Solid-state NMR of active sites in TiO2 photocatalysis: a critical review
Abstract
Titanium dioxide (TiO2) is one of the optimal semiconductor metal oxide photocatalysts with a wide range of application fields, such as heterogeneous catalysis, energy science, and environmental science. Solid-state nuclear magnetic resonance (NMR) spectroscopy is a powerful tool for characterizing both structure and dynamics at an atomic-molecular level in heterogeneous catalysts. In this review, we first provide a brief discussion on the progress in investigating the structures of titanium and oxygen in bulk and on the surface of TiO2 by using various solid-state NMR techniques. Advances in the understanding of electronic structure and properties of TiO2 with distinct surface features, including various crystal facets and heteroatomic adsorption by chemical probe-assisted NMR techniques, are secondly presented. The solid-state NMR characterization of heteroatom active sites (such as 13C, 15N, 11B, 27Al) and their function in TiO2 photocatalysts is described in detail. Finally, a critical discourse assesses the current limitations and prospects of solid-state NMR in its application to the optimization and design of advanced TiO2 photocatalysts.
Keywords
INTRODUCTION
Titanium dioxide (TiO2) has emerged as a leading candidate in the field of photocatalysis owing to its unique confluence of desirable attributes: exceptional optical and electronic properties, robust thermal and chemical stability, environmental benignity, and economic viability. This advantageous combination has propelled TiO2 to the forefront of research in diverse applications, including solar energy harvesting[1-3], photocatalytic hydrogen generation[4-6], CO2 conversion[7-9], and organic pollutant degradation[10,11]. Since 2000, the statistical analysis reported that publications on TiO2 photocatalysis crossed 10,000 per year, suggesting that this field has been intensively interested in research and development.
TiO2 exists in three crystalline phases: anatase, rutile, and brookite, and the former two can be used as semiconductor photocatalysts. The structure of both rutile and anatase TiO2 consists of chains of [TiO6] units, where six O2- ions surround one Ti4+ ion to form an octahedron[12]. However, due to the differences of each [TiO6] octahedron distortion, the Ti–Ti and Ti–O distances, octahedron chain assemblies, the energy band structure and mass density between two crystalline phases of TiO2 are different. Besides the crystalline phases, the differences in crystallite size and specific surface area, crystalline plane and morphology also cause change of properties in TiO2 due to the subsequent change in structures and content of microscopic Ti and O sites on TiO2 surface. For example, using K-edge X-ray absorption near edge structure (XANES) techniques, Chen et al. found that compared to the octahedron Ti sites in TiO2 with big size (50 nm), severe distortion of the Ti site environment exists in TiO2 nanoparticles with small size (1.9 nm)[13]. Due to the truncation of the lattice, the distorted Ti sites should be a penta-coordinate square pyramidal geometry, located mainly on the nanoparticle surface, responsible for the chemisorption of organic molecules. It was also found that more distorted bond angles of bridging oxygens (O–Ti2) and more unsaturated Ti sites (pentacoordinated Ti4+) are present on the (001) facet compared to the (101) facet[14,15]. All that lead to more active bridging O centers, easier O2 adsorption, and higher surface energy on the (001) facet[16,17]. Yu et al. reported that the higher photocatalytic ability of TiO2 thinner films was due to lesser opacity and more surface active sites[18]. Therefore, these microscopic active sites on the TiO2 surface should play a crucial role in improving photocatalytic activities.
The semiconductors-based photocatalytic reactions should involve three steps [Figure 1][6,12,19-21]: Firstly, the electron-hole pairs (carriers) are excited by photons with an energy more than the band gap of TiO2. Secondly, the photogenerated carriers separate or recombine during migration. Finally, the photogenerated carriers react with surface-adsorbed molecules through active sites on the TiO2 surface. As well known, owing to the wide band gap (rutile of 3.0 eV, and anatase of 3.2 eV), ultraviolet (UV) radiation is a prerequisite to facilitating the formation of the carriers during the photocatalytic reaction. Since ca. 50% of the solar radiation on earth is in the visible (vis) region, UV light makes up only ca. 5% of the natural light spectrum; improving the absorption of solar light by TiO2 has become one of the most urgent tasks in photocatalytic research and development. To solve this problem, some common and promising modification approaches, such as heteroatoms (ions) doping[1,22-32] and loading[1,19,32-41], have been utilized to narrow the band gap and enhance the separation efficiency of photogenerated carriers. Additionally, the reaction of photogenerated carriers on the TiO2 surface is also crucial in photocatalysis, in which the photogenerated carrier transfers to surface active sites to form active intermediates, and then the active intermediates react with surface molecules[42-48]. For example, surface hydroxyl (OH)/oxygen (O) sites and adsorbed H2O on TiO2 can trap photogenerated holes to form active paramagnetic intermediates (such as
Figure 1. Semiconductors-based photocatalytic mechanism. Reproduced with permission from[12]. Copyright 1995, American Chemical Society.
An in-depth understanding of active centers, including heteroatoms (ions) and surface active sites is the key to establishing structure-activity relationships, which can facilitate the rational design of highly efficient TiO2 photocatalysts. Thus, numerous techniques, such as high-resolution transmission electron microscopy (HRTEM), Raman spectroscopy, X-ray diffraction (XRD), X-ray photoelectron spectroscopy (XPS), Infrared (IR) spectroscopy, Electron paramagnetic resonance (EPR), solid-state nuclear magnetic resonance spectroscopy (NMR), etc., have been employed to study the structure of photocatalysts and the relevant reaction mechanisms. Generally, the crystal structure is determined by HRTEM, XRD, and Raman spectroscopy[51-56], and the electronic structures can be studied by X-ray photoelectron and X-ray absorption and emission spectroscopies[57-60]. EPR has been used to detect defect sites [Ti3+ and oxygen vacancy (Vo)], and surface paramagnetic species (O2•-, O- species, and •OH)[42,50,61], while IR spectroscopy has been used to detect surface OH and reaction intermediates of EPR and NMR silence[17,53,62].
Among them, solid-state NMR is a powerful tool for characterizing the structure of solid-state materials at the atomic-molecular level[63-70]. Although research on solid-state NMR techniques for photocatalyst active-site structures and photocatalytic reactions is rapidly increasing [Figure 2], the research interest in the application of solid-state NMR techniques is still relatively low compared to other techniques in the field of photocatalysis. To the best of our knowledge, this article presents the first examination of the application of solid-state NMR to TiO2 photocatalysis. Specifically, we conduct an overview of the advances made utilizing solid-state NMR techniques to analyze TiO2 photocatalysts. Through selected examples from the literature, we demonstrate how solid-state NMR has been utilized to reveal the atomic structures and interactions between various sites or components within TiO2. The review also discusses both the current limitations of solid-state NMR methodology in photocatalyst research and promising future directions for this technique.
TITANIUM AND OXYGEN ACTIVE SITES IN TiO2
The past few decades have witnessed a surge in efforts dedicated to unraveling the structural and electronic intricacies of TiO2, employing both experimental and theoretical approaches. However, the structure and coordination environments of Ti and O sites on the TiO2 surface are very different from those in bulk and thus remain poorly understood due to the complex surface structures. A wide variety of active sites exist on the TiO2 surface, including distorted Ti sites, low-coordinated Ti sites, bridging O sites, tri-coordinated O sites, etc. The distorted Ti sites, such as the double-bonded titanyl (Ti=O) groups on the (011) surface of reconstructed rutile TiO2, can promote the dissociation of part of adsorbed water[71,72]. The coordinatively unsaturated Ti atoms, including the penta-coordinated Ti sites (TiO5) on the surface and the tetra-coordinated Ti sites (TiO4) on the edge/corner, are important anchoring sites for the adsorption of targeted molecules (such as H2O, HCHO, and ethylene)[73-76] and for maintaining the high stability of the cocatalysts (such as Pd, Ru, Au, etc.)[77-79]. Theoretical calculations predicted that the bridging O sites (OTi2) of TiO2 can interact with the proton and facilitate the dissociation of H2O, which are closely related to catalytic mechanisms[80-82]. Furthermore, the coordinatively unsaturated Ti and O sites serve as active sites for charge carrier trapping and molecular adsorption during CO2 reduction and methanol conversion[83-85]. Thus, determining the structure and distribution of the Ti and O sites on TiO2 is a prerequisite for understanding the structure-property relationship.
Titanium active sites in TiO2
47/49Ti NMR can be utilized to detect the local environments of Ti sites in TiO2 photocatalysts[86-88]. However, the isotopes have a low natural abundance, with 47Ti at 7.28% and 49Ti at 5.51%. They also exhibit low Larmor frequencies, measuring 22.55 MHz at a magnetic field of 9.4 T. Additionally, the Ti atoms in TiO2 photocatalysts are quadrupole nuclei, with spin quantum numbers of I = 5/2 for 47Ti and I = 7/2 for 49Ti. These nuclei possess a relatively low gyromagnetic ratio and experience significant quadrupolar interactions, which lead to low sensitivity and resolution of these spectra due to the wide signal lineshape[89-91]. The 47/49Ti static NMR spectra were used to monitor the transition of the crystalline phases of brookite, anatase, and rutile in as prepared TiO2 at different annealing temperatures from 400 to 850 °C, and 47/49Ti NMR parameters were extracted from the spectral simulations of the corresponding components of bulk polycrystalline phases [Figure 3][92,93]. The size, crystallinity, and crystal phase of TiO2 can sensitively be reflected on the lineshape of the corresponding NMR signal. For example, the 49Ti NMR parameters, including chemical shift (δiso), quadrupole coupling constant (CQ), and asymmetry parameter (ƞ), for anatase were determined to be -67 ppm, 4.6 MHz, and 0.1, respectively, whereas for rutile the corresponding 49Ti NMR parameters are -15 ppm, 13.4 MHz, and 0.2, respectively. It was found that the narrow peak should be the 49Ti NMR signal in TiO2 bulk and the other relatively broad peak should be the 47Ti NMR signal in TiO2 bulk. With the increase of annealing temperature to 700 °C, the quadrupole linear patterns of 47Ti and 49Ti static NMR signals become more pronounced, indicating that the crystallinity of the anatase becomes better. With the further increase of annealing temperature, two NMR signals corresponding to 47Ti and 49Ti in rutile bulk increase gradually at the expense of the 47Ti and 49Ti NMR signals in anatase bulk, indicating the transition of crystal phase from anatase to rutile.
Figure 3. Experimental and simulated static Hahn-echo 47/49Ti NMR spectra of TiO2. The TiO2 nanoparticles were annealed before solid-state NMR experiments at variable temperatures. Reproduced with permission from[93]. Copyright 2001, American Chemical Society. NMR: Nuclear magnetic resonance.
However, a long-time (ca. 20 h) acquisition at 33.81 MHz using a CMX Infinity 600 spectrometer and large sample quantity (9.5 mm rotor) was necessary to ensure the signal-to-noise ratio of the spectra, and it is difficult to differentiate and assign the seriously overlapped resonances due to the large chemical shift anisotropy (CSA) and quadrupolar interactions. The advanced NMR techniques have been developed to optimize the signal excitation and acquisition. Bräuniger et al. use fast amplitude-modulated (FAM) radiofrequency (RF) pulse trains to enhance the sensitivity of the signal via transferring spin population from the satellite transitions to central transition[94]. In comparison with Hahn-echo acquisition, the intensity of the 47/49Ti central-transition line has increased by more than twice in the magic angle spinning (MAS) NMR spectra of TiO2.
As well known, the 47Ti and 49Ti isotopes exhibit almost identical Larmor frequencies and natural abundances[89]. Thus, to analyze the Ti sites of TiO2, it is important to distinguish the 47Ti and 49Ti signals in the NMR spectra. When these isotopes occur in sites with a significant electric field gradient (EFG), the different nuclear spin quantum numbers would result in varying effective RF fields for the central transition nutation frequencies. As such, Larsen et al. proposed isotope-selective quadrupolar Carr-Purcell Meiboom-Gill (QCPMG) pulse sequence to selectively excite the 47Ti or 49Ti powder linear[95]. The authors performed solid-state NMR experiments and numerical simulations on the anatase and rutile of TiO2. The 47Ti and 49Ti isotopes for anatase, with different quadrupolar interaction between the EFG at the titanium site and their nuclear quadrupole moments (Q), were separated at the field of 21.1 T, and their EFG and CSA tensors were accurately determined [Figure 4].
Figure 4. Experimental static 47Ti and 49Ti NMR spectra of anatase using the QCPMG pulse sequence. (A) The ordinary QCPMG pulse sequence; (B) the devised 49Ti-selective pulse sequence; and (C) the devised 47Ti selective pulse sequence. Reproduced with permission from[95]. Copyright 2006, Elsevier. NMR: Nuclear magnetic resonance; QCPMG: quadrupolar Carr-Purcell Meiboom-Gill.
Precisely discerning the diverse Ti environments in TiO2 presents a significant analytical challenge in static 47/49Ti NMR due to the significant overlap and inherent broadness of their spectral signatures. To overcome this problem, Epifani et al. used the MAS method at 12 kHz. This approach effectively improved the resolution of their NMR signals, allowing for a more detailed and accurate characterization of the titanium environments in both pristine TiO2 and V2O5-loaded TiO2 samples[96]. As shown in Figure 5, two resonances at 213 and -245 ppm were present in the 47/49Ti MAS NMR spectrum of amorphous TiO2 before high-temperature heating (Figure 5, Left), which correspond to surface Ti bonded to OH and lattice Ti sites in TiO2, respectively. A new dominant 49Ti resonance at 792 ppm appeared after heating at 400-500 °C. The resonance at 792 ppm was even more intense in the 47/49Ti MAS NMR spectrum of TiO2-V2O5 nanocrystals (Figure 5, Right). The new signal of 792 ppm should be ascribed to surface Ti sites, having a unique distorted tetrahedral environment unlike that of bulk anatase TiO2. The V2O5 loading facilitates the rearrangement of surface Ti sites to distorted tetrahedral geometry.
Figure 5. 47/49Ti MAS NMR spectra. (Left) pristine TiO2 samples are examined as prepared, and after heating at 400 and 500 °C. (Right) V2O5-loaded TiO2 samples were examined under identical conditions. Reproduced with permission from[96]. Copyright 2015, Elsevier. MAS: Magic angle spinning; NMR: nuclear magnetic resonance.
Oxygen active sites in TiO2
Oxygen is another most important constituent atom of TiO2 and plays a critical role in various chemical processes that occur on the catalyst surface. Thus, an understanding of the structure and distribution of the O sites helps develop more efficient and effective catalytic systems for energy storage and conversion. As previously reported[67-70,97-102], 17O MAS NMR can be utilized to distinguish the local structures of oxygen sites in oxygen-containing materials. However, the 17O quadrupolar nucleus (I = 5/2) exhibits a relatively low gyromagnetic ratio (γ = -5.774 MHz·T-1) and low 17O abundance (0.037%). Thus, it is difficult to use 17O NMR to study the oxygen-containing materials in conventional magnetic fields (≤ 14.1 T), and the 17O isotopic entailment is necessary to acquire the 17O MAS NMR spectra[103]. In the earlier study, the 17O enriched TiO2 was commonly prepared by hydrolysis of organic titanate [such as Ti(Oi-Pr)4] using 17O enriched H2O[101] or high-temperature calcination of TiO2 using 17O enriched O2[104]. Accordingly, the 17O NMR signal of bulk oxygen, which is predominant in TiO2, was solely observed. The 17O MAS NMR spectrum of anatase and rutile TiO2 exhibits a single resonance at 562 and 596 ppm, respectively, which was assigned to tri-coordinated oxygen (OTi3) in the corresponding crystal phase. Besides the resonance in the anatase (562 ppm) or rutile (596 ppm) domain, three resonances at 516, 543, and 572 ppm, corresponding to distorted OTi3 or tetra-coordinated oxygen (OTi4) sites on the interface between anatase and rutile, were observed in the spectra of mixed-phase TiO2 [Figure 6].
Figure 6. 17O MAS NMR spectra of anatase TiO2 and mixed-phase TiO2 of anatase and rutile with 17O enrichment. Reproduced with permission from[104]. Copyright 2014, Elsevier. MAS: Magic angle spinning; NMR: nuclear magnetic resonance.
Recently, Li et al. used 90% 17O-enriched H2O to realize surface-selectively 17O-labeled anatase TiO2 with dominant exposed (001) facets [TiO2(001)] and anatase TiO2 with dominant exposed (001) facets [TiO2(101)] [Figure 7][105]. Based on the 17O MAS NMR spectra and theoretical calculations, surface O sites on TiO2 exposing different facets were roughly distinguished. The signals at high frequencies (600-780 ppm) can be attributed to bridging O sites (OTi2); the peaks at 460-580 ppm should be due to tri-coordinated oxygen (OTi3) species; the resonances at lower frequencies (100-250 ppm) can be assigned to hydroxyl groups (Ti–OH); the signals at -200-30 ppm can be ascribed to adsorbed H2O. The 17O NMR parameters, including quadrupole coupling constant (CQ) and asymmetry parameter (ƞ) [Table 1]. In addition, it has been accepted that the type, content, and structure of oxygen sites on different crystal surfaces vary greatly due to the difference in surface defects and reconstruction, which can be studied by 17O MAS NMR spectroscopy. The 1H → 17O cross-polarization (CP) MAS and two-dimensional (2D) heteronuclear correlation (HETCOR) NMR have been utilized to probe the spatial proximity of the H and O atoms, which, however, is time-consuming and exhibits low detection efficiency for the quadrupolar nucleus with low γ and low surface abundance[106-108]. These conventional correlation NMR techniques validated the assignment of surface OH and adsorbed H2O on the TiO2 surface[106]. To date, it is a great challenge to identify the atomic-level structures of surface O species (including O sites, OH groups, and interfacial H2O) and the detailed interactions between them on TiO2 due to the complexity of the interfacial environments, the high mobility of interfacial H2O, and the interference from outer-layer H2O[109,110].
Figure 7. (A) Experimental and simulated 1D 17O MAS NMR spectra of TiO2 (001). The simulated spectra are based on DFT calculations on different structures; (B) The structure model of TiO2 (101); (C) Experimental and simulated 1D 17O MAS NMR spectra of the fully dried surface-selectively 17O-labeled TiO2 (101) (black line). The simulated spectra (colored lines and peaks) by using parameters obtained from DFT calculations. Reproduced with permission from[105]. Copyright 2017, Springer Nature. 1D: One-dimensional; MAS: magic angle spinning; NMR: nuclear magnetic resonance; DFT: density functional theory.
Summary of the 17O MAS NMR signals occurring in the spectra of TiO2, their chemical shifts (δiso), quadrupole coupling constants (CQ), asymmetry parameters (ƞ), and assignments according to literature
δiso (ppm) | CQ (MHz) | ƞ | Assignment | Ref. |
600-750 | < 1.7 | 0.3-0.8 | OTi2 on anatase surface | [105] |
535-570 | 1.1-1.5 | 0.2-0.8 | OTi3 in anatase | [105] |
530-550 | ca. 1.0 | 0.5-1.0 | OTi3 on anatase (001) facet | [105] |
500-560 | 1.1-1.7 | 0.5-0.8 | OTi3 on anatase (101) facet | [105] |
572 | 2.0 | 0.1 | OTi3 in low-ordered TiO2 | [104] |
596 | 1.8 | 0.6 | OTi3 in rutile | [104] |
543 | 1.6 | 0.6 | Distorted OTi3 or OTi4 near interface between anatase and rutile | [104] |
516 | 1.8 | 0.8 | Distorted OTi3 or OTi4 near interface between anatase and rutile | [104] |
100-300 | 6.2-7.0 | 0.1-0.5 | OH on anatase surface | [105] |
21 | 8.37 | 0.71 | H2O absorbed at step-edge Ti5C in OA | [105] |
7 | 8.58 | 0.7 | H2O absorbed at step-edge Ti5C in OB | [105] |
SURFACE TITANIUM AND OXYGEN SITES STUDIED BY SURFACE-ENHANCED NMR SPECTROSCOPY
Due to the high price of isotope reagents, low detection sensitivity, and low surface atomic content, it is difficult to acquire the 47/49Ti and 17O NMR spectra in a short time. Dynamic nuclear polarization (DNP) transfers the polarization of paramagnetic centers to nearby nuclei by microwave irradiation, which can enhance NMR signals in the ratio of the gyromagnetic ratio of the electron and the polarized nucleus. The DNP NMR spectroscopy instruments need to set up microwave sources and cryogenic probes (T < 120 K) to achieve efficient polarization transfer via cross effect, solid effect, and Overhauser effect[111-118]. The NMR signal enhancement techniques have been used to examine the surface structure of a variety of inorganic and hybrid materials[119-122], known as DNP-surface-enhanced NMR spectroscopy (SENS).
Direct DNP transfers polarization directly to target nuclei[123-125], and indirect DNP transfers polarization first to 1H nuclei and then to target nuclei by CP[126-129]. Both of them can be realized for TiO2 photocatalysts[130,131]. Chen et al. prepared 17O-enriched TiO2 by ball milling (BM) with 17O-enriched
Figure 8. (A) 17O MAS NMR spectra recorded at 14.1T on 17O enriched TiO2; (B) 17O MAS NMR spectrum recorded at 35.2 T on a TiO2 phase enriched in 17O; (C) 17O DNP NMR spectrum of a TiO2 phase enriched in 17O; (D) 17O DNP CPMAS NMR spectra in comparison to the DFS-enhanced echo spectrum. Reproduced with permission from[130]. Copyright 2020, American Chemical Society. MAS: Magic angle spinning; NMR: nuclear magnetic resonance; DNP: dynamic nuclear polarization; CPMAS: cross-polarization magic angle spinning; DFS: double-frequency sweep.
The long-standing obstacle of acquiring well-resolved 17O and/or 47/49Ti NMR spectra of TiO2 photocatalysts at natural abundance has been overcome with the implementation of DNP techniques. To measure the surface O and Ti structures, Nagashima et al. developed a novel pulse sequence of refocused insensitive nuclei enhanced by polarization transfer (RINEPT)-
Figure 9. (A) Pulse sequence of RINEPT-
The 1H → 47/49Ti DNP-enhanced RINEPT-
The 1H → 17O RINEPT-
Figure 10. DNP-enhanced 1H → 17O RINEPT-
The water adsorption and dissociation on the surface of metal oxide is a subject of immense importance in various fields such as photocatalysis, energy science, and material science[2,132-138]. This is because water plays a critical role in various chemical processes that occur on the surface of these materials[42,139-143]. A detailed understanding of the adsorption and dissociation of interfacial H2O on these surfaces can help researchers develop more efficient and effective catalytic systems and materials for energy storage and conversion. It has been confirmed that a water molecule could react with the oxygen vacancy or rupture over the low coordination surface Ti sites of TiO2, and form hydroxyls[141,144-147]. However, water adsorption and dissociation on nondefect titanium sites have been disputed for decades[82,148-152]. It is difficult to distinguish the Ti–OH groups formed by H2O dissociation on nondefect TiO2 surface from either the Ti–OH groups generated by H2O reaction with defect sites or the original Ti–OH groups present on TiO2. The DNP-SENS technique would provide the possibilities for exploring the detailed mechanism of water adsorption and dissociation on metal oxide.
SURFACE ELECTRONIC STRUCTURE AND PROPERTIES STUDIED BY PROBE-ASSISTED NMR TECHNIQUES
Surface structural features (including oxygen vacancies, cations, anions, and hydroxyl groups) play crucial roles in the development of efficient catalysts, especially metal oxides, and have been widely investigated[17,153-164]. The differences in these surface features result in the nano-sized particles with different physical/chemical properties. Taking anatase TiO2 nanocrystallite as an example, each facet [including (101) and (001) facet] possesses distinctive chemical properties due to the differences in both the content and electronic structure of the surface species from facet to facet. Probe molecules, including 13C-carbon monoxide, 15N-pyridine, and 31P-trimethylphosphine (TMP), can be adsorbed onto catalysts, and their different NMR chemical shift values can reflect the various microenvironments of catalyst surfaces. Thus, the chemical probe-assisted NMR has been used to characterize the electronic structure in catalyst structures. Among them, TMP is a sensitive and reliable chemical probe to clarify qualitative and quantitative information on the adsorbed sites of the various catalysts[165-168]. In general, the 31P chemical shift of -2~-5 ppm is ascribed to the TMP interacting with surface H+ (Brönsted acid, BA site), while the 31P chemical shift of -20~-58 ppm corresponds to the TMP interacting with surface exposed metal sites (i.e., Lewis acid, LA site), and a linear correlation between the 31P chemical shift and the LA strength (or the binding energy) was found[169,170].
For TiO2 nanoparticles, TMP, as the nucleophilic probe molecule, can strongly interact with the unsaturated coordinated Ti sites on the surface (that is, the surface TMP-Ti complex). Based on the 31P chemical shift of the TMP-Ti complexes, surface Ti sites on various facets with different strengths of LA, surface energies, and spatial structure can be identified. Recently, Peng et al. prepared high-quality anatase TiO2 nanocrystals with different exposed facets using hydrothermal synthesis with variable hydrogen fluoride (HF, 0-6 mL), labeled as TiO2 powder with 90% (101) facet, (101)-dominated TiO2 with 80% (101) facet, and (001)-dominated TiO2 with 75% (001) facet [Figure 11A-C][162,164]. Probe-assisted 31P solid-state NMR spectroscopy was employed to study the surface features and provided extraordinary sensitivity to their chemical states [Figure 11D-F]. There was almost no TMP-H+ complex at -2~-5 ppm present on the TiO2 powder but the TMP-LA complex had a main signal at -36 ppm and a small shoulder at -29 ppm. According to a previous report[171], the major peak and the shoulder with the integrated area ratios of 89.8% and 10.2% were attributed to the interaction between TMP and surface five-coordinate Ti sites (Ti5C) on (101) and (001) facets, respectively, which is consistent with the density functional theory (DFT) calculation [Figure 11G and H]. When the TMP interacts with the Ti5C sites on the reconstructed (1 × 4) (001) facet [(001)RC], the chemical shift of 31P NMR should be 50 ppm [Figure 11I]. Thus, the TMP-assisted NMR experiment can differentiate between facets of decreasing energy through their chemical shift values: (001) > (101) > (001)RC [Figure 11G-I]. Noteworthily, F ions are retained on the (101) and (001) facets when the TiO2 samples were prepared with HF. Owing to the electronic withdrawing effect of surface F ions exerted on Ti5C on these two facets, around 5-7 ppm downshift in chemical shift of the (101) and (001)-dominated TiO2 retained F ions from the corresponding facets of -36 ppm (101) and -29 ppm (001) in the powder sample to -31 and -22.5 ppm, respectively. The NMR signal of -42.5 ppm was ascribed to the formation of F-containing surface oxygen vacancies on unstable (001) facets. Additionally, the significant increase of the Brønsted acid signal (-2~-3 ppm) was rationalized by the interaction of protons with the fluorine (F). The surface F on the (001) and (101) facets significantly enhanced the LA strength of Ti5C sites by reflecting a downshift of 31P chemical shift. On the other hand, the post calcination of the prepared TiO2 led to partially replacing F with OH, rendering an upshift of 31P chemical shift, suggesting the LA strength of Ti5C decreased. The TiO2 surfaces tend to adsorb various surface impurity groups (including F, OH, and SO4) to relax surface energy, and they have a substantial influence on LA strength of Ti5C sites on TiO2 surfaces, which is closely related to photocatalytic activity.
Figure 11. HRTEM images of as-prepared TiO2 with (A) 90% and (B) 80% (101) facet, and (C) 75% (001) facet, and (D-F) their corresponding 31P MAS NMR spectra of TMP-adsorbed TiO2. Theoretical models and calculated adsorption energy (Ead) between TMP and Ti5C sites on various TiO2 facets, including (G) (001) facet, (H) (101) facet, and (I) the reconstructed (1 × 4) (001) facet (Ti: light grey; O: red; P: orange; C: grey; H: white). Reproduced with permission from[164]. Copyright 2017, Springer Nature. HRTEM: High-resolution transmission electron microscopy; MAS: magic angle spinning; NMR: nuclear magnetic resonance; TMP: trimethylphosphine.
It has been shown that the surface features play a crucial role in photocatalytic H2 evolution[160]. According to the TMP-assisted solid-state NMR spectroscopy, it was found that the electron density of surface Ti5C sites strongly decreased near the F ions, forming a dipole electric field (Fδ- ← Tiδ+). The photogenerated holes and electrons can be efficiently separated under the action of the F-induced surface dipole electric field, which greatly prolongs the lifetime of the photogenerated carriers and, consequently, enhances photocatalytic activities. This point was further validated using a series of surface functional groups (–O–, F, OH, and SO4), which were systematically investigated by TMP-assisted 31P NMR spectroscopy and DFT calculations[162,164]. The NMR spectra are sensitive to variations in the surface electronic properties for the series of TiO2 samples [Figure 12A-C], and accordingly, a rational theoretical model is proposed. The highly sensitive 31P chemical shift values correlate linearly and positively with the electron-withdrawing capacity of the surface functional groups to the Ti5C sites (OH < –O– < SO4 < F), indicating that these functional groups can provide fine-tuning of LA and BA sites on TiO2 surface [Figure 12D and E]. Furthermore, the adsorption energies on surfaces modified by these functional groups (OH < –O– < SO4 < F) show a linear relationship with 31P chemical shift in the solid-state NMR spectra and the activity of photocatalytic H2 evolution[162]. Moreover, the transfer process of photogenerated electrons is more efficient if the reactants are pre-adsorbed on the TiO2 surface. Thus, the TMP-assisted 31P NMR spectroscopy can sensitively probe surface electronic structure and properties, thus facilitating the design and development of efficient photocatalysts.
Figure 12. Summary of the electronic effect (chemical shift) imposed by different adsorbates during sequential treatments/modifications on (A) TiO2 PD, (B) F-capped (101) facet [F-(101)], and (C) F-capped (001) facet [F-(001)]; (D) Illustration of interaction between TMP and surface features on TiO2 facet with various treatments/modifications; (E) The summary of 31P chemical shift of TMP-adsorbed Ti5C on (001)/(101) facets with different treatments and modification. Reproduced with permission from[164]. Copyright 2017, Springer Nature. PD: powder; TMP: trimethylphosphine.
HETEROATOM ACTIVE SITES AND THEIR FUNCTION IN PHOTOCATALYSTS
The heteroatom (ions) doping should be one of the most promising methods to narrow the band gap and extend UV-light adsorption to the vis-light region. However, metallic doping (such as Al, V, and Ag) tends to be thermally unstable and inevitably introduces the recombination centers of photogenerated carriers[172-177]. On the other hand, the non-metallic doping (such as C, B, N, etc.) can introduce impurity bands located at 0.8-0.9 eV (i.e., below the conduction band bottom) due to the formation of localized oxygen vacancies[24,177-179], which results in a low electron mobility in anatase TiO2. As such, although both metallic and non-metallic implantations can achieve vis-light absorption, they do not warrant enhanced photocatalytic activity of the doped TiO2 photocatalysts. An in-depth understanding of heteroatoms (ions) sites and their mechanism is the prerequisite to establishing structure-activity relationship, which can promote the rational design of more efficient TiO2 photocatalysts.
13C-carbon
Carbon-doping of TiO2 can efficiently enhance photocatalytic H2 production, CO2 reduction, and degradation of dyes and some small organic molecules under visible irradiation[180-185]. C-doped TiO2 can be prepared by a simple sol-gel method using various carbon sources, including glucose, sucrose, and the titanium alkoxide precursor itself[186-191]. It has been confirmed that the structure and distribution of carbon species are closely correlated with the photocatalytic activity of C-doped TiO2.
Rockafellow et al. achieved 13C enrichment of carbon species in C-doped TiO2 with 13C-labeled glucose[192]. According to the one-dimensional (1D) 13C MAS NMR spectra with spectral editing and 2D 13C–13C correlation NMR spectrum, the detailed six-carbon fragments were present in C-doped TiO2 before annealing (13C6-TiO2-0, Figure 13A and B). After annealing, the aromatic species were the main component of carbon species in the hybrid TiO2 materials. Interestingly, when a washing step is added between the initial drying and annealing (13C6-TiO2-5W), the major carbon species are transformed into an orthocarbonate structure, with C substituting Ti sites inside the TiO2 [Figure 13C]. However, the reason for this change was still ambiguous. The authors found that the presence or absence of aromatic-carbon species on the TiO2 surface was unrelated to the rate of photocatalytic degradation of quinoline, but had a significant effect on the product distribution. Instead, the reason for the high reactivity is presumed to be the formation of orthocarbonate centers.
Figure 13. (A) 13C NMR spectra of 13C6-TiO2-0. The spectrum of glucose for reference. Spectra of 13C6-TiO2-0 with spectral editing; (B) 2D 13C–13C correlation spectrum of 13C6-TiO2-0. Inserts: two structural fragments consistent with the observed cross peaks; (C) 13C NMR spectra of 13C6-TiO2-5W: quantitative (DP) spectrum of all C and corresponding spectrum of nonprotonated C, J-modulated dephasing spectra, and selection of sp3-hybridized C by a five-pulse CSA filter. Reproduced with permission from[192]. Copyright 2009, American Chemical Society. NMR: Nuclear magnetic resonance; 2D: two-dimensional; DP: direct polarization; CSA: chemical shift anisotropy.
We also reported a study of a minute quantity of carbon species doping on TiO2 by using the titanium alkoxide precursor itself[190]. According to the 13C MAS NMR experiments, the detailed structural changes of surface carbon species have been clarified [Figure 14]. After the C doping, four types of carbon species were observed, including carboxylate (182.9 ppm), graphite-like C (129 ppm), aromatics C (128.3 ppm), alkyl C (7.6-29.8 ppm). After washing the C-doped TiO2 with HCl solution, the graphite-like C species should be the main carbon-containing component in the photocatalyst. It was found that graphite-like C species should be the active site to promote the separation of photogenerated carriers, resulting in high photocatalytic efficiency. In contrast, surface alkoxy and carboxylate C species would poison severely the
Figure 14. 13C MAS NMR spectra of various C-doped TiO2 samples (Right). Proposed hole and electron transfer mechanism in the C-doped TiO2 photocatalyst (Left). Reproduced with permission from[190]. Copyright 2018, American Chemical Society. MAS: Magic angle spinning; NMR: nuclear magnetic resonance.
15N-nitrogen
Nitrogen doping of TiO2 can efficiently promote vis-light adsorption[24,194-199]. Reyes-Garcia et al. reported the detailed structure of nitrogen species in the N-doped TiO2 prepared from different dopant precursors and methods through 15N solid-state NMR analysis[200]. As shown in Figure 15, three types of amino species (at
Figure 15. (A) 15N MAS NMR spectra of 15N-doped TiO2 prepared by sol-gel methods; (B) 15N MAS NMR spectra of 15N-doped TiO2 monolayer and powders by direct nitridation. Reproduced with permission from[200]. Copyright 2007, American Chemical Society. MAS: Magic angle spinning; NMR: nuclear magnetic resonance.
11B-boron
The boron-doping can not only narrow the band gap of TiO2 effectively to extend the absorption band to the vis-light region but also facilitate the separation of photogenerated carriers to promote photocatalytic activities[23,201-205]. Thus, the categories and structure of boron species have been extensively studied to gain their correlations with the photocatalytic properties. The conclusions of the aforementioned research were mostly obtained by XPS, which, however, remain controversial. For example, the XPS peaks at 190.5-
To gain more insights into the structure-activity relationship in photocatalytic reactions, we incorporated FAM RF pulse trains into the MQ MAS sequence, namely the so-called 3QZ-FAM MAS NMR technique, to improve the sensitivity of the 11B NMR spectroscopy and investigate the detailed chemical environments of boron in B-doped and (B, Ag)-codoped TiO2 photocatalysts [Figure 16A and B][202]. Up to five B sites were distinguished, corresponding to surface sites (B5), small B polymer (B2 and B3), interstitial T* (tri-coordinated, B4), and Q* (pseudo-tetrahedral coordinated, B1) sites. Noteworthily, substituted B sites were absent in the materials. A 2D 11B-11B double-quantum (DQ) MAS NMR technique was first used to reveal the spatial distributions of the B sites in the B-doped and (B, Ag)-codoped TiO2 photocatalysts [Figure 16C and D]. Accordingly, we found that only the tri-coordinated interstitial boron (T*) species was near the substitutional Ag species to form [T*–O–Ag] structural units. Combined with the evolution of the chemical states of the B and Ag dopants revealed by in-situ XPS experiments, a unique intermediate structure was formed by the [T*–O–Ag] units trapping the photogenerated electron in the (B, Ag)-codoped TiO2 during the irradiation as shown in Figure 17. To date, the developed 2D 11B-11B DQ Correlation NMR technique has been used to detect the spatial correlation of the B species in various B-containing materials, including boron nitride (BN), activated carbon impregnated with boric acid (B/OAC), boron-substituted MCM-22 zeolite (B-MWW) and silica-supported boron oxide (B/SiO2)[39,212,213,217].
Figure 16. 2D 11B 3QZ-FAM MAS NMR spectra (sheared) of (A) 10% B-doped and (B) (B, Ag)-codoped TiO2 samples. 2D 11B DQ MAS NMR spectra of (C) 10% B-doped and (D) (B, Ag)-codoped TiO2 samples. Possible Boron Species in B-doped and (B, Ag)-codoped TiO2 were shown at the bottom. Reproduced with permission from[202]. Copyright 2013, American Chemical Society. 2D: Two-dimensional; FAM: fast amplitude-modulated; MAS: magic angle spinning; NMR: nuclear magnetic resonance; DQ: double-quantum.
Figure 17. Electron/hole transfer mechanism for the (B, Ag)-codoped TiO2 photocatalyst under irradiation from a solar-light source. Reproduced with permission from[202]. Copyright 2013, American Chemical Society.
27Al-aluminum
Although aluminum (Al)-doping cannot promote vis-light absorption, it would affect the crystal growth, cation diffusivity, and conductivity of TiO2[176,218-220]. For photocatalysis, several contradictory results occurred due to the ambiguity of the structure-activity relationship of the Al sites in the Al-doped TiO2 photocatalysts. Some reports found that Al doping could promote the separation of photogenerated carriers and thus improve photocatalytic activity[221-223]. On the other hand, others proposed that Al doping might introduce recombination centers of photogenerated carriers, which could have a negative impact on photocatalytic activity[220,224]. Thus, it is essential to identify the active Al species in the Al-doped TiO2.
To unravel the origins of the exceptional activity of Al-doped TiO2 with dominant (001) facets [Al-TiO2-xFx(001)], we employed advanced solid-state NMR methods to elucidate the fine structural details of the dopants, specifically F and Al species [Figure 18][225]. Notably, we first applied the 2D 19F–27Al dipolar heteronuclear multiple-quantum coherence (D-HMQC) NMR technique to probe F–Al proximity, enabling definitive confirmation of the F–Ti2Al structural motif within the Al-TiO2-xFx(001) samples. According to the quantitative 19F NMR measurements, the content of the F–Ti2Al structure rises with greater Al doping, while that of other structures (including Ti–F–Ti, F–Ti3, and Ti–F–Al) hardly increases. Combined with the spin-trapping electron spin resonance (ESR) results, it was found that the formation of the F–Ti2Al structure promotes the separation and transfer of photogenerated carriers in the Al-TiO2-xFx(001) photocatalyst and is, therefore, considered to be the active site for photocatalytic reactions. However, with the further increase of Al doping, the oxygen vacancies occur, which should be the recombination centers of photogenerated carriers as reported in the previous work[176,220].
Figure 18. (A) 1D 27Al MAS NMR spectra of TiO2-xFx(001) and Al–TiO2-xFx(001) catalysts; (B) 2D 27Al 3Q MAS NMR spectrum of the
CONCLUSION AND OUTLOOK
Solid-state NMR spectroscopy can provide detailed information about the nature of the active centers and their structure-activity relationships in the TiO2 photocatalysts. The local structure and coordination of different sites or species in the TiO2 framework or on the TiO2 surface can be identified by NMR chemical shift, owing to the high sensitivity of the NMR technique to the surrounding electronic environment. For example, bulk titanium and surface titanium can be distinguished by 47/49Ti chemical shifts, and bulk oxygen, surface oxygen sites, hydroxyl groups, and adsorbed H2O with different coordination states can be identified by 17O chemical shifts. Surface electronic structure and properties, including the type, strength, and concentration of diverse adsorption sites, can be detected by probe-assisted NMR techniques. The correlation and connectivity of different sites or species in TiO2 can be extracted from the specific internuclear interactions, including dipolar-dipolar and J-coupling interactions. Various advanced homonuclear/heteronuclear correlation NMR techniques based on dipole–dipole interaction or J-coupling have been developed to detect the dipolar-dipolar interactions for identifying internuclear proximities or chemical bonding, respectively[226,227]. For example, the spatial proximity between different active sites on hetero-atom (such as C, B, Al, etc.)-modified TiO2 can be probed by 2D homonuclear (13C–13C/11B–11B) and heteronuclear (19F–27Al) correlation NMR spectra, while the 2D 1H–11B/17O/47/49Ti/95Mo correlation NMR can identify the surface B/O/Ti/Mo site in the TiO2 photocatalysts, which facilitate the solution of the complex surface structure.
Although remarkable progress has been achieved on the application and development of solid-state NMR methods, considerable challenges remain in TiO2 photocatalysis for solid-state NMR characterization. It is difficult to detect dilute heteroatom sites and low-content surface/interface species (such as surface O and Ti sites) at high resolution. The intrinsic low sensitivity of solid-state NMR limits its application for this aspect, especially for some infamous nuclei with low natural abundances and low-γ features. All these active centers (including surface active sites and heteroatom sites) are fundamentally important in TiO2 photocatalysis. Moreover, the complexity of complex surface structures, heteroatom distributions, and various covalent and non-covalent interactions in the TiO2 photocatalysts leaves a huge space for advanced solid-state NMR techniques.
Our understanding of the complex structure of TiO2 photocatalysts remains limited by the capabilities of existing solid-state NMR hardware and methodologies. An in-depth understanding of their structure-function relationships is essential for the further development of these fields. The increasing availability of ultra-high-field magnets and cryoprobes is improving solid-state NMR techniques to a higher level of detection sensitivity and resolution. Further development of sensitivity-enhanced 2D NMR techniques would enable the identification of the microstructure, distribution, and interaction of different active sites. Notably, the typical dopant elements used to modify TiO2 photocatalysts, such as 51V, 67Zn, 71Ga, 93Nb, and
DECLARATIONS
Authors’ contributions
Prepared and revised the manuscript: Feng N
Revised the manuscript: Feng N, Xu J, Deng F
Availability of data and materials
Not applicable.
Financial support and sponsorship
This work was supported by the National Natural Science Foundation of China (22372177, 22127801, 22225205, 22320102002, 22161132028), the Strategic Priority Research Program of the Chinese Academy of Sciences (XDB0540000), Natural Science Foundation of Hubei Province (S22H120101), Hubei International Scientific and Technological Cooperation Program (2022EHB021), and International Collaborative Center for Sustainable Catalysis and Magnetic Resonance (SH2303).
Conflicts of interest
Xu J served as editorial member of Chemical Synthesis, and he is Guest Editor of the Special Issue: “Advanced Characterization Techniques and Applications for Catalytic Materials”, while the other authors have declared that they have no conflicts of interest.
Ethical approval and consent to participate
Not applicable.
Consent for publication
Not applicable.
Copyright
© The Author(s) 2024.
REFERENCES
1. Ruan X, Li S, Huang C, Zheng W, Cui X, Ravi SK. Catalyzing artificial photosynthesis with TiO2 heterostructures and hybrids: emerging trends in a classical yet contemporary photocatalyst. Adv Mater 2024;36:e2305285.
2. Fujishima A, Honda K. Electrochemical photolysis of water at a semiconductor electrode. Nature 1972;238:37-8.
3. Tang R, Zhou S, Zhang Z, Zheng R, Huang J. Engineering nanostructure-interface of photoanode materials toward photoelectrochemical water oxidation. Adv Mater 2021;33:e2005389.
4. Warnan J, Reisner E. Synthetic organic design for solar fuel systems. Angew Chem Int Ed Engl 2020;59:17344-54.
5. Wang Z, Hisatomi T, Li R, et al. Efficiency accreditation and testing protocols for particulate photocatalysts toward solar fuel production. Joule 2021;5:344-59.
6. Ismail AA, Bahnemann DW. Photochemical splitting of water for hydrogen production by photocatalysis: a review. Sol Energy Mater Sol Cells 2014;128:85-101.
7. Rawool SA, Yadav KK, Polshettiwar V. Defective TiO2 for photocatalytic CO2 conversion to fuels and chemicals. Chem Sci 2021;12:4267-99.
8. Morikawa T, Sato S, Sekizawa K, Suzuki TM, Arai T. Solar-driven CO2 reduction using a semiconductor/molecule hybrid photosystem: from photocatalysts to a monolithic artificial leaf. Acc Chem Res 2022;55:933-43.
9. Li Y, Lei Y, Li D, et al. Recent progress on photocatalytic CO2 conversion reactions over plasmonic metal-based catalysts. ACS Catal 2023;13:10177-204.
10. Lin Z, Jiang X, Xu W, et al. The effects of water, substrate, and intermediate adsorption on the photocatalytic decomposition of air pollutants over nano-TiO2 photocatalysts. Phys Chem Chem Phys 2024;26:662-78.
11. Wu H, Li L, Wang S, et al. Recent advances of semiconductor photocatalysis for water pollutant treatment: mechanisms, materials and applications. Phys Chem Chem Phys 2023;25:25899-924.
12. Linsebigler AL, Lu G, Yates JT. Photocatalysis on TiO2 surfaces: principles, mechanisms, and selected results. Chem Rev 1995;95:735-58.
13. Chen LX, Rajh T, Jäger W, Nedeljkovic J, Thurnauer MC. X-ray absorption reveals surface structure of titanium dioxide nanoparticles. J Synchrotron Radiat 1999;6:445-7.
15. Pan J, Liu G, Lu GQ, Cheng HM. On the true photoreactivity order of {001}, {010}, and {101} facets of anatase TiO2 crystals. Angew Chem Int Ed Engl 2011;50:2133-7.
16. Yang HG, Sun CH, Qiao SZ, et al. Anatase TiO2 single crystals with a large percentage of reactive facets. Nature 2008;453:638-41.
17. Feng N, Lin H, Song H, et al. Efficient and selective photocatalytic CH4 conversion to CH3OH with O2 by controlling overoxidation on TiO2. Nat Commun 2021;12:4652.
18. Yu J, Zhao X, Zhao Q. Effect of film thickness on the grain size and photocatalytic activity of the sol-gel derived nanometer TiO2 thin films. J Mater Sci Lett 2000;19:1015-7.
19. Zhu J, Liao M, Zhao C, et al. A comprehensive review on semiconductor-based photocatalysts toward the degradation of persistent pesticides. Nano Res 2023;16:6402-43.
20. Chen F, Ma T, Zhang T, Zhang Y, Huang H. Atomic-level charge separation strategies in semiconductor-based photocatalysts. Adv Mater 2021;33:e2005256.
21. Wang H, Liu W, He X, Zhang P, Zhang X, Xie Y. An excitonic perspective on low-dimensional semiconductors for photocatalysis. J Am Chem Soc 2020;142:14007-22.
22. Liu N, Schneider C, Freitag D, et al. Black TiO2 nanotubes: cocatalyst-free open-circuit hydrogen generation. Nano Lett 2014;14:3309-13.
23. Wang F, Jiang Y, Gautam A, Li Y, Amal R. Exploring the origin of enhanced activity and reaction pathway for photocatalytic H2 production on Au/B-TiO2 catalysts. ACS Catal 2014;4:1451-7.
24. Asahi R, Morikawa T, Ohwaki T, Aoki K, Taga Y. Visible-light photocatalysis in nitrogen-doped titanium oxides. Science 2001;293:269-71.
25. Chen S, Hu YH. Color TiO2 materials as emerging catalysts for visible-NIR light photocatalysis, a review. Catal Rev 2023.
26. Li X, Wu X, Liu S, Li Y, Fan J, Lv K. Effects of fluorine on photocatalysis. Chin J Catal 2020;41:1451-67.
27. Peiris S, de Silva HB, Ranasinghe KN, Bandara SV, Perera IR. Recent development and future prospects of TiO2 photocatalysis. J Chin Chem Soc 2021;68:738-69.
28. Xiu Z, Guo M, Zhao T, et al. Recent advances in Ti3+ self-doped nanostructured TiO2 visible light photocatalysts for environmental and energy applications. Chem Eng J 2020;382:123011.
29. Medhi R, Marquez MD, Lee TR. Visible-light-active doped metal oxide nanoparticles: review of their synthesis, properties, and applications. ACS Appl Nano Mater 2020;3:6156-85.
30. Kovačič Ž, Likozar B, Huš M. Photocatalytic CO2 reduction: a review of ab initio mechanism, kinetics, and multiscale modeling simulations. ACS Catal 2020;10:14984-5007.
31. Wu S, Lin Y, Hu YH. Strategies of tuning catalysts for efficient photodegradation of antibiotics in water environments: a review. J Mater Chem A 2021;9:2592-611.
32. Rahman MZ, Raziq F, Zhang H, Gascon J. Key strategies for enhancing H2 production in transition metal oxide based photocatalysts. Angew Chem Int Ed Engl 2023;62:e202305385.
33. Chakhtouna H, Benzeid H, Zari N, Qaiss AEK, Bouhfid R. Recent progress on Ag/TiO2 photocatalysts: photocatalytic and bactericidal behaviors. Environ Sci Pollut Res Int 2021;28:44638-66.
34. Li X, Wei H, Song T, Lu H, Wang X. A review of the photocatalytic degradation of organic pollutants in water by modified TiO2. Water Sci Technol 2023;88:1495-507.
35. Yao S, He J, Gao F, et al. Highly selective semiconductor photocatalysis for CO2 reduction. J Mater Chem A 2023;11:12539-58.
36. Lee D, Kim M, Danish M, Jo W. State-of-the-art review on photocatalysis for efficient wastewater treatment: attractive approach in photocatalyst design and parameters affecting the photocatalytic degradation. Catal Commun 2023;183:106764.
37. Lin S, Huang H, Ma T, Zhang Y. Photocatalytic oxygen evolution from water splitting. Adv Sci 2020;8:2002458.
38. Cao Y, Zhou P, Tu Y, et al. Modification of TiO2 nanoparticles with organodiboron molecules inducing stable surface Ti3+ complex. iScience 2019;20:195-204.
39. Jung D, Saleh LMA, Berkson ZJ, et al. A molecular cross-linking approach for hybrid metal oxides. Nat Mater 2018;17:341-8.
40. Kowalska E, Yoshiiri K, Wei Z, et al. Hybrid photocatalysts composed of titania modified with plasmonic nanoparticles and ruthenium complexes for decomposition of organic compounds. Appl Catal B Environ 2015;178:133-43.
41. Rengifo-herrera JA, Blanco M, Wist J, Florian P, Pizzio LR. TiO2 modified with polyoxotungstates should induce visible-light absorption and high photocatalytic activity through the formation of surface complexes. Appl Catal B Environ 2016;189:99-109.
42. Liu F, Feng N, Wang Q, et al. Transfer channel of photoinduced holes on a TiO2 surface as revealed by solid-state nuclear magnetic resonance and electron spin resonance spectroscopy. J Am Chem Soc 2017;139:10020-8.
43. Jaeger CD, Bard AJ. Spin trapping and electron spin resonance detection of radical intermediates in the photodecomposition of water at titanium dioxide particulate systems. J Phys Chem 1979;83:3146-52.
44. Nosaka Y, Komori S, Yawata K, Hirakawa T, Nosaka AY. Photocatalytic ˙OH radical formation in TiO2 aqueous suspension studied by several detection methods. Phys Chem Chem Phys 2003;5:4731-5.
45. Liu G, Yang HG, Wang X, et al. Visible light responsive nitrogen doped anatase TiO2 sheets with dominant {001} facets derived from TiN. J Am Chem Soc 2009;131:12868-9.
46. Ishibashi K, Fujishima A, Watanabe T, Hashimoto K. Quantum yields of active oxidative species formed on TiO2 photocatalyst. J Photoch Photobio A 2000;134:139-42.
47. Guan H, Lin J, Qiao B, et al. Catalytically active Rh sub-nanoclusters on TiO2 for CO oxidation at cryogenic temperatures. Angew Chem Int Ed Engl 2016;55:2820-4.
48. Wu N, Wang J, Tafen de N, et al. Shape-enhanced photocatalytic activity of single-crystalline anatase TiO2 (101) nanobelts. J Am Chem Soc 2010;132:6679-85.
49. Micic OI, Zhang Y, Cromack KR, Trifunac AD, Thurnauer MC. Trapped holes on titania colloids studied by electron paramagnetic resonance. J Phys Chem 1993;97:7277-83.
50. Yang L, Feng N, Wang Q, Chu Y, Xu J, Deng F. Surface water loading on titanium dioxide modulates photocatalytic water splitting. Cell Rep Phys Sci 2020;1:100013.
51. Yuan W, Zhu B, Fang K, et al. In situ manipulation of the active Au-TiO2 interface with atomic precision during CO oxidation. Science 2021;371:517-21.
52. Song S, Song H, Li L, et al. A selective Au-ZnO/TiO2 hybrid photocatalyst for oxidative coupling of methane to ethane with dioxygen. Nat Catal 2021;4:1032-42.
53. Monai M, Jenkinson K, Melcherts AEM, et al. Restructuring of titanium oxide overlayers over nickel nanoparticles during catalysis. Science 2023;380:644-51.
54. Xu M, Qin X, Xu Y, et al. Boosting CO hydrogenation towards C2+ hydrocarbons over interfacial TiO2-x/Ni catalysts. Nat Commun 2022;13:6720.
55. Wei J, Qin SN, Liu JL, et al. In situ raman monitoring and manipulating of interfacial hydrogen spillover by precise fabrication of Au/TiO2/Pt sandwich structures. Angew Chem Int Ed Engl 2020;59:10343-7.
56. Zhu K, Zhu Q, Jiang M, et al. Modulating Ti t2g orbital occupancy in a Cu/TiO2 composite for selective photocatalytic CO2 reduction to CO. Angew Chem Int Ed Engl 2022;61:e202207600.
57. Balajka J, Hines MA, DeBenedetti WJI, et al. High-affinity adsorption leads to molecularly ordered interfaces on TiO2 in air and solution. Science 2018;361:786-9.
58. Guo Y, Huang Y, Zeng B, et al. Photo-thermo semi-hydrogenation of acetylene on Pd1/TiO2 single-atom catalyst. Nat Commun 2022;13:2648.
59. Chen Y, Soler L, Cazorla C, et al. Facet-engineered TiO2 drives photocatalytic activity and stability of supported noble metal clusters during H2 evolution. Nat Commun 2023;14:6165.
60. Len T, Afanasiev P, Yan Y, Aouine M, Morfin F, Piccolo L. Operando X-ray absorption spectroscopic study of ultradispersed Mo/TiO2 CO2-hydrogenation catalysts: why does rutile promote methanol synthesis? ACS Catal 2023;13:13982-93.
61. Berger T, Sterrer M, Diwald O, et al. Light-induced charge separation in anatase TiO2 particles. J Phys Chem B 2005;109:6061-8.
62. Nakamura R, Imanishi A, Murakoshi K, Nakato Y. In situ FTIR studies of primary intermediates of photocatalytic reactions on nanocrystalline TiO2 films in contact with aqueous solutions. J Am Chem Soc 2003;125:7443-50.
63. Copéret C, Liao WC, Gordon CP, Ong TC. Active sites in supported single-site catalysts: an NMR perspective. J Am Chem Soc 2017;139:10588-96.
64. Xu J, Wang Q, Deng F. Metal active sites and their catalytic functions in zeolites: insights from solid-state NMR spectroscopy. Acc Chem Res 2019;52:2179-89.
65. Ashbrook SE, Sneddon S. New methods and applications in solid-state NMR spectroscopy of quadrupolar nuclei. J Am Chem Soc 2014;136:15440-56.
66. Kwak JH, Hu J, Mei D, et al. Coordinatively unsaturated Al3+ centers as binding sites for active catalyst phases of platinum on γ-Al2O3. Science 2009;325:1670-3.
67. Wang Q, Li W, Hung I, et al. Mapping the oxygen structure of γ-Al2O3 by high-field solid-state NMR spectroscopy. Nat Commun 2020;11:3620.
68. Peng L, Liu Y, Kim N, Readman JE, Grey CP. Detection of Brønsted acid sites in zeolite HY with high-field 17O-MAS-NMR techniques. Nat Mater 2005;4:216-9.
69. Ashbrook SE, Smith ME. Solid state 17O NMR-an introduction to the background principles and applications to inorganic materials. Chem Soc Rev 2006;35:718-35.
70. Wang M, Wu XP, Zheng S, et al. Identification of different oxygen species in oxide nanostructures with 17O solid-state NMR spectroscopy. Sci Adv 2015;1:e1400133.
71. Beck TJ, Klust A, Batzill M, Diebold U, Di Valentin C, Selloni A. Surface structure of TiO2(011)-(2×1). Phys Rev Lett 2004;93:036104.
72. Di Valentin C, Tilocca A, Selloni A, et al. Adsorption of water on reconstructed rutile TiO2(011)-(2 × 1): Ti=O double bonds and surface reactivity. J Am Chem Soc 2005;127:9895-903.
73. He Y, Tilocca A, Dulub O, Selloni A, Diebold U. Local ordering and electronic signatures of submonolayer water on anatase TiO2(101). Nat Mater 2009;8:585-9.
74. Yuan W, Zhu B, Li XY, et al. Visualizing H2O molecules reacting at TiO2 active sites with transmission electron microscopy. Science 2020;367:428-30.
75. Aronson BJ, Blanford CF, Stein A. Solution-phase grafting of titanium dioxide onto the pore surface of mesoporous silicates: synthesis and structural characterization. Chem Mater 1997;9:2842-51.
76. Shukri G, Kasai H. Density functional theory study of ethylene adsorption on clean anatase TiO2 (001) surface. Surf Sci 2014;619:59-66.
77. Sanz JF, Hernández NC, Márquez A. A first principles study of Pd deposition on the TiO2 (110) surface. TheorChem Acc 2000;104:317-22.
78. Zhou G, Jiang L, Dong Y, Li R, He D. Engineering the exposed facets and open-coordinated sites of brookite TiO2 to boost the loaded Ru nanoparticle efficiency in benzene selective hydrogenation. Appl Surf Sci 2019;486:187-97.
79. Vijay A, Mills G, Metiu H. Adsorption of gold on stoichiometric and reduced rutile TiO2 (110) surfaces. J Chem Phys 2003;118:6536-51.
80. Posternak M, Baldereschi A, Delley B. Dissociation of water on anatase TiO2 nanoparticles: the role of undercoordinated Ti atoms at edges. J Phys Chem C 2009;113:15862-7.
81. Dette C, Pérez-osorio MA, Mangel S, Giustino F, Jung SJ, Kern K. Atomic structure of water monolayer on anatase TiO2 (101) surface. J Phys Chem C 2018;122:11954-60.
82. Dette C, Pérez-osorio MA, Mangel S, Giustino F, Jung SJ, Kern K. Single-molecule vibrational spectroscopy of H2O on anatase TiO2 (101). J Phys Chem C 2017;121:1182-7.
83. Gopal NO, Lo HH, Sheu SC, Ke SC. A potential site for trapping photogenerated holes on rutile TiO2 surface as revealed by EPR spectroscopy: an avenue for enhancing photocatalytic activity. J Am Chem Soc 2010;132:10982-3.
84. Nolan M, Iwaszuk A, Gray KA. Localization of photoexcited electrons and holes on low coordinated Ti and O sites in free and supported TiO2 nanoclusters. J Phys Chem C 2014;118:27890-900.
85. Xiong F, Yu YY, Wu Z, et al. Methanol conversion into dimethyl ether on the anatase TiO2(001) surface. Angew Chem Int Ed Engl 2016;55:623-8.
86. Dmitrieva LV, Vorotilova LS, Podkorytov IS, Shelyapina ME. A comparison of NMR spectral parameters of 47Ti and 49Ti nuclei in rutile and anatase. Phys Solid State 1999;41:1097-9.
87. Ganapathy S, Gore KU, Kumar R, Amoureux JP. Multinuclear (27Al, 29Si, 47,49Ti) solid-state NMR of titanium substituted zeolite USY. Solid State Nucl Magn Reson 2003;24:184-95.
88. Yamada K, Saito M, Ohashi R, Nakai T, Deguchi K, Shimizu T. Solid-state 47/49Ti nuclear magnetic resonance of TiO2. Chem Lett 2014;43:1520-1.
89. Quantities, units and symbols in physical chemistry. 2nd editon. 1993. Available from: https://old.iupac.org/publications/books/gbook/green_book_2ed.pdf. [Last accessed on 5 Jul 2024].
92. Bastow TJ, Whitfield HJ. 47,49Ti NMR: evolution of crystalline TiO2 from the gel state. Chem Mater 1999;11:3518-20.
93. Gervais C, Smith ME, Pottier A, Jolivet J, Babonneau F. Solid-State 47,49Ti NMR determination of the phase distribution of titania nanoparticles. Chem Mater 2001;13:462-7.
94. Bräuniger T, Madhu PK, Pampel A, Reichert D. Application of fast amplitude-modulated pulse trains for signal enhancement in static and magic-angle-spinning 47,49Ti-NMR spectra. Solid State Nucl Magn Reson 2004;26:114-20.
95. Larsen FH, Farnan I, Lipton AS. Separation of 47Ti and 49Ti solid-state NMR lineshapes by static QCPMG experiments at multiple fields. J Magn Reson 2006;178:228-36.
96. Epifani M, Comini E, Díaz R, Force C, Siciliano P, Faglia G. TiO2 colloidal nanocrystals surface modification by V2O5 species: Investigation by 47,49Ti MAS-NMR and H2, CO and NO2 sensing properties. Appl Surf Sci 2015;351:1169-73.
97. Gerothanassis IP. Oxygen-17 NMR spectroscopy: basic principles and applications (part I). Prog Nucl Magn Reson Spectrosc 2010;56:95-197.
98. Gerothanassis IP. Oxygen-17 NMR spectroscopy: basic principles and applications. Part II. Prog Nucl Magn Reson Spectrosc 2010;57:1-110.
99. Bastow TJ, Doran G, Whitfield HJ. Electron diffraction and 47,49Ti and 17O NMR studies of natural and synthetic brookite. Chem Mater 2000;12:436-9.
100. Lafond V, Gervais C, Maquet J, Prochnow D, Babonneau F, Mutin PH. 17O MAS NMR study of the bonding mode of phosphonate coupling molecules in a titanium oxo-alkoxo-phosphonate and in titania-based hybrid materials. Chem Mater 2003;15:4098-103.
101. Bastow TJ, Moodie AF, Smith ME, Whitfield HJ. Characterisation of titania gels by 17O nuclear magnetic resonance and electron diffraction. J Mater Chem 1993;3:697-702.
102. Rao Y, Kemp TF, Trudeau M, Smith ME, Antonelli DM. 17O and 15N solid state NMR studies on ligand-assisted templating and oxygen coordination in the walls of mesoporous Nb, Ta and Ti oxides. J Am Chem Soc 2008;130:15726-31.
103. Métro TX, Gervais C, Martinez A, Bonhomme C, Laurencin D. Unleashing the potential of 17O NMR spectroscopy using mechanochemistry. Angew Chem Int Ed Engl 2017;56:6803-7.
104. Sun X, Dyballa M, Yan J, Li L, Guan N, Hunger M. Solid-state NMR investigation of the 16/17O isotope exchange of oxygen species in pure-anatase and mixed-phase TiO2. Chem Phys Lett 2014;594:34-40.
105. Li Y, Wu XP, Jiang N, et al. Distinguishing faceted oxide nanocrystals with 17O solid-state NMR spectroscopy. Nat Commun 2017;8:581.
106. Li Y, Wu XP, Liu C, et al. NMR and EPR studies of partially reduced TiO2. Acta Phys Chim Sin 2020;36:1905021.
107. Peng L, Huo H, Liu Y, Grey CP. 17O magic angle spinning NMR studies of Brønsted acid sites in zeolites HY and HZSM-5. J Am Chem Soc 2007;129:335-46.
108. Merle N, Trébosc J, Baudouin A, et al. 17O NMR gives unprecedented insights into the structure of supported catalysts and their interaction with the silica carrier. J Am Chem Soc 2012;134:9263-75.
109. Guo Q, Ma Z, Zhou C, Ren Z, Yang X. Single molecule photocatalysis on TiO2 surfaces. Chem Rev 2019;119:11020-41.
110. Chen J, Hope MA, Lin Z, et al. Interactions of oxide surfaces with water revealed with solid-state NMR spectroscopy. J Am Chem Soc 2020;142:11173-82.
111. Ravera E, Luchinat C, Parigi G. Basic facts and perspectives of Overhauser DNP NMR. J Magn Reson 2016;264:78-87.
112. Gurinov A, Sieland B, Kuzhelev A, et al. Mixed-valence compounds as polarizing agents for overhauser dynamic nuclear polarization in solids*. Angew Chem Int Ed Engl 2021;60:15371-5.
113. Küçük SE, Biktagirov T, Sezer D. Carbon and proton Overhauser DNP from MD simulations and ab initio calculations: TEMPOL in acetone. Phys Chem Chem Phys 2015;17:24874-84.
114. Wiśniewski D, Karabanov A, Lesanovsky I, Köckenberger W. Solid effect DNP polarization dynamics in a system of many spins. J Magn Reson 2016;264:30-8.
115. Banerjee D, Shimon D, Feintuch A, Vega S, Goldfarb D. The interplay between the solid effect and the cross effect mechanisms in solid state 13C DNP at 95 GHz using trityl radicals. J Magn Reson 2013;230:212-9.
116. Liao W, Ghaffari B, Gordon CP, Xu J, Copéret C. Dynamic nuclear polarization surface enhanced NMR spectroscopy (DNP SENS): principles, protocols, and practice. Curr Opin Colloid In 2018;33:63-71.
117. Park H, Uluca-Yazgi B, Heumann S, et al. Heteronuclear cross-relaxation effect modulated by the dynamics of N-functional groups in the solid state under 15N DP-MAS DNP. J Magn Reson 2020;312:106688.
118. Hovav Y, Feintuch A, Vega S. Theoretical aspects of dynamic nuclear polarization in the solid state - the cross effect. J Magn Reson 2012;214:29-41.
119. Rankin AGM, Trébosc J, Pourpoint F, Amoureux JP, Lafon O. Recent developments in MAS DNP-NMR of materials. Solid State Nucl Magn Reson 2019;101:116-43.
120. Lesage A, Lelli M, Gajan D, et al. Surface enhanced NMR spectroscopy by dynamic nuclear polarization. J Am Chem Soc 2010;132:15459-61.
121. Rossini AJ, Zagdoun A, Lelli M, Lesage A, Copéret C, Emsley L. Dynamic nuclear polarization surface enhanced NMR spectroscopy. Acc Chem Res 2013;46:1942-51.
122. Kobayashi T, Perras FA, Slowing II, Sadow AD, Pruski M. Dynamic nuclear polarization solid-state NMR in heterogeneous catalysis research. ACS Catal 2015;5:7055-62.
123. Li W, Wang Q, Xu J, et al. Probing the surface of γ-Al2O3 by oxygen-17 dynamic nuclear polarization enhanced solid-state NMR spectroscopy. Phys Chem Chem Phys 2018;20:17218-25.
124. Blanc F, Sperrin L, Jefferson DA, Pawsey S, Rosay M, Grey CP. Dynamic nuclear polarization enhanced natural abundance 17O spectroscopy. J Am Chem Soc 2013;135:2975-8.
125. Perras FA, Boteju KC, Slowing II, Sadow AD, Pruski M. Direct 17O dynamic nuclear polarization of single-site heterogeneous catalysts. Chem Commun 2018;54:3472-5.
126. Perras FA, Kobayashi T, Pruski M. Natural Abundance 17O DNP two-dimensional and surface-enhanced NMR spectroscopy. J Am Chem Soc 2015;137:8336-9.
127. Giovine R, Trébosc J, Pourpoint F, Lafon O, Amoureux JP. Magnetization transfer from protons to quadrupolar nuclei in solid-state NMR using PRESTO or dipolar-mediated refocused INEPT methods. J Magn Reson 2019;299:109-23.
128. Zhao X, Hoffbauer W, Schmedt auf der Günne J, Levitt MH. Heteronuclear polarization transfer by symmetry-based recoupling sequences in solid-state NMR. Solid State Nucl Magn Reson 2004;26:57-64.
129. Perras FA, Kobayashi T, Pruski M. PRESTO polarization transfer to quadrupolar nuclei: implications for dynamic nuclear polarization. Phys Chem Chem Phys 2015;17:22616-22.
130. Chen CH, Gaillard E, Mentink-Vigier F, et al. Direct 17O isotopic labeling of oxides using mechanochemistry. Inorg Chem 2020;59:13050-66.
131. Nagashima H, Trébosc J, Kon Y, Sato K, Lafon O, Amoureux JP. Observation of low-γ quadrupolar nuclei by surface-enhanced NMR spectroscopy. J Am Chem Soc 2020;142:10659-72.
132. Khan SU, Al-Shahry M, Ingler WB Jr. Efficient photochemical water splitting by a chemically modified n-TiO2. Science 2002;297:2243-5.
133. Kudo A, Miseki Y. Heterogeneous photocatalyst materials for water splitting. Chem Soc Rev 2009;38:253-78.
134. Zou Z, Ye J, Sayama K, Arakawa H. Direct splitting of water under visible light irradiation with an oxide semiconductor photocatalyst. Nature 2001;414:625-7.
135. Cortright RD, Davda RR, Dumesic JA. Hydrogen from catalytic reforming of biomass-derived hydrocarbons in liquid water. Nature 2002;418:964-7.
136. Fu Q, Saltsburg H, Flytzani-Stephanopoulos M. Active nonmetallic Au and Pt species on ceria-based water-gas shift catalysts. Science 2003;301:935-8.
137. Takata T, Jiang J, Sakata Y, et al. Photocatalytic water splitting with a quantum efficiency of almost unity. Nature 2020;581:411-4.
138. Liu Z, Huang E, Orozco I, et al. Water-promoted interfacial pathways in methane oxidation to methanol on a CeO2-Cu2O catalyst. Science 2020;368:513-7.
139. Saavedra J, Doan HA, Pursell CJ, Grabow LC, Chandler BD. The critical role of water at the gold-titania interface in catalytic CO oxidation. Science 2014;345:1599-602.
140. Merte LR, Peng G, Bechstein R, et al. Water-mediated proton hopping on an iron oxide surface. Science 2012;336:889-93.
141. Hussain H, Tocci G, Woolcot T, et al. Structure of a model TiO2 photocatalytic interface. Nat Mater 2017;16:461-6.
143. Lin L, Hisatomi T, Chen S, Takata T, Domen K. Visible-light-driven photocatalytic water splitting: recent progress and challenges. Trends Chem 2020;2:813-24.
144. Wang ZT, Wang YG, Mu R, et al. Probing equilibrium of molecular and deprotonated water on TiO2(110). Proc Natl Acad Sci U S A 2017;114:1801-5.
145. Du Y, Deskins NA, Zhang Z, Dohnálek Z, Dupuis M, Lyubinetsky I. Two pathways for water interaction with oxygen adatoms on TiO2(110). Phys Rev Lett 2009;102:096102.
146. Kristoffersen HH, Hansen JO, Martinez U, et al. Role of steps in the dissociative adsorption of water on rutile TiO2(110). Phys Rev Lett 2013;110:146101.
147. Kamal C, Stenberg N, Walle LE, et al. Core-level binding energy reveals hydrogen bonding configurations of water adsorbed on TiO2(110) surface. Phys Rev Lett 2021;126:016102.
148. Vittadini A, Selloni A, Rotzinger FP, Grätzel M. Structure and energetics of water adsorbed at TiO2 anatase 101 and 001 surfaces. Phys Rev Lett 1998;81:2954-7.
149. Tilocca A, Selloni A. Vertical and lateral order in adsorbed water layers on anatase TiO2(101). Langmuir 2004;20:8379-84.
150. Walle LE, Borg A, Johansson EMJ, et al. Mixed dissociative and molecular water adsorption on anatase TiO2 (101). J Phys Chem C 2011;115:9545-50.
151. Patrick CE, Giustino F. Structure of a water monolayer on the anatase TiO2(101) surface. Phys Rev Appl 2014;2:014001.
152. Fasulo F, Piccini G, Muñoz-garcía AB, Pavone M, Parrinello M. Dynamics of water dissociative adsorption on TiO2 anatase (101) at monolayer coverage and below. J Phys Chem C 2022;126:15752-8.
153. Boles MA, Ling D, Hyeon T, Talapin DV. The surface science of nanocrystals. Nat Mater 2016;15:141-53.
154. Zhang X, Qin J, Xue Y, et al. Effect of aspect ratio and surface defects on the photocatalytic activity of ZnO nanorods. Sci Rep 2014;4:4596.
155. Mueller DN, Machala ML, Bluhm H, Chueh WC. Redox activity of surface oxygen anions in oxygen-deficient perovskite oxides during electrochemical reactions. Nat Commun 2015;6:6097.
156. Llordés A, Wang Y, Fernandez-Martinez A, et al. Linear topology in amorphous metal oxide electrochromic networks obtained via low-temperature solution processing. Nat Mater 2016;15:1267-73.
157. Zandi O, Hamann TW. Determination of photoelectrochemical water oxidation intermediates on haematite electrode surfaces using operando infrared spectroscopy. Nat Chem 2016;8:778-83.
158. Feng N, Liu F, Huang M, et al. Unravelling the efficient photocatalytic activity of boron-induced Ti3+ species in the surface layer of TiO2. Sci Rep 2016;6:34765.
159. Setvín M, Aschauer U, Scheiber P, et al. Reaction of O2 with subsurface oxygen vacancies on TiO2 anatase (101). Science 2013;341:988-91.
160. Peng YK, Keeling B, Li Y, et al. Unravelling the key role of surface features behind facet-dependent photocatalysis of anatase TiO2. Chem Commun 2019;55:4415-8.
161. Peng YK, Ye L, Qu J, et al. Trimethylphosphine-assisted surface fingerprinting of metal oxide nanoparticle by 31P solid-state NMR: a zinc oxide case study. J Am Chem Soc 2016;138:2225-34.
162. Peng YK, Chou HL, Edman Tsang SC. Differentiating surface titanium chemical states of anatase TiO2 functionalized with various groups. Chem Sci 2018;9:2493-500.
163. Peng Y, Fu Y, Zhang L, et al. Probe-molecule-assisted NMR spectroscopy: a comparison with photoluminescence and electron paramagnetic resonance spectroscopy as a characterization tool in facet-specific photocatalysis. ChemCatChem 2017;9:155-60.
164. Peng YK, Hu Y, Chou HL, et al. Mapping surface-modified titania nanoparticles with implications for activity and facet control. Nat Commun 2017;8:675.
165. Zheng A, Liu SB, Deng F. 31P NMR chemical shifts of phosphorus probes as reliable and practical acidity scales for solid and liquid catalysts. Chem Rev 2017;117:12475-531.
166. Zheng A, Huang SJ, Liu SB, Deng F. Acid properties of solid acid catalysts characterized by solid-state 31P NMR of adsorbed phosphorous probe molecules. Phys Chem Chem Phys 2011;13:14889-901.
167. Yao Q, Zhang L, Huang D, et al. MAS NMR studies on the formation and structure of oxygen vacancy on the CeO2 {110} surface under a reducing atmosphere. J Phys Chem C 2023;127:13021-33.
168. Wu Y, Wang Y, Huang D, et al. Direct quantification of oxygen vacancy on the TiO2 surface by 31P solid-state NMR. Chem Catal 2023;3:100556.
169. Chu Y, Yu Z, Zheng A, et al. Acidic strengths of Brønsted and lewis acid sites in solid acids scaled by 31P NMR chemical shifts of adsorbed trimethylphosphine. J Phys Chem C 2011;115:7660-7.
170. Hu Y, Guo B, Fu Y, et al. Facet-dependent acidic and catalytic properties of sulfated titania solid superacids. Chem Commun 2015;51:14219-22.
171. Zhang H, Yu H, Zheng A, Li S, Shen W, Deng F. Reactivity enhancement of 2-propanol photocatalysis on SO42-/TiO2: insights from solid-state NMR spectroscopy. Environ Sci Technol 2008;42:5316-21.
172. Choi W, Termin A, Hoffmann MR. The role of metal ion dopants in quantum-sized TiO2: correlation between photoreactivity and charge carrier recombination dynamics. J Phys Chem 1994;98:13669-79.
173. Vamathevan V, Amal R, Beydoun D, Low G, Mcevoy S. Photocatalytic oxidation of organics in water using pure and silver-modified titanium dioxide particles. J Photoch Photobio A 2002;148:233-45.
174. He C, Yu Y, Hu X, Larbot A. Influence of silver doping on the photocatalytic activity of titania films. Appl Surf Sci 2002;200:239-47.
175. Jaegers NR, Wang Y, Hu JZ, Wachs IE. Impact of hydration on supported V2O5/TiO2 catalysts as explored by magnetic resonance spectroscopy. J Phys Chem C 2021;125:16766-75.
176. Stebbins JF. Aluminum substitution in rutile titanium dioxide: new constraints from high-resolution 27Al NMR. Chem Mater 2007;19:1862-9.
177. Chen X, Mao SS. Titanium dioxide nanomaterials: synthesis, properties, modifications, and applications. Chem Rev 2007;107:2891-959.
178. Rumaiz AK, Woicik JC, Cockayne E, Lin HY, Jaffari GH, Shah SI. Oxygen vacancies in N doped anatase TiO2: experiment and first-principles calculations. Appl Phys Lett 2009;95:262111.
179. Cronemeyer DC. Infrared absorption of reduced rutile TiO2 single crystals. Phys Rev 1959;113:1222-6.
180. Tai Z, Sun G, Wang T, Li Z, Tai J. Netted C-doped TiO2 mesoporous nanostructure decorated by Cu nanoparticles for photocatalytic CO2 reduction. ACS Appl Nano Mater 2022;5:18070-9.
181. Dong Y, Luo X, Wang Y, et al. A robust novel 0D/2D MoS3 QDs/C-doped atomically thin TiO2(B) nanosheet composite for highly efficient photocatalytic H2 evolution. Appl Surf Sci 2022;599:153972.
182. Li Y, Ren Z, Gu M, Duan Y, Zhang W, Lv K. Synergistic effect of interstitial C doping and oxygen vacancies on the photoreactivity of TiO2 nanofibers towards CO2 reduction. Appl Catal B Environ 2022;317:121773.
183. Shayegan Z, Haghighat F, Lee C. Carbon-doped TiO2 film to enhance visible and UV light photocatalytic degradation of indoor environment volatile organic compounds. J Environ Chem Eng 2020;8:104162.
184. Yang Y, Liu L, Qi Q, et al. A low-cost and stable Fe2O3/C-TiO2 system design for highly efficient photocatalytic H2 production from seawater. Catal Commun 2020;143:106047.
185. Noorimotlagh Z, Kazeminezhad I, Jaafarzadeh N, Ahmadi M, Ramezani Z. Improved performance of immobilized TiO2 under visible light for the commercial surfactant degradation: role of carbon doped TiO2 and anatase/rutile ratio. Catal Today 2020;348:277-89.
186. Lettmann C, Hildenbrand K, Kisch H, Macyk W, Maier WF. Visible light photodegradation of 4-chlorophenol with a coke-containing titanium dioxide photocatalyst. Appl Catal B Environ 2001;32:215-27.
187. Ohno T, Tsubota T, Nishijima K, Miyamoto Z. Degradation of methylene blue on carbonate species-doped TiO2 photocatalysts under visible light. Chem Lett 2004;33:750-1.
188. Xu C, Killmeyer R, Gray ML, Khan SU. Photocatalytic effect of carbon-modified n-TiO2 nanoparticles under visible light illumination. Appl Catal B Environ 2006;64:312-7.
189. Xu C, Shaban YA, Ingler WB, Khan SU. Nanotube enhanced photoresponse of carbon modified (CM)-n-TiO2 for efficient water splitting. Sol Energy Mater Sol Cells 2007;91:938-43.
190. Liu F, Feng N, Yang L, Wang Q, Xu J, Deng F. Enhanced photocatalytic performance of carbon-coated TiO2-x with surface-active carbon species. J Phys Chem C 2018;122:10948-55.
191. Chen C, Long M, Zeng H, et al. Preparation, characterization and visible-light activity of carbon modified TiO2 with two kinds of carbonaceous species. J Mol Catal A Chem 2009;314:35-41.
192. Rockafellow EM, Fang X, Trewyn BG, Schmidt-rohr K, Jenks WS. Solid-state 13C NMR characterization of carbon-modified TiO2. Chem Mater 2009;21:1187-97.
193. Feng N, Lin H, Deng F, Ye J. Interfacial-bonding Ti–N–C boosts efficient photocatalytic H2 evolution in close coupling g-C3N4/TiO2. J Phys Chem C 2021;125:12012-8.
194. Feng G, Mao J, Sun T, et al. Nitrogen-doped titanium dioxide for selective photocatalytic oxidation of methane to oxygenates. ACS Appl Mater Interfaces 2024;16:4600-5.
195. Bhowmick S, Saini CP, Santra B, et al. Modulation of the work function of TiO2 nanotubes by nitrogen doping: implications for the photocatalytic degradation of dyes. ACS Appl Nano Mater 2023;6:50-60.
196. Chen C, Wu M, Yang C, et al. Electron-donating N-–Ti3+–Ov interfacial sites with high selectivity for the oxidation of primary C–H bonds. Cell Rep Phys Sci 2022;3:100936.
197. Kwon J, Choi K, Schreck M, Liu T, Tervoort E, Niederberger M. Gas-phase nitrogen doping of monolithic TiO2 nanoparticle-based aerogels for efficient visible light-driven photocatalytic H2 production. ACS Appl Mater Interfaces 2021;13:53691-701.
198. Liang M, Bai X, Yu F, Ma J. A confinement strategy to in-situ prepare a peanut-like N-doped, C-wrapped TiO2 electrode with an enhanced desalination capacity and rate for capacitive deionization. Nano Res 2021;14:684-91.
199. Kong X, Peng Z, Jiang R, et al. Nanolayered heterostructures of N-doped TiO2 and N-doped carbon for hydrogen evolution. ACS Appl Nano Mater 2020;3:1373-81.
200. Reyes-garcia EA, Sun Y, Reyes-gil K, Raftery D. 15N solid state NMR and EPR characterization of N-doped TiO2 photocatalysts. J Phys Chem C 2007;111:2738-48.
201. Feng N, Zheng A, Wang Q, et al. Boron environments in B-doped and (B, N)-codoped TiO2 photocatalysts: a combined solid-state NMR and theoretical calculation study. J Phys Chem C 2011;115:2709-19.
202. Feng N, Wang Q, Zheng A, et al. Understanding the high photocatalytic activity of (B, Ag)-codoped TiO2 under solar-light irradiation with XPS, solid-state NMR, and DFT calculations. J Am Chem Soc 2013;135:1607-16.
203. Zhao W, Ma W, Chen C, Zhao J, Shuai Z. Efficient degradation of toxic organic pollutants with Ni2O3/TiO2-xBx under visible irradiation. J Am Chem Soc 2004;126:4782-3.
204. Reyes-garcia EA, Sun Y, Raftery D. Solid-state characterization of the nuclear and electronic environments in a boron−fluoride co-doped TiO2 visible-light photocatalyst. J Phys Chem C 2007;111:17146-54.
205. Wu T, Xie Y, Yin L, Liu G, Cheng H. Switching photocatalytic H2 and O2 generation preferences of rutile TiO2 microspheres with dominant reactive facets by boron doping. J Phys Chem C 2015;119:84-9.
206. Liu G, Zhao Y, Sun C, Li F, Lu GQ, Cheng HM. Synergistic effects of B/N doping on the visible-light photocatalytic activity of mesoporous TiO2. Angew Chem Int Ed Engl 2008;47:4516-20.
207. Gopal NO, Lo HH, Ke SC. Chemical state and environment of boron dopant in B,N-codoped anatase TiO2 nanoparticles: an avenue for probing diamagnetic dopants in TiO2 by electron paramagnetic resonance spectroscopy. J Am Chem Soc 2008;130:2760-1.
208. In S, Orlov A, Berg R, et al. Effective visible light-activated B-doped and B,N-codoped TiO2 photocatalysts. J Am Chem Soc 2007;129:13790-1.
209. Zaleska A, Sobczak JW, Grabowska E, Hupka J. Preparation and photocatalytic activity of boron-modified TiO2 under UV and visible light. Appl Catal B Environ 2008;78:92-100.
210. Coudurier G, Auroux A, Vedrine JC, Farlee RD, Abrams L, Shannon RD. Properties of boron-substituted ZSM-5 and ZSM-11 zeolites. J Catal 1987;108:1-14.
211. de Ruiter R, Kentgens A, Grootendorst J, Jansen J, van Bekkum H. Calcination and deboronation of [B]-MFI single crystals. Zeolites 1993;13:128-38.
212. Dorn RW, Heintz PM, Hung I, et al. Atomic-level structure of mesoporous hexagonal boron nitride determined by high-resolution solid-state multinuclear magnetic resonance spectroscopy and density functional theory calculations. Chem Mater 2022;34:1649-65.
213. Mark LO, Dorn RW, Mcdermott WP, et al. Highly selective carbon-supported boron for oxidative dehydrogenation of propane. ChemCatChem 2021;13:3611-8.
214. Medek A, Harwood JS, Frydman L. Multiple-quantum magic-angle spinning NMR: a new method for the study of quadrupolar nuclei in solids. J Am Chem Soc 1995;117:12779-87.
215. Wang SH, Xu Z, Baltisberger JH, Bull LM, Stebbins JF, Pines A. Multiple-quantum magic-angle spinning and dynamic-angle spinning NMR spectroscopy of quadrupolar nuclei. Solid State Nucl Magn Reson 1997;8:1-16.
216. Smith ME, van Eck ERH. Recent advances in experimental solid state NMR methodology for half-integer spin quadrupolar nuclei. Prog Nucl Mag Res Sp 1999;34:159-201.
217. Dorn RW, Cendejas MC, Chen K, et al. Structure determination of boron-based oxidative dehydrogenation heterogeneous catalysts with ultra-high field 35.2 T 11B solid-state NMR spectroscopy. ACS Catal 2020;10:13852-66.
218. Sasaki J, Peterson N, Hoshino K. Tracer impurity diffusion in single-crystal rutile (TiO2-x). J Phys Chem Solids 1985;46:1267-83.
219. Bak T, Burg T, Kang S, et al. Charge transport in polycrystalline titanium dioxide☆. J Phys Chem Solids 2003;64:1089-95.
220. Gesenhues U. Al-doped TiO2 pigments: influence of doping on the photocatalytic degradation of alkyd resins. J Photoch Photobio A 2001;139:243-51.
221. Kotzamanidi S, Frontistis Z, Binas V, Kiriakidis G, Mantzavinos D. Solar photocatalytic degradation of propyl paraben in Al-doped TiO2 suspensions. Catal Today 2018;313:148-54.
222. Murashkina AA, Rudakova AV, Ryabchuk VK, et al. Influence of the dopant concentration on the photoelectrochemical behavior of Al-doped TiO2. J Phys Chem C 2018;122:7975-81.
223. Gionco C, Livraghi S, Maurelli S, et al. Al- and Ga-doped TiO2, ZrO2, and HfO2: the nature of O 2p trapped holes from a combined electron paramagnetic resonance (EPR) and density functional theory (DFT) study. Chem Mater 2015;27:3936-45.
224. Su CY, Wang LC, Liu WS, Wang CC, Perng TP. Photocatalysis and hydrogen evolution of Al- and Zn-doped TiO2 nanotubes fabricated by atomic layer deposition. ACS Appl Mater Interfaces 2018;10:33287-95.
225. Yang L, Feng N, Deng F. Aluminum-doped TiO2 with dominant {001} facets: microstructure and property evolution and photocatalytic activity. J Phys Chem C 2022;126:5555-63.
226. Xu J, Wang Q, Li S, Deng F. Solid-state NMR in zeolite catalysis. 1st edition. Singapore: Springer. 2019.
Cite This Article

How to Cite
Download Citation
Export Citation File:
Type of Import
Tips on Downloading Citation
Citation Manager File Format
Type of Import
Direct Import: When the Direct Import option is selected (the default state), a dialogue box will give you the option to Save or Open the downloaded citation data. Choosing Open will either launch your citation manager or give you a choice of applications with which to use the metadata. The Save option saves the file locally for later use.
Indirect Import: When the Indirect Import option is selected, the metadata is displayed and may be copied and pasted as needed.
About This Article
Special Issue
Copyright
Author Biographies
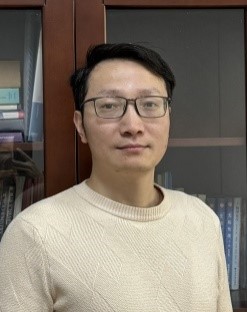
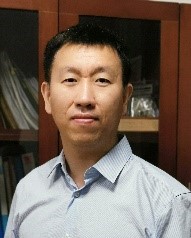
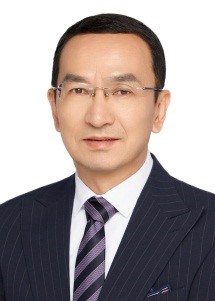
Comments
Comments must be written in English. Spam, offensive content, impersonation, and private information will not be permitted. If any comment is reported and identified as inappropriate content by OAE staff, the comment will be removed without notice. If you have any queries or need any help, please contact us at [email protected].