENTAL
Materials
Sodium aluminate [chemically pure (CP), Sinopharm Chemical Reagent, China], silica sol (SiO2 32.3%, Qingdao Yuminyuan, China), sodium hydroxide [analytical reagent (AR), Tianjin Tianda Chemical Reagent, China], tetraethyl ammonium bromide (TEABr, AR, Sinopharm Chemical Reagent, China) and gallium oxide (99.99%, Tianjin Guangfu Fine Chemical Research Institute, China) were used as aluminum, silica, alkaline, template and gallium sources to synthesize ZSM-12 and Ga isomorphously substituted GaZSM-12 samples, respectively. Pseudoboehmite (71%, Shandong Aluminum, China) and 5% Pd(NO3)2 aqueous solution (Shanxi Kaida, China) were used to prepare Pd/Al2O3 and further bifunctional catalysts.
Synthesis of Z12, [Ga,Al]Z12 and GaZ12 samples partially and completely isomorphously substituted by gallium
The ZSM-12 sample was synthesized via a hydrothermal crystallization method. The NaOH, NaAlO2 and TEABr were first dissolved in deionized water, followed by the addition of silica sol under vigorous stirring to obtain the initial gel with a molar composition of 130SiO2:1.0Al2O3:4.225Na2O:16.25TEA+:1690H2O. The obtained initial gel was then moved into a Teflon-lined autoclave, followed by crystallization at 160 °C for three days. After crystallization, the sample was centrifuged, washed, dried for 12 h at 110 °C, and calcined for 6 h at 650 °C in air with a heating rate of 10 °C/min to obtain a Na-type ZSM-12 sample. Finally, the Na-type ZSM-12 sample was mixed with 0.5 mol/L NH4NO3 solution at a liquid/solid ratio of 30:1 for 3 h, followed by drying and calcination to obtain the H-type ZSM-12 sample, which is denoted as Z12.
The nanosized Ga partially isomorphously substituted ZSM-12 sample was synthesized by the same process except that the initial gel was prepared with a molar composition of 130SiO2:0.5Al2O3:0.5Ga2O3:
To synthesize the nanosized Ga completely isomorphously substituted ZSM-12 sample, the same method as the [Ga,Al]Z12 sample was employed except that the initial gel was prepared with a molar composition of 130SiO2:1Ga2O3:4.225Na2O:16.25TEA+:1690H2O. The synthesized sample was named GaZ12.
The preparation of bifunctional catalysts
To prepare the bifunctional catalysts, the pseudoboehmite was first calcined in air at 600 °C for 5 h with a heating rate of 10 °C/min to obtain the γ-Al2O3 support. Then, take 0.6 mL of the 5% Pd(NO3)2 aqueous solution and dilute it to 5.5 mL. After that, the 5 g obtained γ-Al2O3 was wetness-impregnated in the diluted Pd(NO3)2 aqueous solution to achieve the Pd loading of the final sample of 0.6 wt.%. The impregnated sample was then dried for 12 h at 110 °C and calcined for 4 h at 600 °C in air with a heating rate of 10 °C/min to obtain the 0.6Pd/A sample. Then, the 0.6Pd/A sample was mixed with the same mass of the Z12, [Ga,Al]Z12 and GaZ12 samples, crushed into 20-40 mesh particles and reduced in a fixed bed reactor at
Characterizations
All zeolite samples were analyzed using the X-ray powder diffractometer (XRD) with a Bruker D8 Advance diffractometer, which has a Cu Kα X-ray source (λ = 1.5406 Å) with the tube voltage of 40 kV and the tube current of 20 mA. The scanning angle range of 2θ = 5°-55° and the relative crystallinity of all samples were obtained by calculating the area of the characteristic diffraction peak of 2θ. The textural properties of all samples were measured by Autosorb-iQ2 automatic specific surface and porosity analyzer. The high temperature (300 °C) vacuum pretreatment was carried out in the degassing station at 1.0 × 10-3 Pa for 12 h to remove impurities. After the pretreatment, the sample tube was then transferred to a workstation, and nitrogen was adsorbed to saturation at the temperature of liquid nitrogen, and then heated at a certain rate to record the signals of N2 adsorption and desorption. The SIGMA500 microscope was employed to investigate the morphology of all samples observed by scanning electron microscopy (SEM), and a JEM-F200 microscope was employed to investigate the morphology of synthesized ZSM-12 crystal and distribution of elements by transmission electron microscopy (TEM) at 200 kV. The 29Si, 27Al and 71Ga magic angle spinning nuclear magnetic resonance (MAS NMR) spectra were all acquired on a Bruker advanced 400M spectrometer by loading samples into a 4 mm ZrO2 rotor at room temperature and a magnetic field of 9.4 T and rotating at 10, 10 and 12 kHz, respectively. The framework Si/(Al+Ga)F ratio was calculated by Equation (1):
in which I represents the resonance peak area in 29Si MAS NMR while n indicates the number of Al atoms connected to Si. The acidity of all samples was characterized by temperature-programmed desorption (TPD) with NH3 as a probe molecule. The sample (20-40 mesh) was first heated to 600 °C for 1 h in argon atmosphere, then cooled to 120 °C. The Fourier Transform Infrared Spectrometer (FT-IR) spectra of all synthesized samples were measured using a PerkinElmer PE-100 Spectrum Frontier FT-IR spectrometer. Inductively coupled plasma (ICP) apparatus (Inductively Coupled Plasma, PerkinElmer 200) was applied for analysis of trace elements in the synthesized ZSM-22 samples. Prior to the characterization, 0.025 g of the ZSM-22 sample was mixed with 1.5 mL of 40 wt.% HF, which was added into a high-density polyethylene (HDPE) bottle. After that, 0.125mL of HNO3 (45 wt.%), 0.375mL of HCl (45 wt.%) and 5 mL of distilled water are added to dissolve the ZSM-22 sample, followed by the addition of 12 mL saturated H3BO3 solution to avoid the Si evaporation. The solution is then diluted to 30 mL to be analyzed by ICP at last. The X-ray photoelectron spectroscopy (XPS) measurements were conducted on a Kratos, ULTRA AXIS DLD with an Al Ka achromatic X-ray source (1,486.6 eV) for all reduced catalysts. The NH3 was injected and adsorbed for 30 min until saturated followed by the physical purging of argon. Finally, a programmed temperature increase (10 °C/min) to 650 °C was applied for NH3 desorption, and a signal was recorded using a thermal conductivity detector (TCD). The Brønsted acidity of all samples was further characterized using a PerkinElmer PE-100 Spectrum Frontier FT-IR spectrometer. After vacuum pretreatment at 350 °C at
Catalytic tests for n-hexadecane hydroisomerization
All catalysts were tested for n-hexadecane hydroisomerization in a fixed-bed reactor. Before the reaction, 1 g of the calcined catalyst is placed in a constant temperature area of the tube and introduced into the hydrogen at 400 °C for 1 h reduction. The test conditions were 2.0 MPa pressure, 3.7 h-1 weight hourly space velocity (WHSV) and 500 H2/n-hexadecane volume ratio. The product analysis was measured using a gas chromatograph (Agilent 7820) with a flame ionization detector (FID) and an HP-1 (60 m × 250 μm × 1 mm) capillary column.
The conversion of n-hexadecane, the selectivity of iso-hexadecane and the yield of iso-hexadecane were calculated using Equations (2-4):
The nas value, representative of the average acid steps of one n-hexadecane molecule contacted during the hydroisomerization between two Pd sites, was calculated according to the production distribution of
in which the proportions of mono-branched iso-hexadecane, multi-branched iso-hexadecane and cracking products in the process of hydroisomerization are represented as “Mono”, “Multi” and “Cracked”, respectively. The NC value represented the average number of molecules formed after the cleavage of each
RESULTS AND DISCUSSION
Structure and morphology of the Z12, [Ga,Al]Z12 and GaZ12 samples
The XRD patterns of the Z12, [Ga,Al]Z12 and GaZ12 samples are shown in Figure 1. For all the samples, only the characteristic diffraction peaks ascribed to typical MTW structure are observed at approximately 7.6°, 8.8°, 20.9°, 22.9° and 23.3°, suggesting that the three samples are all pure [Figure 1A]. Moreover, the 2θ of the diffraction peaks for the three samples decreases as more Ga atoms are substituted in the samples [Figure 1B], which suggests that isomorphous substitution by Ga leads to a larger interplanar spacing. The crystal cell volume data listed in Table 1 show that the unit cell parameters and volume of the three samples both increase in the order of Z12 < [Ga,Al]Z12 < GaZ12, which originates from the longer Ga–O bond compared with the Al–O bonds for the GaZ12 and [Ga,Al]Z12 samples, with framework Al atoms being completely or partially isomorphously substituted by Ga atoms, respectively[33]. In addition to the unit cell size, the half-width of the diffraction peaks of the [Ga,Al]Z12 and GaZ12 samples also increases. Based on the Scherrer equation, the calculated average crystal size of the three samples demonstrates that Ga substitution effectively reduces the crystal size of synthesized ZSM-12 samples [Table 1].
Figure 1. (A) XRD patterns of the Z12, [Ga,Al]Z12 and GaZ12 samples; and (B) locally enlarged 2θ patterns between 20° and 25° for the three samples. XRD: X-ray powder diffractometer.
The unit cell parameters and mean average sizes of the Z12, [Ga,Al]Z12 and GaZ12 samples based on the XRD patterns
Samples | Unit cell parameters (Å) | Unit cell volume (Å3) | Crystal sizea (nm) | ||
A | b | c | |||
Z12 | 25.105 | 5.046 | 24.361 | 3,085.96 | 42 |
[Ga,Al]Z12 | 25.226 | 5.047 | 24.395 | 3,106.05 | 36 |
GaZ12 | 25.311 | 5.064 | 24.454 | 3,134.08 | 24 |
Figure 2 shows SEM and TEM images of the Z12 [Figure 2A-C], [Ga,Al]Z12 [Figure 2D-F] and GaZ12 samples [Figure 2G-I]. The Z12 sample shows a morphology of spherical aggregates of about 1 μm, which are composed of nanocrystals of approximately 40-50 nm [Figure 2A and B]. For the partially and completely Ga isomorphous substituted [Ga,Al]Z12 and GaZ12 samples, the aggregates change to ellipsoidal shapes. Additionally, the sizes of the aggregates and single grains of [Ga,Al]Z12 decrease to 800-900 and 30-40 nm, respectively [Figure 2D and E]. For the GaZ12 sample, the aggregates and single grains further decrease to 600-700 and 20-30 nm, respectively [Figure 2G and H]. These phenomena are consistent with the calculated results based on the Scherrer equation, demonstrating that Ga isomorphous substitution is an effective method for reducing the size of synthesized ZSM-12 samples, which will be beneficial for improving the mass transfer properties of zeolite channels. Moreover, Si [Figure 2C(i), F(i) and I(i)], Al
Figure 2. (A, D, G) SEM images, (B, E, H) TEM images, [C(i), F(i), I(i)] Si EDS mapping and [C(ii), F(ii), I(ii)] Al or/and Ga EDS mapping of the (A-C) Z12, (D-F) [Ga,Al]Z12 and (G-I) GaZ12 samples. SEM: Scanning electron microscopy; TEM: transmission electron microscopy; EDS: energy dispersive X-ray spectrometry.
For the N2 adsorption-desorption isotherms of the Z12, [Ga,Al]Z12 and GaZ12 samples shown in Supplementary Figure 1, a steep adsorption curve at P/P0 < 0.01, and the hysteresis loop observed at P/P0 between 0.5-0.9 suggest the existence of micropores and intercrystal mesopores for all the samples. According to the isotherms, the surface area and pore volume of the samples were calculated and are shown in Table 2. Compared with those of Z12, the external surface area and mesopore volume of the [Ga,Al]Z12 and GaZ12 samples are both evidently larger, indicating a decrease in the crystal size and an increase in the mesoporosity.
The textural properties of the Z12, [Ga,Al]Z12 and GaZ12 samples
Samples | Surface area (m2/g) | Pore volume (cm3/g) | ||||
BET | Microporea | External | Totalb | Microporea | Mesopore | |
Z12 | 342 | 300 | 42 | 0.226 | 0.118 | 0.108 |
[Ga,Al]Z12 | 337 | 275 | 62 | 0.265 | 0.109 | 0.156 |
GaZ12 | 334 | 258 | 76 | 0.255 | 0.103 | 0.152 |
The compositions of the Z12, [Ga,Al]Z12 and GaZ12 samples
27Al, 71Ga, and 29Si MAS NMR were conducted to investigate the chemical environment of the synthesized Z12, [Ga,Al]Z12 and GaZ12 samples. As shown in Figure 3A and B, strong peaks at a chemical shift of
Figure 3. 27Al MAS NMR spectra of the (A) Z12 and (B) [Ga,Al]Z12 samples and 71Ga MAS NMR spectra of the (C) [Ga,Al]Z12 and (D) GaZ12 samples. MAS: Magic angle spinning; NMR: nuclear magnetic resonance.
In the 29Si MAS NMR spectra of the three samples [Supplementary Figure 3], four resonance peaks at chemical shifts of approximately -101, -105, -109 and -113 ppm are observed, which correspond to Si(3SiOH), Si(3Si1Al), and Si(4Si0Al) species in two different environments, respectively. The framework Si/(Al+Ga)FW ratios of the three samples were further calculated. Although the Si/(Al+Ga) ratios in the initial gel are the same [Table 3], the calculated Si/(Al+Ga)FW ratios of the three samples increase in the order of Z12 (63) < [Ga,Al]Z12 (70) < GaZ12 (89), suggesting that the substitution of Ga into the ZSM-12 framework is more difficult than that of Al.
Chemical components of the Z12, [Ga,Al]Z12 and GaZ12 samples
Samples | Chemical compositions | |||
Si/(Al+Ga)Gel | Si/(Al+Ga)Bulka | Si/(Al+Ga)FWb | Si/(Al+Ga)Surfc | |
Z12 | 65 | 62 | 63 | 27 |
[Ga,Al]Z12 | 65 | 67 | 70 | 17 |
GaZ12 | 65 | 86 | 89 | 43 |
In addition to the MAS NMR measurements, ICP and XPS characterizations were also conducted for the Z12, [Ga,Al]Z12 and GaZ12 samples to further determine the bulk Si/(Al+Ga)Bulk and the surface
Furthermore, from the XPS spectra of the [Ga,Al]Z12 and GaZ12 samples [Figure 4], the peak at a binding energy of 1,118.5 eV is attributed to the Ga2O3 species located at the external surface, while the peak at 1,119.4 eV is ascribed to the Ga species located in the micropores or that interact strongly with the negatively charged framework of the synthesized samples[34]. The existence of the two kinds of Ga species at different locations was also proven by H2-TPR measurements. From Supplementary Figure 4, two reduction peaks exist between 230 and 440 °C for the [Ga,Al]Z12 and GaZ12 samples, which are not found for the Z12 sample. The reduction peak at lower temperatures originates from the reduction of Ga2O3 species on the surface of synthesized crystals, and the peak observed at higher temperatures corresponds to the reduction of Ga species located in the channel or that interact strongly with the negatively charged sample framework.
Acidity of the Z12, [Ga,Al]Z12 and GaZ12 samples
The acidity of the Z12, [Ga,Al]Z12 and GaZ12 samples was characterized by NH3-TPD. For the three samples, two NH3 desorption peaks at varying temperatures are found in the NH3-TPD curves, which originate from the desorption of NH3 from weak and strong acid sites of the samples, respectively
The desorption temperatures of NH3 and acid density of the Z12, [Ga,Al]Z12 and GaZ12 samples
Samples | Twda (°C) | Tsdb (°C) | Brønsted acid density (μmol·g-1) | Lewis acid density (μmol·g-1) | |
Strong | Total | Total | |||
Z12 | 168 | 362 | 26.7 | 31.2 | 10.2 |
[Ga,Al]Z12 | 168 | 338 | 24.4 | 29.3 | 15.8 |
GaZ12 | 168 | 313 | 20.3 | 25.3 | 23.8 |
Since Brønsted acid sites are responsible for isomerization and cracking, quantitatively determining the Brønsted acid density from the Py-IR is more important. As shown in Supplementary Figure 6, two characteristic adsorption peaks appear at ~1,455 and ~1,545 cm-1 in the Py-IR of Z12, [Ga,Al]Z12 and GaZ12 samples, which are attributed to Lewis and Brønsted acid sites, respectively. Compared with those of the Z12 sample, the total and strong Brønsted acid densities of the [Ga,Al]Z12 and GaZ12 samples both decrease as the Ga amount in the samples increases because of the decreasing total amount of framework Ga and Al atoms [Table 4]. As a result, fewer frameworks bridging hydroxyl groups Si-O(H+)-Ga and
Characteristics of the prepared bifunctional catalysts
According to previous reports, bifunctional catalysts with appropriate metal-acid proximity by locating metal sites on the Al2O3 instead of zeolite generally demonstrate improved catalytic performance for the
Characteristics of 0.3Pd/A-Z12, 0.3Pd/A-[Ga,Al]Z12 and 0.3Pd/A-GaZ12 bifunctional catalysts
Samples | Brønsted acid density (μmol·g-1) | DPd (%)a | CPd (μmol·g-1)b | CPd/CH+c | |
Strong | Total | ||||
0.3Pd/Z12-A | 8.9 | 10.5 | 27.2 | 7.7 | 0.733 |
0.3Pd/A-Z12 | 13.4 | 15.6 | 39.4 | 11.1 | 0.712 |
0.3Pd/A-[Ga,Al]Z12 | 12.2 | 14.7 | 39.4 | 11.1 | 0.756 |
0.3Pd/A-GaZ12 | 10.2 | 12.7 | 39.4 | 11.1 | 0.875 |
n-hexadecane hydroisomerization over bifunctional catalysts
Figure 5A and B shows that, for the 0.3Pd/Z12-A, 0.3Pd/A-Z12, 0.3Pd/A-[Ga,Al]Z12 and 0.3Pd/A-GaZ12 catalysts, the n-hexadecane conversion all demonstrate typical S-shaped curves as reaction temperature increases. Compared with the 0.3Pd/Z12-A catalyst with Pd deposited directly on ZSM-12 zeolite and the closest metal-acid proximity (metal-acid distance on atomic scale), the 0.3Pd/A-Z12 catalyst with Pd loaded on the γ-Al2O3 exhibited improved catalytic activity due to the higher Brønsted acid density [Table 5]. For the 0.3Pd/A-Z12, 0.3Pd/A-[Ga,Al]Z12 and 0.3Pd/A-GaZ12 catalysts (metal-acid distance on micrometer scale) with the same metal-acid proximity, the n-hexadecane conversion decreased at the same temperature as the Ga amount increased because of their weaker Brønsted acidity after isomorphous substitution by Ga [Figure 5B].
Figure 5. (A and B) The n-hexadecane conversion; and (C and D) the iso-hexadecane yield over different catalysts. The test conditions were 2.0 MPa pressure, 3.7 h-1 space velocity (WHSV) and 500 H2/n-hexadecane volume ratio. WHSV: Weight hourly space velocity.
The iso-hexadecane yields over the four catalysts are shown in Figure 5C and D. The 0.3Pd/Z12-A catalyst demonstrates a maximum iso-hexadecane yield of 69.9%, while for the 0.3Pd/A-Z12 catalyst, the maximum iso-hexadecane yield increases to 74.3%. This result indicates that appropriate metal-acid proximity for the 0.3Pd/A-Z12 catalyst is evidently beneficial for the promotion of the iso-hexadecane yield. For the 0.3Pd/Z12-A catalyst, the intermediates generated on Pd sites loaded on ZSM-12 zeolite then diffuse to the Brønsted acid sites on the ZSM-12 zeolite so that further isomerization and even cracking easily occur due to their limited diffusion in the micropore channel. However, for the 0.3Pd/A-Z12 catalyst, the intermediates are generated on Pd sites loaded on γ-Al2O3 and then diffuse to the Brønsted acid sites on the surface or at the opening of ZSM-12 zeolite instead of diffusing into the micropores, which can effectively limit the cracking of these intermediates due to the avoidance of diffusion into micropores[36]. As a result, the maximum iso-hexadecane yield over the 0.3Pd/A-Z12 catalyst was higher. Furthermore, the maximum iso-hexadecane yield over the 0.3Pd/A-[Ga,Al]Z12 catalyst increased to 77.9% [Figure 5D]. The 0.3Pd/A-GaZ12 catalyst demonstrated the maximum iso-hexadecane yield of 80.6%, which is much greater than that reported in previous works [Supplementary Table 3] and is 6.3% higher compared with the 0.3Pd/A-Z12 catalyst. Moreover, the nas values (the acid steps that the alkene intermediates undergo between two Pd sites) were calculated. The nas for the four catalysts decreased as 0.3Pd/Z12-A (1.81) > 0.3Pd/A-Z12 (1.62) > 0.3Pd/A-[Ga,Al]Z12 (1.49) > 0.3Pd/A-GaZ12 (1.38) [Supplementary Table 4]. Owing to the avoidance of diffusion of the intermediates into micropores, the nas value of 0.3Pd/A-Z12 is only 1.62, which leads to a higher maximum iso-hexadecane yield compared with the 0.3Pd/Z12-A catalyst. For the 0.3Pd/A-[Ga,Al]Z12 and 0.3Pd/A-GaZ12 catalysts, the increase in the number of substituted Ga atoms further reduces the Brønsted acid strength and density and effectively increases their CPd/CH+ ratios, which makes the metal-acid balance of the bifunctional catalysts more favorable and reveal lower nas during hydroisomerization. In addition, the crystal sizes of Ga-substituted ZSM-12 samples are also reduced, which causes greater mesoporosity and improved diffusion of alkene intermediates, resulting in effectively limiting the cracking of intermediates over the 0.3Pd/A-[Ga,Al]Z12 and 0.3Pd/A-GaZ12 catalysts, so that the maximum iso-hexadecane yield is promoted.
To further study the effects of Ga isomorphous substitution on the n-hexadecane hydroisomerization, the product distribution and the proportion of cracking products at the maximum yield of iso-hexadecane [Figure 6], as well as the product distribution in the kinetic control region [Supplementary Figure 8] over the catalysts, were investigated. From Supplementary Figure 8, when the conversion n-hexadecane is 9%~14%, the mono-branched iso-hexadecanes are the main products for the three catalysts. Meanwhile, when the conversion is higher (90%~93%), the main products are the multi-branched iso-hexadecanes for the three catalysts [Figure 6A]. As the Ga amount in the isomorphously substituted ZSM-12 zeolite rises, the quantity of multi-branched iso-hexadecanes also grows, while the distribution of cracking products decreases. In particular, for the 0.3Pd/A-GaZ12 catalyst, the multi-branched iso-hexadecanes distribution (64.6%) was the highest among the three catalysts. This result originates from the smaller grains and more openings of the 0.3Pd/A-GaZ12 catalyst, which can promote further isomerization to form multi-branched iso-hexadecanes. Simultaneously, its weaker acid strength also inhibits the further cracking of the multi-branched products. Therefore, the largest distribution of multi-branched iso-hexadecanes has been obtained for the 0.3Pd/A-GaZ12 catalyst. Furthermore, from the cracking product distribution shown in Figure 6B, the proportion of C3-C6 cracked products over the 0.3Pd/A-GaZ12 catalyst is evidently reduced, and the proportion of C8-C12 liquid hydrocarbons is greater. This phenomenon demonstrates that Ga isomorphous substitution can also suppress the secondary cracking owing to the reduced acid strength and increase in the CPd/CH+ ratio.
Figure 6. (A) Products distribution and (B) the proportion of cracking products at the maximum iso-hexadecane yield over 0.3Pd/A-Z12, 0.3Pd/A-[Ga,Al]Z12 and 0.3Pd/A-GaZ12 bifunctional catalysts. The test conditions were 2.0 MPa pressure, 3.7 h-1 space velocity (WHSV) and 500 H2/n-hexadecane volume ratio. WHSV: Weight hourly space velocity.
CONCLUSIONS
Herein, the effective method for in situ synthesis of [Ga,Al]Z12 and GaZ12 samples is established, in which framework Al atoms are partially or completely isomorphously substituted by Ga atoms. The characteristic results indicate that the Ga-substituted [Ga,Al]Z12 and GaZ12 samples have a greater mesoporosity and weaker Brønsted acidity compared with the Z12 silica aluminate sample. The 0.3Pd/A-Z12, 0.3Pd/A-[Ga,Al]Z12 and 0.3Pd/A-GaZ12 catalysts with appropriate metal-acid proximity are prepared by mixing 0.6Pd/A sample with 0.6 wt.% Pd loading on γ-Al2O3 and the corresponding ZSM-12 samples. These catalysts show higher iso-hexadecane yield for the n-hexadecane hydroisomerization in comparison with the 0.3Pd/Z12-A catalyst with 0.6 wt.% Pd directly deposited on ZSM-12 zeolite with closer metal-acid proximity. The enhanced mesoporosity, milder acidity and larger CPd/CH+ ratio of the 0.3Pd/A-[Ga,Al]Z12 and 0.3Pd/A-GaZ12 bifunctional catalysts are beneficial for improving the iso-hexadecane yield and distribution of multi-branched iso-hexadecane products due to the achievement of more favorable metal-acid balance and effectively suppressed cracking of iso-olefin intermediates. The 0.3Pd/A-GaZ12 catalyst reveals a maximum iso-hexadecane yield of 80.6% and a proportion of multi-branched iso-hexadecane of 64.6%. Therefore, this work demonstrates an applicable method for the preparation of effective bifunctional catalysts to produce second-generation biodiesel with iso-alkanes as the main components via the hydroisomerization of long-chain n-alkanes.
DECLARATIONS
Authors’ contributions
Writing-original draft, methodology, visualization, investigation, formal analysis, data curation: Lin H
Investigation: Xu C
Writing-original draft, visualization, software, data curation, validation, funding acquisition: Wang W
Conceptualization, methodology, resources, writing-reviewing and editing, funding acquisition, supervision, project administration: Wu W
Availability of data and materials
Since parts of the data of the article involves the cooperation with other companies, we cannot make all data applicable. However, if some researchers are interested in our work and want to reproduce it, they can contact us directly and we will provide the corresonding data.
Financial support and sponsorship
This work is supported by the National Natural Science Foundation of China (No. 22278115) and the National Key R&D Project, Intergovernmental International Science and Technology Innovation Cooperation Key Project (2018YFE0108800).
Conflicts of interest
All authors declared that there are no conflicts of interest.
Ethical approval and consent to participate
Not applicable.
Consent for publication
Not applicable.
Copyright
© The Author(s) 2024.
Supplementary Materials
REFERENCES
1. Fan L, Lyu Y, Fu J, et al. Metal particle size effects over the Ni/SAPO-11 bifunctional catalyst. Appl Surf Sci 2023;636:157736.
2. Ding S, Parlett CM, Fan X. Recent developments in multifunctional catalysts for fatty acid hydrodeoxygenation as a route towards biofuels. Mol Catal 2022;523:111492.
3. Chen LH, Sun MH, Wang Z, Yang W, Xie Z, Su BL. Hierarchically structured zeolites: from design to application. Chem Rev 2020;120:11194-294.
5. Yuan K, Jia X, Wang S, et al. Effect of crystal size of ZSM-11 zeolite on the catalytic performance and reaction route in methanol to olefins. Chem Synth 2023;4:31.
6. Niu P, Liu P, Xi H, et al. Design and synthesis of Pt/ZSM-22 catalysts for selective formation of iso-Dodecane with branched chain at more central positions from n-Dodecane hydroisomerization. Appl Catal A Gen 2018;562:310-20.
7. Yang C, Wang W, Wang D, et al. The promotion effects of MoOx species in the highly effective NiMo/MgAl2O4 catalysts for the hydrodeoxygenation of methyl palmitate. J Environ Chem Eng 2022;10:107761.
8. Lyu Y, Yu Z, Yang Y, et al. Metal and acid sites instantaneously prepared over Ni/SAPO-11 bifunctional catalyst. J Catal 2019;374:208-16.
9. Tan Y, Zhao Y, Li H, et al. Inhibition of terminal C–C bonds cleavage drives high selectivity of n-alkane hydroisomerization. J Catal 2023;428:115144.
10. Xiong S, Sun J, Li H, Wang W, Wu W. The synthesis of hierarchical ZSM-22 zeolite with only the PHMB template for hydroisomerization of n-hexadecane. Micropor Mesopor Mat 2024;365:112895.
11. Wang W, Liu C, Wu W. Bifunctional catalysts for the hydroisomerization of n-alkanes: the effects of metal-acid balance and textural structure. Catal Sci Technol 2019;9:4162-87.
12. He L, Fu W, Li L, et al. Study of CA-treated ZSM-22 zeolite with enhanced catalytic performance in the hydroisomerization of long-chain n-dodecane. New J Chem 2021;45:2820-9.
13. Han Y, Yuan J, Xing M, et al. Shape selectivity of zeolite for hydroisomerization of long-chain alkanes. New J Chem 2023;47:1401-12.
14. Li S, Mezari B, Wu H, Kosinov N, Hensen EJ. ZSM-12 nanocrystals with tunable acidity directed by rigid diquats: synthesis and catalytic applications. Fuel 2023;333:126363.
15. Wang S, Wang C, Liu H, et al. Acceleration effect of sodium halide on zeolite crystallization: ZSM-12 as a case study. Micropor Mesopor Mat 2022;331:111652.
16. Lu X, Guo Y, Zhang Y, Ma R, Fu Y, Zhu W. Enhanced catalytic activity of Pt/H-ZSM-12 via alkaline post-treatment for the hydroisomerization of n-hexane. Micropor Mesopor Mat 2020;306:110459.
17. Liu J, Yang H, Song Z, Sun J, Guo X. Isomerization of n-dodecane for high selectivity of multibranched iso-dodecane over Pt/ZSM-22 - Y catalyst. Ind Eng Chem Res 2023;62:21112-9.
18. Guo K, Ma A, Wang Z, et al. Investigation of n-heptane hydroisomerization over alkali-acid-treated hierarchical Pt/ZSM-22 zeolites. New J Chem 2022;46:16752-63.
19. Zhang L, Liu N, Dai C, et al. Recent advances in shape selectivity of MFI zeolite and its effect on the catalytic performance. Chem Synth 2023;3:2.
20. Sun J, Xiong S, Wu Q, Wang W, Wu W. The preparation of nanosized Pd/ZSM-23 bifunctional catalysts for n-hexadecane hydroisomerization by employing PHMB as the growth modifier. Trans Tianjin Univ 2023;29:482-91.
21. Li X, Sun M, Rooke JC, Chen L, Su B. Synthesis and applications of hierarchically porous catalysts. Chinese J Catal 2013;34:22-47.
22. Sun J, Xiong S, Lin H, et al. The effects of growth modifiers on the catalytic performance of hierarchical Pd/ZSM-23 bifunctional catalysts for n-hexadecane hydroisomerization. Fuel 2023;347:128406.
23. Lari GM, Mondelli C, Pérez-ramı́rez J. Gas-phase oxidation of glycerol to dihydroxyacetone over tailored iron zeolites. ACS Catal 2015;5:1453-61.
24. Yabushita M, Kobayashi H, Osuga R, et al. Mechanochemical approach to preparation of MFI zeolites substituted isomorphously by both Al and Fe as durable catalysts for the dimethyl ether to olefin reaction. Ind Eng Chem Res 2021;60:2079-88.
25. Liu S, Ren J, Zhu S, et al. Synthesis and characterization of the Fe-substituted ZSM-22 zeolite catalyst with high n-dodecane isomerization performance. J Catal 2015;330:485-96.
26. Geerts L, Ramachandran RK, Dendooven J, et al. Creation of gallium acid and platinum metal sites in bifunctional zeolite hydroisomerization and hydrocracking catalysts by atomic layer deposition. Catal Sci Technol 2020;10:1778-88.
27. Al-yassir N, Akhtar MN, Al-khattaf S. Physicochemical properties and catalytic performance of galloaluminosilicate in aromatization of lower alkanes: a comparative study with Ga/HZSM-5. J Porous Mater 2012;19:943-60.
28. Su X, Wang G, Bai X, et al. Synthesis of nanosized HZSM-5 zeolites isomorphously substituted by gallium and their catalytic performance in the aromatization. Chem Eng J 2016;293:365-75.
29. Shang S, Li W, Zhou A, et al. Fe-substituted Pt/HZSM-48 for superior selectivity of i-C12 in n-dodecane hydroisomerization. Ind Eng Chem Res 2022;61:1056-65.
30. Dai X, Li L, Cheng Y, et al. In-situ synthesis of gallium-containing SAPO-11 molecular sieves and superior catalytic performance of their NiWS supported catalysts for hydroisomerization of n-hexadecane. Eur J Inorg Chem 2023;26:e202200557.
31. Yang Z, Cao J, Zhu C, et al. In situ reforming of lignite pyrolysis volatiles for enriching light aromatics over Ga substituted HZSM-5. Chem Eng Sci 2022;248:117235.
32. Liu S, He Y, Zhang H, et al. Design and synthesis of Ga-doped ZSM-22 zeolites as highly selective and stable catalysts for n-dodecane isomerization. Catal Sci Technol 2019;9:2812-27.
33. Vieira LH, Carvalho KT, Urquieta-gonzález EA, Pulcinelli SH, Santilli CV, Martins L. Effects of crystal size, acidity, and synthesis procedure on the catalytic performance of gallium and aluminum MFI zeolites in glycerol dehydration. J Mol Catal A Chem 2016;422:148-57.
34. Su X, Fang Y, Gao P, et al. In-situ microwave synthesis of nano-GaZSM-5 bifunctional catalysts with controllable location of active GaO+ species for olefins aromatization. Micropor Mesopor Mat 2020;306:110388.
35. Zečević J, Vanbutsele G, de Jong KP, Martens JA. Nanoscale intimacy in bifunctional catalysts for selective conversion of hydrocarbons. Nature 2015;528:245-8.
36. Guo C, Wang W, Zhang Y, et al. Influences of the metal-acid proximity of Pd-SAPO-31 bifunctional catalysts for n-hexadecane hydroisomerization. Fuel Process Technol 2021;214:106717.
37. Wang D, Liu J, Cheng X, et al. Trace Pt clusters dispersed on SAPO-11 promoting the synergy of metal sites with acid sites for high-effective hydroisomerization of n-alkanes. Small Methods 2019;3:1800510.
38. Thybaut JW, Marin GB. Chapter two - Multiscale aspects in hydrocracking: from reaction mechanism over catalysts to kinetics and industrial application. Adv Catal 2016;59:109-238.
Cite This Article
How to Cite
Lin, H.; Xu C.; Wang W.; Wu W. In situ synthesis of nanosized ZSM-12 zeolite isomorphously substituted by gallium for the n-hexadecane hydroisomerization. Chem. Synth. 2024, 4, 49. http://dx.doi.org/10.20517/cs.2024.40
Download Citation
Export Citation File:
Type of Import
Tips on Downloading Citation
Citation Manager File Format
Type of Import
Direct Import: When the Direct Import option is selected (the default state), a dialogue box will give you the option to Save or Open the downloaded citation data. Choosing Open will either launch your citation manager or give you a choice of applications with which to use the metadata. The Save option saves the file locally for later use.
Indirect Import: When the Indirect Import option is selected, the metadata is displayed and may be copied and pasted as needed.
About This Article
Special Issue
Copyright
Data & Comments
Data
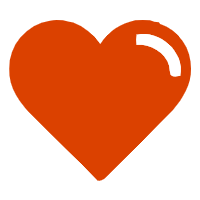
Comments
Comments must be written in English. Spam, offensive content, impersonation, and private information will not be permitted. If any comment is reported and identified as inappropriate content by OAE staff, the comment will be removed without notice. If you have any queries or need any help, please contact us at support@oaepublish.com.