Current knowledge about immunotherapy resistance for melanoma and potential predictive and prognostic biomarkers
Abstract
Melanoma still reaches thousands of new diagnoses per year, and its aggressiveness makes recovery challenging, especially for those with stage III/IV unresectable melanoma. Immunotherapy, emerging as a beacon of hope, stands at the forefront of treatments for advanced melanoma. This review delves into the various immunotherapeutic strategies, prominently featuring cytokine immunotherapy, adoptive cell therapy, immune checkpoint inhibitors, and vaccinations. Among these, immune checkpoint inhibitors, notably anti-programmed cell death-1 (PD-1) and anti-cytotoxic T lymphocyte antigen-4 (CTLA-4) antibodies, emerge as the leading strategy. However, a significant subset of melanoma patients remains unresponsive to these inhibitors, underscoring the need for potent biomarkers. Efficient biomarkers have the potential to revolutionize the therapeutic landscape by facilitating the design of personalized treatments for patients with melanoma. This comprehensive review highlights the latest advancements in melanoma immunotherapy and potential biomarkers at the epicenter of recent research endeavors.
Keywords
INTRODUCTION
Melanoma, recognized as an aggressive form of skin cancer, represents a substantial challenge to both patients and clinicians due to its propensity for relapse and metastasis[1]. In 2024, it is anticipated that there will be 100,640 new cases of melanoma in the United States, with an estimated 8,290 deaths[2]. In contrast, melanoma in China is relatively rare, leading to delayed diagnoses and treatments. Statistical reports from 2022 indicate approximately 8,800 new cases and 5,400 deaths in China, accounting for 61% of the cases[3]. Meantime, melanoma has emerged as the fifth most prevalent cancer in the European Union (EU) since 2020, representing 4% of new cancer cases and 1.3% of all cancer deaths. Notably, the mortality rate in men exceeds that in women[4]. The factor that is considered to be the most common cause of melanoma cancer is thought to be ultraviolet rays (UV)[5]. UV exposure causes DNA mutations in protective melanocytes[6]. Conventional chemotherapy has historically offered limited success, with a response rate of merely 10% and brief survival periods[7]. However, a groundbreaking shift occurred with the advent of immune checkpoint inhibitors (ICIs), which have revolutionized the prognosis for melanoma and various skin cancers. Immunotherapy has emerged as the standard adjuvant therapy for patients with high-risk resected stage III or IV melanoma, and its promising results extend to diverse skin cancer types[8,9]. The objective response rate (ORR) for immunotherapy treatment for melanoma has increased from 16% during the period of high-dose interleukin (IL)-2 therapy to 61% with the combination treatment of anti-programmed cell death-1 (PD-1) antibody Nivolumab and anti-cytotoxic T lymphocyte antigen-4 (CTLA-4) antibody Ipilimumab[10,11]. This improvement is attributed to the complex interplay of immune escape mechanisms, driven by the heterogeneity of melanoma cells both within and across tumors[12,13]. Furthermore, the genetic mutations resulting from this cellular heterogeneity may have clinical significance as promising biomarkers. This is because such specific mutations reflect the tumor’s characteristics of immune escape and response to treatment, thus providing a basis for personalized therapeutic strategies. Consequently, the urgent need to identify specific biomarkers that predict immunotherapy outcomes in melanoma and skin cancer patients has spurred intensive research in this field[14]. This review aims to provide a comprehensive overview of immunotherapy for melanoma, exploring its applications, benefits, mechanisms of resistance, and associated molecular biomarkers. We highlight the latest developments and future perspectives in melanoma research, aiming to offer insights into the evolving landscape of treatment strategies.
STRATEGIES AND ADVANCES OF IMMUNOTHERAPY IN MELANOMA
Immunotherapy, a revolutionary treatment approach that harnesses the body’s immune system to combat cancer, has emerged as a transformative breakthrough in the management of skin cancers, particularly melanoma. Unlike traditional treatments like chemotherapy, which directly attack cancer cells, immunotherapy works by unleashing the power of the immune system to recognize and eliminate cancerous cells[15,16]. This innovative approach encompasses various strategies, such as immune checkpoint inhibitors, cytokine immunotherapy, adoptive cell therapy, and vaccinations. These techniques have demonstrated remarkable success in melanoma, with immune checkpoint inhibitors like anti-PD-1 and anti-CTLA-4 antibodies standing out due to their efficacy. Furthermore, adoptive cell therapy and tumor vaccinations also promise to expand treatment options. Overall, these advancements in immunotherapy offer new hope for patients who previously faced limited treatment options and grim prognoses.
ICIs
ICIs represent a significant milestone in melanoma treatment, substantially improving the survival rates of patients. In 1996, the concept of immune checkpoint blocking was first introduced. Currently, anti-PD-1 antibodies and anti-CTLA-4 antibodies are among the most widely used immune checkpoint inhibitors, providing significant therapeutic benefits from either monotherapy or combination[17-19].
PD-1/programmed cell death ligand 1 (PD-L1) interaction involves a ligand and receptor between tumor cells and T cells. The ligands PD-L1/PD-L2 on the surface of tumor cells specifically bind to the PD-1 receptor on the surface of T cells. This binding inhibits the immune recognition of tumor cells by T cells and causes immune escape[20,21]. Nivolumab and Pembrolizumab are FDA-approved anti-PD-1 monoclonal antibodies (mAb) used to treat melanoma. In July 2014, Nivolumab was approved in Japan for treating advanced melanoma, becoming the world’s first PD-1 inhibitor to receive regulatory approval. Pembrolizumab, developed by Merck Sharp & Dohme for treating unresectable or metastatic melanoma following ipilimumab therapy, effectively boosts the body’s immune response. They have been widely adopted in clinical practice, and there is a growing trend toward combining them with multiple drugs to enhance drug sensitivity or act as adjuvant or neoadjuvant therapy[22,23]. The results of a clinical trial using Relatlimab (LAG-3 inhibitor) and Nivolumab for neoadjuvant therapy in thirty patients with stage III resectable melanoma or single-metastatic stage IV melanoma showed that 57% of patients had complete remission and 70% had a partial response. This combination was well-tolerated during neoadjuvant therapy, although some toxicity was observed during adjuvant therapy[24].
A retrospective study of blood sample analysis in patients with stage IIIb-IV melanoma after three months of combined therapy with cetirizine and anti-PD-1 antibodies showed that combining anti-PD-1 mAb with cetirizine can significantly improve the progression-free survival (PFS) (28 vs. 15 months; 30 vs. 15 months in ICI-treated patients) and overall survival (OS) (36 vs. 23 months; 40 vs. 22 months in ICI-treated patients) of patients who had received other ICI treatments[25]. Anti-PD-1 antibodies have also been used to treat different cancers, such as non-small cell lung cancer. Research demonstrated through meta-analysis that chemotherapy combined with anti-PD-1 antibody was superior to combination therapy with anti-PD-L1 antibody, with better OS (P = 0.022) and PFS (P = 0.029)[26].
CTLA-4 is a transmembrane receptor on T cells, which modulates the immune response of T cells by binding to antigen-presenting cell surface receptors. Blocking CTLA-4 mAb can lead to a surge in T cell proliferation and their subsequent attack on tumor cells[27,28]. In 2011, the FDA approved Ipilimumab to treat unresectable advanced melanoma, with 5-year survival rates for naïve advanced melanoma patients treated with the ipilimumab monotherapy reported between 21.4% and 49.5%[29]. As adjuvant therapy for stage III melanoma after resection, ipilimumab has shown a 5-year recurrence-free survival rate of 40.8% and an OS of 65.4% at five years[30-32].
In 2023, the American Society of Clinical Oncology (ASCO) issued an update to the guidelines for the systemic treatment of melanoma patients, introducing new recommendations for neoadjuvant pembrolizumab for patients with resectable stage IIIB-IV cutaneous melanoma, adjuvant nivolumab or pembrolizumab for stage IIB-C disease, and adjuvant nivolumab plus ipilimab as a potential option for stage IV disease. For patients with unresectable or metastatic cutaneous melanoma, Nivolumab + Relatlimumab (LAG-3 inhibitor ) was added as a potential option, regardless of BRAF mutation status, and nivolumab + ipilimumab plus nivolumab was highlighted as superior to BRAF/MEK inhibitor therapy in the first-line treatment of patients with BRAF-mutated disease[33].
Cytokine immunotherapy
Cytokine immunotherapy, rooted in the principle of modulating the body’s innate immune responses against malignancies, has been pivotal in reshaping the treatment landscape for melanoma. Among the array of treatments, interferons (IFNs) and high-dose IL-2 have traditionally been at the forefront of melanoma immunotherapy[34,35]. Specifically, high-dose IL-2 and interferon-alpha have been approved by the FDA as immunotherapy drugs for melanoma[36]. In addition, cytokines such as IL-10, IL-12, IL-15, IL-18, and IL-21 have been proven to mobilize immune regulation in treating other cancers[37,38]. These cytokines are often described as hormones, which play dual roles in regulating the immune system’s response against tumor cells and directly inducing cancer cell apoptosis, cell cycle arrest, and angiogenesis inhibition[39]. For instance, IFN-alpha is known to enhance the cytotoxicity and the antitumor ability of NK cells and strengthen CD8+ cytotoxic T lymphocytes (CTLs)[40]. Interferons have been used as adjuvant therapy for melanoma for many years, and the first clinical trial of IFN-2b as an adjuvant therapy that significantly improves high-risk melanoma OS and recurrence-free survival increased disease-free survival to 1.7 years and OS to 3.8 years[41]. Notably, IFN-alpha-1b, the first genetically engineered recombinant drug approved by the China Food and Drug Administration, has been proven effective in prolonging the survival of melanoma patients[42].
In East Asia, where melanoma constitutes a smaller percentage of cancer cases and often goes undetected until advanced stages, the response rate to immune checkpoint inhibitors is notably lower. Combining ICIs with IFN has been explored to enhance response rates and therapeutic effects. A retrospective study evaluating the combination of IFN-alpha-1b and anti-PD-1 mAB in untreated stage IV melanoma patients observed a 32.8% objective response rate and 18-month overall survival. While the combination therapy was associated with a high rate of adverse events, less than 10% of patients experienced severe (grade ¾) adverse effects, significantly lower than those observed with the combination of ICI and IFN-alpha-2b[43,44]. The therapeutic landscape of melanoma is continuously evolving, with ongoing clinical trials and research focusing on harnessing the full potential of cytokine immunotherapy. The convergence of these therapies with other approaches, such as immune checkpoint inhibitors, offers hope for more tailored and effective treatment strategies for melanoma patients.
Adoptive cell therapy
Adoptive cell therapy (ACT) represents a paradigm shift in cancer treatment, harnessing the patient’s immune cells for therapeutic purposes. This strategy offers the promise of a tailored approach, especially for patients who are refractory to traditional treatments. ACT is centered on manipulating a patient’s immune cells, amplifying their tumor-fighting capabilities, and then reintroducing them to the body to target and destroy cancer cells. The two primary types of ACT that have garnered attention in melanoma treatment are tumor-infiltrating lymphocyte (TIL) therapy and chimeric antigen receptor (CAR) T-cell therapy[45-47]. TIL therapy capitalizes on the presence of immune cells within the tumor microenvironment. By isolating these cells and stimulating their proliferation ex vivo, typically using agents like IL-2, clinicians can reinfuse a substantial number (around 1011) of these primed cells into the patient[48]. Historically, TIL’s debut in cancer treatment traces back to the 1980s, and its efficacy, as evidenced by an ORR of 41% in melanoma, attests to its potential[49]. On the other hand, CAR T-cell therapy involves genetic modification of T cells to express receptors specifically engineered to target tumor-associated antigens. This tailored recognition facilitates the precise targeting of cancer cells, paving the way for innovative therapeutic strategies.
To harness the full potential of TIL for personalized immunotherapy, ongoing research seeks to unearth a broader spectrum of antigens, encompassing tumor-associated, tumor-specific, and unconventional antigens, which include those antigens originate from non-coding regions of the genome or are produced through aberrant transcription, translation, or post-translational modification processes, from coding regions, whether wild-type or mutated[50]. This is particularly pivotal for patients demonstrating resistance or treatment failure with ICIs[51]. Notably, melanoma patients with BRAF and NRAS mutations who have failed to respond optimally to agents like anti-PD-1 or anti-CTLA-4 antibodies have exhibited either partial response or long-stable disease (SD) after receiving TIL treatment[52].
In February 2024, Lifileucel received accelerated approval from the U.S. FDA, becoming the first approved TIL therapy to treat solid tumors. Lifileucel’s definitive clinical trial (NCT02360579) is an open-label, multi-cohort, multi-center Phase 2 clinical trial in patients with advanced melanoma who have progressed on or after PD-1/PD-L1 inhibitors. Based on the trial results, Lifileucel demonstrated significant clinical benefit, with an ORR of 31.4% as assessed by an independent review committee (IRC) at a median follow-up of 27.6 months, including patients with complete response (CR) and partial response (PR), and the most prolonged duration of response was 55.8 months[53]. The latest clinical trial results confirmed its efficacy in non-small cell lung cancer. In the Phase 2 clinical trial in patients with metastatic non-small cell lung cancer, Lifileucel demonstrated an objective response rate of 21.4% in patients who had undergone prior immunotherapy and observed responses in some refractory tumor types, despite some manageable adverse events and individual patient deaths due to treatment-related adverse events[54].
However, the promise of ACT comes with challenges. The intricate process of cell extraction, modification, expansion, and reinfusion is time-consuming and costly. Before patients receive TIL infusions, a brief non-myeloablative (NMA) lymphodepletion regimen, usually chemotherapy, IL-2, or systemic radiation therapy, is required. This is so that after receiving the TIL infusion, lymphocyte deletion stimulates the body to enhance the effect of TIL[55]. Though it is an essential process, chemotherapy and IL-2 therapy are the most toxic parts of the treatment process. However, TILs that can generate autoimmune stimulation signals are being developed so that patients no longer need to be treated with IL-2, thereby reducing adverse effects. As the realm of ACT continues to evolve, the horizon is replete with opportunities from refining cell preparation techniques to exploring combinations with other therapeutic modalities. The journey toward optimizing ACT for melanoma patients is well underway.
Vaccination for melanoma
Cancer vaccines are an alternative immunotherapy option for patients who tolerate ICIs. Cancer vaccines come in different forms with varying antigen types and contents, including peptide vaccines, whole cell vaccines, dendritic cell vaccines, neoantigen vaccines, nucleic acid (DNA, RNA) vaccines, and protein vaccines, among others[56-59]. Their ability to elicit an immune response against tumors makes them a viable alternative, especially for patients who might be tolerant or refractory to ICIs. A recent clinical trial of an immune-modulatory vaccine activates IDO/PD-L1 specific T cells, targets cancer cells by IDO- and PD-L1-specific CD8+ T cells, and releases pro-inflammatory cytokines by CD4+ T cells. The strategy combined with anti-PD-1 antibody showed 62.7%-90.5% of ORR with 27.4%-60.8% of complete responses[60].
With deepening the understanding of tumor biology and immunology, personalized vaccines are becoming a research hotspot. These vaccines are tailored to the patient’s tumor characteristics and genetic background to improve the targeting and effectiveness of the vaccine. In clinical trial NCT01970358, eight patients with surgically resected IIIB/C or IVM1a/b melanoma obtained persistent cancer control after receiving personal neoantigen vaccination, NeoVax, a peptide vaccine targeting up to 20 antigens per patient. The peptide vaccine was designed by comparing whole-exome sequencing of tumor cells and normal cells of individual patients to find mutant peptides that can bind to HLA-A and HLA-B. NeoVax-induced T cells can specifically target melanoma and exhibit memory phenotype[61,62]. Another clinical trial for the liposomal RNA (RNA-LPX) vaccine, Fixvac, which encodes four tumor-associated antigens (TAAs) - New York oesophageal squamous cell carcinoma 1 (NY-ESO-1), melanoma-associated antigen A3 (MAGE-A3), tyrosinase, and transmembrane phosphatase with tensin homology (TPTE), showed a great response when vaccinated alone or combined with checkpoint inhibitors for ICI-experienced patients. Fixvac increased the release of cytokines, such as IFN-α, IFN-γ, IL-6, IFN-inducible protein (IP)-10, and IL-12 p70 subunit in plasma at a relatively low concentration in humans compared to what was required to achieve such immune outcomes in mice[57]. Another neoantigen-based vaccine, NEO-PV-01, combined with or without anti-PD-1 antibody in an Ib clinical trial of 60 patients who received the vaccine, ORR was 59% (39%-78%), 39% (17%-64%), and 27% (8%-55%) in melanoma, non-small cell lung cancer (NSCLC), and bladder cancer patients, respectively. No significant adverse effects were found[63]. Another class of cancer vaccines that can specifically target cancer stem cells (CSCs) is also one of the current research hotspots. Recent CSC vaccine research on murine melanoma stem cell (MSCs)-based vaccine was an IL-33 modified MSCs vaccine that could target the B16F10-CD44+CD133+ cells. Meanwhile, dendritic cells would mature after the vaccine is received and trigger CTL responses, together with the activation of the CD8+ T cells, which are the effector against tumors[64].
Here, we have to mention a clinical trial of the mRNA vaccine in combination with pembrolizumab for treating tumors initiated by Moderna Inc. and Merck Sharp & Dohme LLC. Moderna developed mRNA-4157 (V940), an mRNA neoantigen tailored to the patient’s specific tumor DNA sequence to stimulate the body’s immune response. In a phase II trial of the combination, in patients with completely resected melanoma stage III and IV melanoma, the combination with mRNA-4157 reduced the risk of recurrence and death by 44% compared to Pembrolizumab alone. The 18-month recurrence-free survival (RFS) rate was 78.6% (combination) vs. 62.2% (pembrolizumab), with 18-month distant metastasis-free survival (DMFS) rates being 91.8% (combination) vs. 76.8% (pembrolizumab), respectively (NCT03897881)[65]. This promising outcome has now entered a phase 3 clinical trial (NCT05933577).
Thanks to the intersection of computer science and biotechnology, the invention of tumor vaccines has become more accessible. With big data and computer algorithms, it is possible to compute and design tumor vaccines formed by combinations of multiple antigens. Yazdani et al. developed a novel multiepitope peptide vaccine by combining computerized bioinformatics, molecular docking, and dynamic stimulation assessments that combine three immunogenic proteins, NYESO-1, gp-100, and MART-1, with multiple helper epitopes as adjuvants[59]. Although the actual effect of this vaccine against melanoma still needs to be proved by further experiments, it shows us the application and future trend of computers in vaccine design. Another research on melanoma vaccine described a strategy for cancer immunotherapy based on DNA nanodevices. The researchers designed and fabricated a DNA robotic nanostructure that effectively protects antigens and adjuvants in vivo and transports them to draining lymph nodes (dLNs). Within dLNs, the nanodevice can respond to and release antigens and adjuvants in an acidic environment, activate dendritic cells, and elicit a robust, tumor-specific CD8+ CTL response via the Toll-like receptor (TLR) pathway and antigenic peptide presentation[58]. Although this research is murine-based, it is worth mentioning that it has demonstrated the potential of DNA nanodevice vaccines in cancer immunotherapy.
Bispecific antibodies
Bispecific antibodies (bsAbs) are artificially designed antibodies that can specifically bind to two antigens or epitopes simultaneously. BsAbs are a new frontier in tumor immunotherapy in the 21st century. These antibodies can directly connect T cells to tumor cells, enhancing the immune system’s attack on tumors. Compared with monospecific antibodies, bispecific antibodies have more robust specificity and targeting, which can reduce off-target toxicity. There have been a few bsAbs approved by the FDA today. Blinatumomab from Amgen is the first approved bispecific antibody for the treatment of adult relapsed or refractory precursor B-cell acute lymphoblastic leukemia (ALL)[66]; amivantamab from Johnson&Johnson is a bsAb for EGFR-positive metastatic NSCLC[67]; Tebentafusp is an FDA-approved bispecific fusion protein by Immunocore in 2022 for the treatment of HLA-A*02:01-positive patients with unresectable or metastatic uveal melanoma. In the open-label, phase 3 trial (NCT03070392) of Tebentafusp, the OS of patients who received Tebentafusp at 1 year was 73% and 36% of PFS at 6 months, both significantly higher than the control group[68]. In the latest clinical trial results (NCT03070392), the 3-year median OS was 21.6 months, significantly higher than the control group’s 16.9 months, demonstrating the long-term benefits of Tebentafusp for patients[69].
Combination immunotherapy
Combination immunotherapy, also known as cocktail immunotherapy or combination therapy, involves the simultaneous or sequential use of multiple immunotherapeutic agents to enhance the anti-cancer immune response. Combination immunotherapy aims to improve treatment efficacy, overcome resistance mechanisms, and achieve better outcomes for patients with various types of cancer, including melanoma. The rationale behind combination immunotherapy is to exploit different aspects of the immune response and the tumor microenvironment to achieve a more potent and sustained anti-cancer effect. A clinical trial (NCT03470922) demonstrated a combination immunotherapy of Relatlimab (LAG-3-blocking antibody)-nivolumab (PD-1-blocking antibody)[70]. The combination showed a 10.1-month PFS, while the progression-free survival of nivolumab alone was 4.6 months.
It is important to note that the specific combination therapies used in clinical practice may vary depending on the type and stage of cancer and the individual patient’s characteristics. Therefore, careful patient selection and close monitoring are essential to manage potential adverse events. However, to maximize the efficacy of combined therapy, it is more important to use the correct order of different therapeutic drugs, especially when both immunotherapy and targeted therapy can significantly improve the patient’s OS. The DREAMseq stage III trial answers this question (NCT02224781). Patients with advanced BRAFV600-mutant metastatic melanoma were involved in this 2-step clinical trial to compare the effect of different dosing sequences on treatment efficacy[71]. Out of a total of 265 patients, only 73 patients went onto the step 2 trial. Of these, 27 patients continued to receive Dabrafenib (BRAF inhibitor) and Trametinib (MEK inhibitor) after first receiving Nivolumab and Ipilimumab. In comparison, 46 patients received Dabrafenib and Trametinib first, then Nivolumab and Ipilimumab. The 2-year OS of the former was 71.8% (95%CI, 62.5 to 79.1), while the 2-year OS of the latter was 51.5% (95%CI, 41.7 to 60.4). The DREAMseq trial confirmed that preferred nivolumab/ipilimumab followed by BRAF and MEK inhibitor therapy has better control of melanoma, providing a practical clinical treatment reference.
In therapy widely used with anti-PD-1 monoclonal antibodies, especially for patients with stage III/IV melanoma, the combined treatment of Nivolumab and Ipilimumab has significantly increased patient response rates. In a randomized clinical trial of patients with untreated stage III/IV melanoma, patients were assigned to receive initial treatment with nivolumab plus ipilimumab, followed by continued treatment with nivolumab and ipilimumab switching to placebo, or treatment with ipilimumab plus nivolumab placebo. At least five years of follow-up, the 5-year OS was 52% in the nivolumab plus ipilimumab combination group compared with the ipilimumab group, which was significantly higher than the 44% treated with nivolumab alone and the 26% treated with ipilimumab alone. These results suggest that the combination of nivolumab and ipilimumab demonstrated good long-term survival efficacy in this clinical trial[71,72]. For melanoma patients who have progressed to lymph node metastases or have both primary and metastatic lesions, Nivolumab plus ipilimumab may be an effective neoadjuvant treatment strategy to help control lesions and metastases, thereby providing patients with better surgical conditions. However, it is essential to note that this treatment strategy is not suitable for all patients and should be individualized on a case-by-case basis (NCT02437279, NCT02977052)[73]. A subsequent clinical trial, CheckMate-915, evaluated using Nivolumab combined with ipilimumab versus nivolumab alone as adjuvant therapy in patients with stage IIIB-D or IV melanoma resected. The results showed that in all randomized patient populations and patients with PD-L1 expression levels of less than 1%, nivolumab combined with ipilimumab did not improve RFS compared with nivolumab alone. In addition, the incidence of adverse events was higher in the combination treatment group[74].
Furthermore, patients who received anti-CTLA-4 antibodies prior and continued to receive anti-PD-1 antibodies had more tumor mutation burdens than patients who did not experience anti-CTLA-4 therapy, which means an increase in the number of non-synonymous mutations in the genome of tumor cells that may lead to the production of neoantigens that activate the immune system’s attack on tumors. Among them, the most significant mutation is PIK3C2G missense. This may be because anti-CTLA-4 therapy (a) increases the immune recognition of tumor cells, leading to tumor cell death and the release of neoantigens, which may contain mutation sites; (b) alters the tumor microenvironment, increases the inflammatory state of the tumor, and promotes the accumulation of mutations; (c) affects the biological properties of tumor cells, for example, by altering DNA repair mechanisms or cell cycle regulation, increasing mutation rate[75]. A phase 2 clinical trial (Checkmate-204; NCT02320058) showed that the Nivolumab/Ipilimumab combination is also effective in melanoma patients with brain metastasis. The clinical results showed that the 6-month PFS rate was 64.2% intracranial and 75.9%, respectively. The 9-month PFS rates were 59.5% and 70.4%, respectively[76]. Clinical trials are ongoing to explore new combinations and optimize existing ones, aiming to provide more effective treatment options for patients with various types of cancer.
MECHANISM OF RESISTANCE TO IMMUNOTHERAPY
While immunotherapy has significantly improved the treatment outcomes for many melanoma patients, not all patients respond to immunotherapy, and some may develop resistance over time. The underlying mechanisms for such disparities in treatment outcomes are intricate and multifaceted. Understanding these mechanisms is crucial for developing strategies to overcome immunotherapy resistance in melanoma and other cancers. Based on this knowledge of resistance, scientists are actively studying these processes to identify new targets and combination therapies that can enhance the effectiveness of immunotherapy and improve patient outcomes. A comprehensive depiction of the resistance mechanism of cancer immunotherapy can be found in Figure 1, offering readers a visual insight into these complex processes.
Figure 1. Mechanism of resistance to Immunotherapy. The tumor microenvironment of melanoma secretes various cytokines, chemokines, growth factors, and microRNAs that inhibit the function of immune cells or promote the recruitment of immunosuppressive cells. MDSC: Myeloid-derived suppressor cells; TSLP: thymic stromal lymphopoietin; Treg: regulatory T cell; IFN: interferon; ATRA: all-trans retinoic acid. The figure was created with Biorender (http://www.biorender.com).
Tumor microenvironment changes
The tumor microenvironment (TME) is a complex and dynamic ecosystem surrounding cancer cells. Within this environment, various cell types, including immune cells, fibroblasts, and blood vessels, interact with the tumor[77]. Melanoma cells can exploit this microenvironment to evade immune surveillance by secreting various soluble factors that create an immunosuppressive microenvironment. These factors may include cytokines, chemokines, and growth factors, as well as microRNAs that inhibit the function of immune cells or promote the recruitment of immunosuppressive cells. Additionally, the tumor microenvironment may be enriched with factors that induce the differentiation of immune cells into regulatory or tolerogenic phenotypes, further dampening the antitumor immune response. They may release chemical signals that attract immunosuppressive cells such as regulatory T cells (Tregs) and myeloid-derived suppressor cells (MDSCs). These suppressive immune cells create an immunosuppressive shield around the tumor, preventing the effective activation and functioning of antitumor immune cells[78-80].
Melanoma induces keratinocytes to generate cytokine thymic stromal lymphopoietin (TSLP), transmits signals through the TSLP receptor of DC, promotes the expression of GATA3+Treg, and inhibits the activity of IFN-γ and the proliferation of CD8+ T cells. In lung squamous cell carcinoma (LUSC), it has also been found that the tumor produces immunosuppression through high expression of anti-inflammatory factors, such as TGFβ and CCL18[81-84]. Studies have found that Treg can cooperate with cancer cells to suppress immune activity. Inactive TGFβ secreted by cancer cells into the TME is activated by the β8 chain of αvβ8 integrin (Itgβ8) secreted by Treg[85]. Activated TGFβ promotes Treg development and suppresses effector T cell cytotoxicity in mammals. Arkhypov et al. demonstrated that soluble heat-shock protein 90α (HSP90α) prompts monocytes to become MDSC via TLR4 signaling and PD-L1 expression, potentially offering a target for countering immunosuppression in melanoma and improving immune-based therapies[86]. It was observed in melanoma patients that ATRA and IFN-β induce the expression of CD38 in the tumor microenvironment after treatment with anti-PD-1 antibodies, which inhibit the function of CD8+ T cells through adenosine receptor signaling, which may lead to resistance to anti-PD-1 therapy[87].
MicroRNA (miRNA) plays a significant role in tumors by being secreted into the tumor microenvironment (TME) via exosomes to regulate the immune environment and facilitate immune escape. In one study, two miRNAs, hsa-miR-498 and hsa-miR-3187-3p, are found in melanoma exosomes and dampen immune responses by altering CD8+ T cell behavior. These miRNAs affect CD45 expression, a key component in T cell signaling, leading to reduced TNFα secretion. The study also identified 206 distinct miRNAs in melanoma exosomes and proposed a shared motif guiding miRNA sorting[88].
Metabolic changes in tumor cells and T cells also play an important role in immunotherapy resistance in melanoma. Tumor cells adapt to the microenvironment and evade immune surveillance by regulating their own metabolism. For example, tumor cells can undergo aerobic glycolysis, similar to the metabolic demands of effector T cells, leading to nutrient competition between the two[89]. In addition, metabolites such as lactate secreted by tumor cells can alter the TME and inhibit the metabolism and cytotoxicity of T cells, thereby affecting the effect of immunotherapy. The function of T cells in the tumor microenvironment is also affected by metabolic reprogramming. Studies have shown that the metabolic state of the tumor microenvironment can inhibit the metabolism and cytotoxicity of CD8+ T cells. For example, lactate secreted by tumor cells alters the metabolism of CD8+ T cells by inhibiting pyruvate carboxylase (PC) activity, weakening their cytolytic activity. In addition, succinic acid secretion in the tumor microenvironment and autocrine activation of succinate receptor 1 (SUCNR1) play an important role in maintaining the cytotoxicity of CD8+ T cells[90].
In addition to secreting various cytokines to regulate the TME, melanoma cells can upregulate immune checkpoint molecules, such as PD-L1, as a protective mechanism[91]. While the PD-1/PD-L1 pathway has been a primary target for immunotherapy, other immune checkpoints can be upregulated in melanoma cells. For example, T cell immunoglobulin and mucin domain 3 (TIM-3) and LAG-3 are additional inhibitory receptors expressed on T cells[92-94]. TIM-3 is a negative costimulatory molecule widely expressed in various immune cells. It mediates TIM-3+ T cell dysfunction and T cell exhaustion by binding to the ligand Gal-9, thereby inhibiting the antitumor immune response of T cells[95]. In the tumor microenvironment, especially in tumor-infiltrating T cells, co-expression of TIM-3 and LAG-3 correlates with the exhaustion status of T cells. T-cell function can be further suppressed when these receptors interact with their respective ligands on tumor cells. This redundancy in immune checkpoint pathways provides an alternative route for melanoma cells to evade immune destruction. As mentioned in the ICI section above, LAG-3 is a third-generation checkpoint, and outstanding achievements have been made in the research and development of its inhibitors. Hopefully, TIM-3 combined with LAG-3 blockade would also be an active treatment option.
Loss of immunity tracking and response
Immunotherapy relies on the ability of the immune system to recognize specific antigens present in cancer cells. These antigens act as flags, signaling the immune system to attack the tumor.
Antigen loss
Melanoma cells can evolve and undergo genetic changes that result in the loss or downregulation of these antigenic markers[96-98]. Consequently, the immune system loses its targets, and the tumor becomes “invisible” to the immune cells, rendering immunotherapy less effective. This phenomenon, known as antigen loss, is a significant hurdle in generating sustained antitumor immune responses[99].
Impaired antigen presentation
Antigen-presenting cells (APCs), particularly dendritic cells, play a crucial role in activating T cells by presenting tumor antigens to them[100,101]. This process can be disrupted in melanoma, leading to a reduced immune response. Melanoma cells may produce factors that inhibit dendritic cell maturation or migration to the lymph nodes, where they interact with T cells. Consequently, the presentation of tumor antigens to T cells is impaired, diminishing the chances of initiating a robust antitumor immune reaction[102].
T-cell exhaustion
T cells are critical players in the immune response against cancer. T cells become activated upon recognizing tumor antigens and attack the cancer cells. However, the prolonged exposure of T cells to the tumor microenvironment can lead to exhaustion, where they lose their effector functions and become less responsive to antigens[103]. This T cell exhaustion is driven by persistent antigen stimulation and the engagement of inhibitory receptors, such as PD-1 and CTLA-4. The interaction between PD-L1 on tumor cells and its receptor PD-1 on T cells leads to T cell exhaustion and immune tolerance, further aiding the tumor in evading immune attacks[104]. As a result, the exhausted T cells fail to mount a potent and sustained immune attack against the melanoma cells.
Role of tumor-infiltration lymphocytes
TILs are immune cells that have successfully penetrated the tumor microenvironment. High levels of TILs have been associated with better responses to immunotherapy. However, not all melanomas have significant TIL infiltration, which can limit the effectiveness of immunotherapy. Strategies to enhance TIL recruitment and activation are areas of ongoing research. For instance, high expression of ZEB1 is associated with a reduction in CD8+ T cell infiltration in human melanoma samples. By implementing an increase or decrease in ZEB1 function in a mouse model, the study found that ZEB1 regulates tumor growth by controlling the recruitment of CD8+ T cells in tumors. In addition, ZEB1 directly inhibits the secretion of chemokines that attract T cells, including CXCL10. These findings suggest that ZEB1 is an essential factor in regulating immune escape in melanoma, and strategies targeting ZEB1 may help improve the efficacy of immunotherapy[105].
While the immune system is adept at tracking and responding to melanoma, the tumor’s adaptive mechanisms can hinder this process. Understanding and countering these evasive tactics will be paramount in refining and enhancing immunotherapeutic approaches for melanoma.
Heterogeneity-based immune-escape
Melanoma is a genetically heterogeneous disease, and tumors can harbor various subpopulations with distinct genetic profiles. Some subpopulations may be less susceptible to the selected immunotherapy due to the lack of targetable antigens or alterations in immunoregulatory pathways[106]. The primary mutations discussed in melanoma research include BRAF, PTEN, and NRAS mutations.
BRAF mutations
Clinically, the most prevalent mutation is the BRAF mutation, accounting for 50% of all mutations, and BRAFV600E accounts for 90% of BRAF mutations. BRAF mutations induce cell proliferation and migration by activating the MAPK signaling pathway. BRAF inhibitors have been widely used as targeted drugs, such as dabrafenib, vemurafenib, and encorafenib. However, the tumor heterogeneity of melanoma could easily lead to off-target effects of these drugs. Some common drug combinations are used to deal with tumor heterogeneity, such as BRAF/MEK inhibitors. Tian et al. also combined a BRAF/MEK inhibitor with an anti-PD-1 inhibitor in treating colorectal cancer (NCT03668431)[107]. Clinical trials combine various tumor drugs to find a treatment combination with higher efficiency. However, discovering the immune escape mechanism caused by different mutations is the fundamental way to solve resistant problems.
PTEN mutations
PTEN mutations belonging to the PI3K pathway can trigger immune evasion in melanoma. PTEN mutation not only reduces the cytotoxicity of T cells but also indirectly affects the functions of other immune cells, such as reducing the immune activity of B cells. In addition, some melanomas have low microphthalmia-associated transcription factor (MITF) expression. In the melanomas with simultaneous deletion of MITF and PTEN, there is a more obvious reduction of T cell recruitment. In addition, MITFlow/PTENneg also reduces the expression of Major Histocompatibility Complex (MHC)-related genes, thereby causing resistance to immune checkpoint inhibitors[108].
Loss of MHC antigen
MHC antigens play a vital role in the immune system, and they are critical factors in immune cell recognition and clearance of tumor cells. MHC molecules, especially MHC class I and II molecules, play a central role in the antigen presentation process. They can present antigenic fragments inside tumor cells to T cells, thereby activating T cell-mediated immune responses. The absence or decreased expression level of MHC molecules can weaken the recognition and attack of tumor cells by T cells, leading to immune escape. Melanoma cells can reduce the expression of MHC molecules through a variety of mechanisms. For example, they may silence MHC gene expression through epigenetic regulation, such as DNA methylation. In addition, specific cytokines in the tumor microenvironment may also affect the expression and function of MHC molecules. Deletion or downregulation of MHC antigens limits immunotherapy’s effectiveness, as immune checkpoint inhibitors (e.g., PD-1/PD-L1 inhibitors) depend on T cell recognition of tumor antigens. If MHC molecule expression is insufficient, T cells may not be able to recognize tumor cells effectively. They thus cannot be activated or maintained in an activated state, leading to resistance to immunotherapy[16]. The relationship between the loss of MHC-I antigen presentation mechanism and immune evasion could also be demonstrated in studies of melanoma resistance to MAPK inhibitor therapy. The study found that during the drug resistance phase of treatment, the expression of MHC-I molecules on the surface of tumor cells decreased, resulting in a decrease in T cell infiltration, thereby weakening the antitumor immune response. These results suggest that MHC-I molecules play a vital role in the tumor immune microenvironment and that activating signaling pathways such as MAPK, PI3K-mTOR, and Wnt is associated with the downregulation of MHC-I molecular expression. These findings provide a new therapeutic strategy to overcome resistance to MAPK inhibitors and immune checkpoint blockade therapies in melanoma[109].
Other genetic factors
Besides a few well-studied melanoma mutations, many genetic mutations or deletions of different functions are associated with immunotherapy resistance. Previous studies have demonstrated that ipilimumab promotes IFN-γ production and can promote T cell proliferation in a tumor environment, helping immunotherapy. However, Gao et al. analyzed the clinical data of patients who did not respond to ipilimumab and found that gene defects in the IFN-γ pathway lead to melanoma’s response to immune checkpoint inhibitors, especially anti-CTLA-4 Antibody insensitivity[110]. Moreover, as the tumor grows and responds to treatment, it can accumulate new genetic mutations, including those involved in immune evasion. This genetic diversity within the tumor can pose challenges for treatment, as different subclones may respond differently to immunotherapy[111]. The highly adaptive nature of tumors results in some cancer cells with specific genetic mutations or resistance-conferring changes surviving and proliferating when the immune system targets and eliminates vulnerable tumor cells[112]. Over time, these drug-resistant cells can dominate the tumor mass, leading to treatment failure. The ability of tumors to “escape” the immune response is an ongoing challenge in cancer immunotherapy.
MOLECULAR BIOMARKERS OF IMMUNOTHERAPY
Immunotherapy has emerged as a game-changer in cancer treatment, offering the promise of durable responses and improved survival for patients with various malignancies. However, the efficacy of immunotherapy is variable, and not all patients benefit equally from these novel treatments. They identified reliable predictive and prognostic biomarkers to determine which patients are most likely to respond to immunotherapy and tailor treatment strategies accordingly[113,114]. This section will explore the current state of predictive and prognostic biomarkers for immunotherapy and their potential role in guiding personalized cancer treatment [Table 1].
Summary of molecular biomarkers
Biomarkers | Biological context | Correlation with response | Ref. | |
Predictive biomarkers | PD-L1 | A ligand expressed on tumor cells that binds to the PD-1 receptor on T cells | + | [116-120] |
TMB | Number of somatic mutations per coding area of a tumor genome | + | [121-123] | |
MSI | Accumulation of errors in repetitive DNA sequences | + | [124-126] | |
TLS | Immune cell aggregates present in non-lymphoid tissues | + | [127-129] | |
TA-HEC | High endothelial venules where immune cells enter the tumor site | + | [130,131] | |
Immune cell subsets | TREM2 macrophage subsets, γδ T cell subsets, B cell subsets, etc. | - | [132] | |
Prognostic biomarkers | TILs | Immune cells that have migrated into the tumor microenvironment | + | [133-135] |
Immune-related genes | Gene sets obtained through data analysis that could influence immune infiltration scores | + | [136] | |
Gut microbiome | Gut microbiome influences immunotherapy’s efficacy | + | [137-139] | |
FAM | Fatty acid synthesis and its transportation | - | [140-142] | |
HHLA2 | Immune checkpoint of the B7 family | + | [143-145] | |
Circulating biomarkers | Serum LDH | Prognostic/predictive | - | [146-148] |
The NLR | Prognostic | - | [149,150] | |
CRP | Prognostic | - | [151,152] | |
Diversity and clonality of TCRs | Prognostic/predictive | + | [153,154] | |
Circulating EVs | Predictive | - | [155-158] | |
Blood cell composition | Predictive | +/- | [159] |
Predictive biomarkers
Predictive biomarkers are biomolecular or genetic characteristics that help predict a patient’s response to a specific treatment. In the context of immunotherapy, these biomarkers can assist in identifying patients who are more likely to benefit from ICIs or other immunotherapeutic agents. Predictive biomarkers can spare patients from unnecessary treatments and associated toxicities while ensuring that those most likely to respond receive timely and targeted therapies[115].
One of the most extensively studied predictive biomarkers for immunotherapy is PD-L1 expression on tumor cells. PD-L1 is a ligand expressed on tumor cells that binds to the PD-1 receptor on T cells, suppressing their immune response[116]. High levels of PD-L1 expression have been associated with improved responses to anti-PD-1/PD-L1 therapy in multiple cancer types, including melanoma, non-small cell lung cancer, urothelial carcinoma, triple-negative breast cancer, gastric adenocarcinoma, and ovarian cancer[117,118]. Based on determining PD-L1 expression levels, physicians can better decide which patients may benefit from immune checkpoint inhibitor therapy. For example, the approval of certain drugs is based on specific thresholds for PD-L1 expression levels, such as the PD-L1 Tumor Proportion Score (TPS) of ≥ 1% when using pembrolizumab in patients with non-small cell lung cancer. Changes in the expression level of PD-L1 in the tumor treatment stage can also help with precision therapy. For example, in a mouse model of melanoma MAPK-targeted treatment, it was found that the loss of ITCH expression in tumor cells can lead to upregulation of PD-L1 expression, decreased infiltration of CD8+ T cells within tumors, and increased number of regulatory CD4+ T cells and type 2 tumor-associated macrophages, thereby promoting tumor escape immunosuppression and generating treatment resistance, while high expression of ITCH can inhibit the upregulation of PD-L1 during treatment and maintain CD8+ effector T in tumors Proliferation and activity of cells, so activating the E3 ubiquitin ligase activity of ITCH can be used as a combination therapy with MAPK-targeted therapy[119]. However, the clinical utility of PD-L1 as a sole predictive biomarker is limited by variable expression levels across tumor types and heterogeneity within individual tumors[120]. PD-L1 expression may not be sufficient as a potent biomarker in some rare tumor subtypes. For example, some tumors may achieve immune evasion through other pathways, making the association between PD-L1 expression and treatment response not obvious.
Tumor mutational burden (TMB), the number of somatic mutations per coding area of a tumor genome, has emerged as a potential predictor of immunotherapy response[121]. Cancers with high TMB tend to produce more neoantigens, which the immune system recognizes as foreign, increasing the likelihood of an antitumor immune response. Several studies have shown that patients with high TMB exhibit improved responses to immunotherapy, irrespective of the tumor type[122,123].
Microsatellite instability (MSI) is another promising biomarker associated with immunotherapy response. MSI results from deficient DNA mismatch repair, leading to an accumulation of errors in repetitive DNA sequences[124,125]. MSI-high tumors are more responsive to immunotherapy, as these tumors generate a higher load of neoantigens, enhancing the immune system’s ability to recognize and target cancer cells[126].
Tertiary lymphoid structures (TLS) are immune cell aggregates in non-lymphoid tissues, usually in an inflammatory environment, and have been found in various cancers, including melanoma. TLS have CD20+ B cells wrapped by CD4+ T cells[127,128]. The study found that TLS-high tumors are more sensitive to anti-CTLA-4 antibodies, indicating a higher overall survival rate after receiving anti-CTLA-4 antibodies[129].
After cancer patients receive ICI therapy, immune cells enter the tumor site through tumor-associated high endothelial venules (TA-HEVs), and their endothelial cells (TA-HEC) are associated with T-cell infiltration[130]. The biopsy analysis of 93 melanoma patients proved that the higher the frequency and maturity of TA-HEC, the better the survival rate of patients receiving the combination of anti-PD-1 antibody and anti-CTLA-4 antibody[131].
In addition to finding a biomarker positively correlated with ICI efficacy, finding a biomarker that can predict ICI non-response will provide another basis for prediction. Analysis of a single-cell sequencing data set of melanoma samples found that patients who did not respond to ICI therapy had overexpressed TREM2 macrophage subsets and γδ T cell subsets and had fewer B cell subsets[132].
Prognostic biomarkers: beyond response prediction
While predictive biomarkers focus on identifying patients who are likely to respond to immunotherapy, prognostic biomarkers provide information about the patient’s overall disease outcome, regardless of treatment response. These biomarkers can aid in understanding the patient’s prognosis and inform treatment decisions beyond immunotherapy[113,114].
One of the well-established prognostic biomarkers in cancer is TILs. TILs are immune cells that have migrated into the tumor microenvironment and play a crucial role in antitumor immune responses[133]. High levels of TILs have been associated with better prognosis and improved responses to immunotherapy in various cancer types, including melanoma and breast cancer[134,135].
In addition to immune cells as prognostic markers, some studies have also screened a series of immune-related genes to obtain a gene set that can effectively respond to immunotherapy. Huang et al. screened 63 immune genes related to melanoma prognosis, sorted out a gene set with eight immune genes (PSME1, CDC42, CMTM6, HLA-DQB1, HLA-C, CXCR6, CD8B, TNFSF13) as melanoma prognostic markers, and verified their good accuracy as prognostic markers by using TCGA cohort[136].
The gut microbiome has emerged as a potential prognostic biomarker for immunotherapy response[137]. Studies have shown that the gut microbiome’s composition can influence immunotherapy’s efficacy, as certain bacterial species can modulate the systemic immune response[138]. Understanding the role of the gut microbiome in immunotherapy outcomes could open new avenues for optimizing treatment responses. A recent metagenomic sequencing study of stool samples from 106 of 120 patients who participated in a clinical trial of the combination of ipilimumab and nivolumab found that gut microbial abundance could improve patient response to ICI. The study also points to a specific strain of Faecalibacterium associated with a positive reaction to combined ICI therapy in cancer patients[139]. This study highlights the importance of gut microbiome strain level analysis for understanding and predicting the efficacy of ICI therapy in the gut microbiome. It provides new insights for the development of microbiome-based cancer treatment strategies.
Fatty acid metabolism (FAM) is an integral part of the human body’s metabolic pathway and is cells’ main energy-generating metabolic pathway. Dysregulation of fatty acid metabolism can be found in various tumors[140]. A study screened nine FAM-related lncRNAs from the TCGA database as prognostic biomarkers for female cervical cancer[141]. Xu et al. also screened a group of FAM molecular subtypes through the TCGA database, in which six significant genes (ACSL5, ALOX5AP, CD1D, CD74, IL4I1, and TBXAS1) can act as biomarkers for melanoma prognosis[142]. Tumors with higher levels of expression have a higher sensitivity to chemotherapy. At the same time, high gene expression predicts tumors’ immune evasion ability and is highly correlated with PD-1 signaling pathways, T cell antigen presentation, and immune checkpoints. Therefore, the high expression of these genes also indicates a poor response to ICI.
HERV-HLTR-associating 2 (HHLA2) is an immune checkpoint of the B7 family[143]. HHLA2 can be a prognostic biomarker in various cancers, such as ovarian and pan-cancer (all cancers)[144]. Huang et al. examined the relationship between HHLA2 expression and the prognosis of melanoma patients[145]. They found a positive correlation between HHLA2 and CD8+ levels in tissues from 81 patients with advanced unresectable melanoma. Melanoma with high expression of HHLA2 predicts a better prognosis.
Circulating biomarkers
Compared with biomarkers that need to be obtained by complex testing methods such as immunohistochemistry, H&E staining, and next-generation sequencing, circulating biomarkers are a series of non-invasive biomarkers, usually obtained through peripheral blood or body fluid testing. The information acquisition is relatively easy and more conducive to early detection and real-time monitoring of treatment prognosis.
Serum lactate dehydrogenase (LDH) is one of the tumor prognostic biomarkers, and elevated LDH levels predict poor prognosis for tumor immunotherapy. LDH is also considered an effective predictive and prognostic biomarker in cases of melanoma[146,147]. However, the significance of LDH changes under different treatments still needs to be accumulated through research data. In a retrospective study of patients with malignant melanoma in multiple melanoma centers around the world, patients with BRAFV600 mutations with elevated LDH levels who received anti-PD-1 antibody and anti-CTLA-4 antibody had longer PFS and OS than patients treated with first-line targeted therapy or a single ICI. Still, in BRAF wt patients with high levels of LDH, combination therapy is not significantly superior to ICI alone, which also indicates some limitations of LDH as a biomarker for melanoma[148].
The neutrophil-to-lymphocyte ratio (NLR) is an essential parameter in blood counts that reflects the inflammatory status and immune surveillance balance. In a variety of solid tumor patients, an elevated NLR is often associated with a worse prognosis. During melanoma immunotherapy, changes in NLR may indicate treatment response and patient survival, and a decrease in NLR may be associated with treatment response and longer PFS[149]. The rationale behind NLR as a biomarker has also been discovered in the latest retrospective study. Transcriptome analysis showed that genes associated with elevated NLR, such as CD39, CCNA1, LDHA, and IL18R1, played a role in immunosuppression and inflammation, which could be the possible reasons for the poor prognosis of high NLR[150].
C-reactive protein (CRP) is an acute-phase protein usually elevated in response to inflammation or infection. In melanoma immunotherapy, elevated CRP levels may reflect the body’s immune response to the tumor. Some studies have pointed out that CRP levels are related to the efficacy of immunotherapy and patient survival and that patients with higher CRP levels may have poor treatment outcomes[151,152].
The diversity and clonality of T cell receptors (TCRs) are important indicators for assessing T cell immune responses. In melanoma immunotherapy, TCR diversity and clonality analysis can help to understand tumor-specific T cell activity and the efficacy of immunotherapy. Studies have shown that increased TCR diversity after treatment may be associated with better treatment response and survival[153]. In addition, further studies have shown that TCR repertoire metrics in tumor-infiltrating T lymphocytes (TIL/Tc) diversity and clonality have prognostic and predictive effects on OS and anti-PD-1 antibodies, respectively. High TIL/Tc diversity enables the identification of patients who can control tumors without PD1 inhibitors. Thus, further research on this approach will provide crucial information on the choice of treatment options for melanoma[154].
Circulating extracellular vesicles (EVs) are vesicles composed of lipid bilayers, which can contain a variety of biomolecules, such as proteins, lipids, receptors, etc. Apoptotic bodies are often considered larger vesicles originating from the cell membrane’s budding and containing cellular debris. At the same time, exosomes are smaller EVs formed within the endosomes and released after fusion into the cell membrane[155]. EVs are believed to affect the tumor microenvironment and the immune system’s response against tumors[156]. The surface of the EV membrane expresses PD-L1 and CTLA-4. Therefore, EVs will likely be used as predictive biomarkers[157]. Serratì et al. demonstrated that patients who do not respond to ICI therapy may have higher levels of PD-L1+EV and PD-1+EV (from tumor cells and immune cells, respectively), so they can serve as two predictive biomarkers for melanoma immunotherapy[158].
Additionally, blood cell composition in the circulating blood is also an essential non-invasive biomarker. Changes in the levels of CD8+ T Cells, CD4+ T Cells, Nature Killer cells, B cells, Myeloid cells, and circulating tumor cells in peripheral blood and the ratio between them have different degrees of predictive effect. Splendiani et al. provide a good summary of the ability of these cells to act as biomarkers[159]. The research and application of non-invasive circulating biomarkers bring significant advantages to cancer management, as they minimize risk and discomfort for patients by detecting blood or other bodily fluid samples. These biomarkers help enable early detection and diagnosis of cancer, facilitating patients to start treatment promptly, thereby improving survival. In addition, they can monitor the effect of treatment in real time, providing doctors with a basis for adjusting treatment plans and accurately assessing the patient’s prognosis. By identifying high-risk populations and guiding individualized treatment, circulating biomarkers improve the precision and effectiveness of treatment, help reduce healthcare costs, and improve patients’ quality of life. Its convenience and accessibility have further promoted the progress of dynamic cancer monitoring and research, opening up new possibilities for discovering new therapeutic targets and promoting the development of cancer treatment technologies.
While significant progress has been made in identifying predictive and prognostic biomarkers for immunotherapy, several challenges remain. The heterogeneity of tumors and their microenvironments and the dynamic nature of the immune response make biomarker discovery and validation complex[160,161]. In addition, the boundary between predictive and prognostic biomarkers is usually unclear, so when people discover a new biomarker, it is impossible to categorize it definitively as predictive or prognosis[162]. To address these challenges, multi-omic approaches, such as integrating genomics, transcriptomics, and proteomics data, are employed to identify comprehensive biomarker signatures[163,164]. Machine learning algorithms and artificial intelligence are also used to analyze large datasets and uncover novel associations between biomarkers and treatment responses[165,166]. Moreover, combining predictive and prognostic biomarkers may offer a more comprehensive assessment of patient outcomes and treatment responses[167]. Clinicians can tailor treatment strategies and optimize patient outcomes by integrating multiple biomarkers.
PERSPECTIVES AND CONCLUSIONS
Immunotherapy has revolutionized the treatment landscape for melanoma, providing remarkable clinical benefits for many patients. Identifying predictive and prognostic biomarkers has further improved patient selection and treatment outcomes. However, challenges exist, such as resistance development and identifying the most effective treatment combinations. In this review, we conducted a multifaceted summary of studies related to melanoma immunotherapy in the past three years by searching the PubMed database. First, this review summarizes the six main immunotherapy strategies for melanoma at this stage and three major resistance mechanisms. The therapeutic effect of each immunotherapy varies, and to minimize the drug’s side effects and reduce the recurrence rate, it is clinically preferred to provide different combination therapy regimens according to each patient’s specific situation. Finding the proper treatment for each patient and giving precision treatment will become the mainstream of future research. Therefore, diverse and accurate biomarkers are essential. This review also summarizes the novel biomarkers published in the database in the past three years. These biomarkers are extensions of some common biomarkers and provide new ideas for subsequent biomarker research. The development of biomarkers will take immunotherapy for cancer to a new level. A variety of research methods are mentioned in this review, including basic research, prospective research, retrospective research, and clinical trials. Among them, it is inevitable that some retrospective studies may have problems with incomplete or incomplete records, and there may be potential bias in data collection, resulting in an inability to accurately reflect the actual situation, which leads to the questioning of the reliability of the study results. Recognizing these inherent limitations, this paper endeavors to mitigate them by carefully excluding low-quality studies during the article selection process. Furthermore, in certain clinical trials, the presence of samples that are either too small or too large, as well as the exclusion of prematurely terminated samples from the entire process, can undeniably introduce bias to the experimental results.
As melanoma immunotherapy evolves, several promising avenues deserve exploration to enhance treatment efficacy and patient outcomes. While immunotherapy has shown remarkable results, some patients still experience treatment resistance. Understanding the mechanisms underlying resistance to immunotherapy and developing strategies to overcome it will be vital in improving long-term responses and reducing relapse rates. In addition, immunotherapy is continuously advancing, and the discovery of novel immunotherapeutic approaches such as adoptive T-cell therapy, cancer vaccines, and oncolytic viruses should be further explored for their potential in melanoma treatment. Much of the melanoma immunotherapy research has focused on advanced or metastatic disease. Investigating the role of immunotherapy in earlier stages of melanoma may offer opportunities for better long-term disease control and potential cures. Continued monitoring of patients who have received immunotherapy is essential to gain insights into the durability of responses and possible long-term side effects. Understanding the long-term safety and efficacy profiles will aid in optimizing treatment strategies and managing patient expectations.
The future of immunotherapy for melanoma holds excellent promise. As research advances, we can expect more refined treatment strategies considering individual patient characteristics and tumor biology. Personalized medicine based on predictive biomarkers will likely become a standard approach, leading to increased response rates and improved survival. Moreover, novel therapeutic targets and combination therapies will emerge as we uncover the intricacies of the tumor microenvironment and the immune response. Collaborative efforts between clinicians, researchers, and industry stakeholders will be crucial in translating these discoveries into clinical applications. Ultimately, ongoing research and clinical trials will pave the way for continued progress in immunotherapy for melanoma, bringing us closer to improving patient outcomes and achieving long-lasting remissions of this devastating disease.
DECLARATIONS
Acknowledgments
We appreciate and acknowledge the support from the Laboratory and Research Center and the Office of Research and Sponsored Programs at Wenzhou-Kean University.
Authors’ contributions
Conceived the contents and structure: Tian X, Yang Y
Wrote the original manuscript: Song L
Revised and improved the manuscript: Tian X, Yang Y
All authors have read and agreed to the published version of the manuscript.
Availability of data and materials
Not applicable.
Financial support and sponsorship
This research was funded by Wenzhou Municipal Key Laboratory for Applied Biomedical and the Biopharmaceutical Informatics [WB20211227000125] and Zhejiang Bioinformatics International Science and Technology Cooperation Center at Wenzhou-Kean University [WB20210429000008].
Conflicts of interest
All authors declared that there are no conflicts of interest.
Ethical approval and consent to participate
Not applicable.
Consent for publication
Not applicable.
Copyright
© The Author(s) 2024.
REFERENCES
1. Turner N, Ware O, Bosenberg M. Genetics of metastasis: melanoma and other cancers. Clin Exp Metastasis 2018;35:379-91.
4. Koczkodaj P, Sulkowska U, Didkowska J, Rutkowski P, Mańczuk M. Melanoma mortality trends in 28 European countries: a retrospective analysis for the years 1960-2020. Cancers 2023;15:1514.
5. Lopes FCPS, Sleiman MG, Sebastian K, Bogucka R, Jacobs EA, Adamson AS. UV exposure and the risk of cutaneous melanoma in skin of color: a systematic review. JAMA Dermatol 2021;157:213-9.
7. Pham JP, Joshua AM, da Silva IP, Dummer R, Goldinger SM. Chemotherapy in cutaneous melanoma: is there still a role? Curr Oncol Rep 2023;25:609-21.
8. Carlino MS, Larkin J, Long GV. Immune checkpoint inhibitors in melanoma. Lancet 2021;398:1002-14.
9. Mandala M, Larkin J, Ascierto PA, et al. Correction: Adjuvant nivolumab for stage III/IV melanoma: evaluation of safety outcomes and association with recurrence-free survival. J Immunother Cancer 2021;9:e003188corr1.
10. Dillman RO, Barth NM, Vandermolen LA, Mahdavi K, Mcclure SE. Should high-dose interleukin-2 still be the preferred treatment for patients with metastatic melanoma? Cancer Biother Radio 2012;27:337-43.
11. Postow MA, Chesney J, Pavlick AC, et al. Nivolumab and ipilimumab versus ipilimumab in untreated melanoma. N Engl J Med 2015;372:2006-17.
12. Somasundaram R, Villanueva J, Herlyn M. Chapter eleven - Intratumoral heterogeneity as a therapy resistance mechanism: role of melanoma subpopulations. Adv Pharmacol 2012;65:335-59.
14. Wang DR, Wu XL, Sun YL. Therapeutic targets and biomarkers of tumor immunotherapy: response versus non-response. Signal Transduct Target Ther 2022;7:331.
15. Zhang Y, Zhang Z. The history and advances in cancer immunotherapy: understanding the characteristics of tumor-infiltrating immune cells and their therapeutic implications. Cell Mol Immunol 2020;17:807-21.
16. Huang AC, Zappasodi R. A decade of checkpoint blockade immunotherapy in melanoma: understanding the molecular basis for immune sensitivity and resistance. Nat Immunol 2022;23:660-70.
17. Leach DR, Krummel MF, Allison JP. Enhancement of antitumor immunity by CTLA-4 blockade. Science 1996;271:1734-6.
18. Brahmer JR, Drake CG, Wollner I, et al. Phase I study of single-agent anti-programmed death-1 (MDX-1106) in refractory solid tumors: safety, clinical activity, pharmacodynamics, and immunologic correlates. J Clin Oncol 2010;28:3167-75.
19. Buchbinder EI, Desai A. CTLA-4 and PD-1 pathways: similarities, differences, and implications of their inhibition. Am J Clin Oncol 2016;39:98-106.
20. Patsoukis N, Wang Q, Strauss L, Boussiotis VA. Revisiting the PD-1 pathway. Sci Adv 2020;6:eabd2712.
21. Wu Q, Jiang L, Li SC, He QJ, Yang B, Cao J. Small molecule inhibitors targeting the PD-1/PD-L1 signaling pathway. Acta Pharmacol Sin 2021;42:1-9.
22. Ivashko IN, Kolesar JM. Pembrolizumab and nivolumab: PD-1 inhibitors for advanced melanoma. Am J Health Syst Pharm 2016;73:193-201.
23. Patel SP, Othus M, Chen Y, et al. Neoadjuvant-adjuvant or adjuvant-only pembrolizumab in advanced melanoma. N Engl J Med 2023;388:813-23.
24. Amaria RN, Postow M, Burton EM, et al. Neoadjuvant relatlimab and nivolumab in resectable melanoma. Nature 2022;611:155-60.
25. Mallardo D, Simeone E, Vanella V, et al. Concomitant medication of cetirizine in advanced melanoma could enhance anti-PD-1 efficacy by promoting M1 macrophages polarization. J Transl Med 2022;20:436.
26. Brito ABC, Camandaroba MPG, de Lima VCC. Anti-PD1 versus anti-PD-L1 immunotherapy in first-line therapy for advanced non-small cell lung cancer: a systematic review and meta-analysis. Thorac Cancer 2021;12:1058-66.
27. Snyder A, Makarov V, Merghoub T, et al. Genetic basis for clinical response to CTLA-4 blockade in melanoma. N Engl J Med 2014;371:2189-99.
28. Sarnaik AA, Weber JS. Recent advances using anti-CTLA-4 for the treatment of melanoma. Cancer J 2009;15:169-73.
29. Sondak VK, Smalley KS, Kudchadkar R, Grippon S, Kirkpatrick P. Ipilimumab. Nat Rev Drug Discov 2011;10:411-2.
30. Maio M, Grob J, Aamdal S, et al. Five-year survival rates for treatment-naive patients with advanced melanoma who received ipilimumab plus dacarbazine in a phase III trial. J Clin Oncol 2015;33:1191-6.
31. Eggermont AM, Chiarion-Sileni V, Grob JJ, et al. Prolonged survival in stage III melanoma with ipilimumab adjuvant therapy. N Engl J Med 2016;375:1845-55.
32. Ascierto PA, Del Vecchio M, Mackiewicz A, et al. Correction: Overall survival at 5 years of follow-up in a phase III trial comparing ipilimumab 10 mg/kg with 3 mg/kg in patients with advanced melanoma. J Immunother Cancer 2020;8:e000391corr1.
33. Seth R, Agarwala SS, Messersmith H, et al. Systemic therapy for melanoma: ASCO guideline update. J Clin Oncol 2023;41:4794-820.
35. Bastholt L, Svane IM, Bjerregaard JK, Herrstedt J, Hróbjartsson A, Schmidt H. High-dose interleukin-2 and interferon as first-line immunotherapy for metastatic melanoma: long-term follow-up in a large unselected Danish patient cohort. Eur J Cancer 2019;115:61-7.
36. Davar D, Ding F, Saul M, et al. High-dose interleukin-2 (HD IL-2) for advanced melanoma: a single center experience from the University of Pittsburgh Cancer Institute. J Immunother Cancer 2017;5:74.
37. Floros T, Tarhini AA. Anticancer cytokines: biology and clinical effects of interferon-α2, interleukin (IL)-2, IL-15, IL-21, and IL-12. Semin Oncol 2015;42:539-48.
39. Shen J, Xiao Z, Zhao Q, et al. Anti-cancer therapy with TNFα and IFNγ: a comprehensive review. Cell Prolif 2018;51:e12441.
40. Hansen ML, Woetmann A, Krejsgaard T, et al. IFN-α primes T- and NK-cells for IL-15-mediated signaling and cytotoxicity. Mol Immunol 2011;48:2087-93.
41. Kirkwood JM, Strawderman MH, Ernstoff MS, Smith TJ, Borden EC, Blum RH. Interferon alfa-2b adjuvant therapy of high-risk resected cutaneous melanoma: the eastern cooperative oncology group trial EST 1684. J Clin Oncol 2023;41:425-35.
42. Shi Q, Liu L, Zhang W, et al. Interferon-α1b for the treatment of metastatic melanoma: results of a retrospective study. Anticancer Drug 2021;32:1105-10.
43. Zhu G, Shi Q, Zhao B, et al. Efficacy and safety of interferon-alpha 1b combined with PD-1 monoclonal antibody in patients with unresectable stage IV melanoma: a retrospective study. J Cancer Res Clin Oncol 2023;149:6263-9.
44. Davar D, Wang H, Chauvin J, et al. Phase Ib/II study of pembrolizumab and pegylated-interferon alfa-2b in advanced melanoma. J Clin Oncol 2018;36:3450-8.
45. Chen L, Chen F, Li J, et al. CAR-T cell therapy for lung cancer: potential and perspective. Thorac Cancer 2022;13:889-99.
46. Xue G, Zheng N, Fang J, et al. Adoptive cell therapy with tumor-specific Th9 cells induces viral mimicry to eliminate antigen-loss-variant tumor cells. Cancer Cell 2021;39:1610-22.e9.
47. Wang S, Sun J, Chen K, et al. Perspectives of tumor-infiltrating lymphocyte treatment in solid tumors. BMC Med 2021;19:140.
48. Kumar A, Watkins R, Vilgelm AE. Cell therapy with TILs: training and taming T cells to fight cancer. Front Immunol 2021;12:690499.
49. Dafni U, Michielin O, Lluesma SM, et al. Efficacy of adoptive therapy with tumor-infiltrating lymphocytes and recombinant interleukin-2 in advanced cutaneous melanoma: a systematic review and meta-analysis. Ann Oncol 2019;30:1902-13.
50. Leko V, Rosenberg SA. Identifying and targeting human tumor antigens for T cell-based immunotherapy of solid tumors. Cancer Cell 2020;38:454-72.
51. Chesney J, Lewis KD, Kluger H, et al. Efficacy and safety of lifileucel, a one-time autologous tumor-infiltrating lymphocyte (TIL) cell therapy, in patients with advanced melanoma after progression on immune checkpoint inhibitors and targeted therapies: pooled analysis of consecutive cohorts of the C-144-01 study. J Immunother Cancer 2022;10:e005755.
52. Hirai I, Funakoshi T, Kamijuku H, et al. Adoptive cell therapy using tumor-infiltrating lymphocytes for melanoma refractory to immune-checkpoint inhibitors. Cancer Sci 2021;112:3163-72.
53. Sarnaik AA, Hamid O, Khushalani NI, et al. Lifileucel, a tumor-infiltrating lymphocyte therapy, in metastatic melanoma. J Clin Oncol 2021;39:2656-66.
54. Schoenfeld AJ, Lee SM, Doger de Speville B, et al. Lifileucel, an autologous tumor-infiltrating lymphocyte monotherapy, in patients with advanced non-small cell lung cancer resistant to immune checkpoint inhibitors. Cancer Discovery 2024.
55. Rosenberg SA, Spiess P, Lafreniere R. A new approach to the adoptive immunotherapy of cancer with tumor-infiltrating lymphocytes. Science 1986;233:1318-21.
56. Rezaei T, Davoudian E, Khalili S, et al. Strategies in DNA vaccine for melanoma cancer. Pigment Cell Melanoma Res 2021;34:869-91.
57. Sahin U, Oehm P, Derhovanessian E, et al. An RNA vaccine drives immunity in checkpoint-inhibitor-treated melanoma. Nature 2020;585:107-12.
58. Liu S, Jiang Q, Zhao X, et al. A DNA nanodevice-based vaccine for cancer immunotherapy. Nat Mater 2021;20:421-30.
59. Yazdani Z, Rafiei A, Irannejad H, Yazdani M, Valadan R. Designing a novel multiepitope peptide vaccine against melanoma using immunoinformatics approach. J Biomol Struct Dyn 2022;40:3312-24.
60. Kjeldsen JW, Lorentzen CL, Martinenaite E, et al. A phase 1/2 trial of an immune-modulatory vaccine against IDO/PD-L1 in combination with nivolumab in metastatic melanoma. Nat Med 2021;27:2212-23.
61. Hu Z, Leet DE, Allesøe RL, et al. Personal neoantigen vaccines induce persistent memory T cell responses and epitope spreading in patients with melanoma. Nat Med 2021;27:515-25.
62. Ott PA, Hu Z, Keskin DB, et al. An immunogenic personal neoantigen vaccine for patients with melanoma. Nature 2017;547:217-21.
63. Ott PA, Hu-Lieskovan S, Chmielowski B, et al. A phase Ib trial of personalized neoantigen therapy plus anti-PD-1 in patients with advanced melanoma, non-small cell lung cancer, or bladder cancer. Cell 2020;183:347-62.e24.
64. Yin Q, Zhao N, Chang Y, et al. Melanoma stem cell vaccine induces effective tumor immunity against melanoma. Hum Vaccin Immunother 2023;19:2158670.
65. Khattak A, Weber JS, Meniawy T, et al. Distant metastasis-free survival results from the randomized, phase 2 mRNA-4157-P201/KEYNOTE-942 trial. J Clin Oncol 2023;41:LBA9503.
66. Topp MS, Gökbuget N, Stein AS, et al. Correction to Lancet Oncol 2015; 16: 60, 61. Safety and activity of blinatumomab for adult patients with relapsed or refractory B-precursor acute lymphoblastic leukaemia: a multicentre, single-arm, phase 2 study. Lancet Oncol 2015;16:e158.
67. Park K, Haura EB, Leighl NB, et al. Amivantamab in EGFR exon 20 insertion-mutated non-small-cell lung cancer progressing on platinum chemotherapy: initial results from the CHRYSALIS phase I study. J Clin Oncol 2021;39:3391-402.
68. Nathan P, Hassel JC, Rutkowski P, et al; IMCgp100-202 Investigators. Overall survival benefit with tebentafusp in metastatic uveal melanoma. N Engl J Med 2021;385:1196-206.
69. Hassel JC, Piperno-Neumann S, Rutkowski P, et al. Three-year overall survival with tebentafusp in metastatic uveal melanoma. N Engl J Med 2023;389:2256-66.
70. Tawbi HA, Schadendorf D, Lipson EJ, et al; RELATIVITY-047 Investigators. Relatlimab and nivolumab versus nivolumab in untreated advanced melanoma. N Engl J Med 2022;386:24-34.
71. Atkins MB, Lee SJ, Chmielowski B, et al. Combination dabrafenib and trametinib versus combination nivolumab and ipilimumab for patients with advanced BRAF-mutant melanoma: the DREAMseq trial-ECOG-ACRIN EA6134. J Clin Oncol 2023;41:186-97.
72. Larkin J, Chiarion-Sileni V, Gonzalez R, et al. Five-year survival with combined nivolumab and ipilimumab in advanced melanoma. N Engl J Med 2019;381:1535-46.
73. Versluis JM, Reijers ILM, Rozeman EA, et al. Neoadjuvant ipilimumab plus nivolumab in synchronous clinical stage III melanoma. Eur J Cancer 2021;148:51-7.
74. Weber JS, Schadendorf D, Del Vecchio M, et al. Adjuvant therapy of nivolumab combined with ipilimumab versus nivolumab alone in patients with resected stage IIIB-D or stage IV melanoma (CheckMate 915). J Clin Oncol 2023;41:517-27.
75. Campbell KM, Amouzgar M, Pfeiffer SM, et al. Prior anti-CTLA-4 therapy impacts molecular characteristics associated with anti-PD-1 response in advanced melanoma. Cancer Cell 2023;41:791-806.e4.
76. Tawbi HA, Forsyth PA, Algazi A, et al. Combined nivolumab and ipilimumab in melanoma metastatic to the brain. N Engl J Med 2018;379:722-30.
78. Zhao H, Teng D, Yang L, et al. Myeloid-derived itaconate suppresses cytotoxic CD8+ T cells and promotes tumour growth. Nat Metab 2022;4:1660-73.
79. Singh L, Muise ES, Bhattacharya A, et al. ILT3 (LILRB4) promotes the immunosuppressive function of tumor-educated human monocytic myeloid-derived suppressor cells. Mol Cancer Res 2021;19:702-16.
80. Jenkins L, Jungwirth U, Avgustinova A, et al. Cancer-associated fibroblasts suppress CD8+ T-cell infiltration and confer resistance to immune-checkpoint blockade. Cancer Res 2022;82:2904-17.
81. Yao W, German B, Chraa D, et al. Keratinocyte-derived cytokine TSLP promotes growth and metastasis of melanoma by regulating the tumor-associated immune microenvironment. JCI Insight 2022;7:e161438.
82. Yang M, Lin C, Wang Y, Chen K, Zhang H, Li W. Identification of a cytokine-dominated immunosuppressive class in squamous cell lung carcinoma with implications for immunotherapy resistance. Genome Med 2022;14:72.
83. Tabolacci C, Cordella M, Mariotti S, et al. Melanoma cell resistance to vemurafenib modifies inter-cellular communication signals. Biomedicines 2021;9:79.
84. Pandya P, Kublo L, Stewart-ornstein J. p53 promotes cytokine expression in melanoma to regulate drug resistance and migration. Cells 2022;11:405.
85. Lainé A, Labiad O, Hernandez-vargas H, et al. Regulatory T cells promote cancer immune-escape through integrin αvβ8-mediated TGF-β activation. Nat Commun 2021;12:6228.
86. Arkhypov I, Özbay Kurt FG, Bitsch R, et al. HSP90α induces immunosuppressive myeloid cells in melanoma via TLR4 signaling. J Immunother Cancer 2022;10:e005551.
87. Chen L, Diao L, Yang Y, et al. CD38-mediated immunosuppression as a mechanism of tumor cell escape from PD-1/PD-L1 blockade. Cancer Discov 2018;8:1156-75.
88. Vignard V, Labbé M, Marec N, et al. MicroRNAs in tumor exosomes drive immune escape in melanoma. Cancer Immunol Res 2020;8:255-67.
89. Madden MZ, Rathmell JC. The complex integration of T-cell metabolism and immunotherapy. Cancer Discov 2021;11:1636-43.
90. Elia I, Rowe JH, Johnson S, et al. Tumor cells dictate anti-tumor immune responses by altering pyruvate utilization and succinate signaling in CD8+ T cells. Cell Metab 2022;34:1137-50.e6.
91. Chen G, Huang AC, Zhang W, et al. Exosomal PD-L1 contributes to immunosuppression and is associated with anti-PD-1 response. Nature 2018;560:382-6.
92. Huang L, Xu Y, Fang J, et al. Targeting STAT3 abrogates Tim-3 upregulation of adaptive resistance to PD-1 blockade on regulatory T cells of melanoma. Front Immunol 2021;12:654749.
93. Kreidieh FY, Tawbi HA. The introduction of LAG-3 checkpoint blockade in melanoma: immunotherapy landscape beyond PD-1 and CTLA-4 inhibition. Ther Adv Med Oncol 2023;15:17588359231186027.
94. Adashek JJ, Kato S, Nishizaki D, et al. LAG-3 transcriptomic expression patterns across malignancies: implications for precision immunotherapeutics. Cancer Med 2023;12:13155-66.
95. Solinas C, De Silva P, Bron D, Willard-gallo K, Sangiolo D. Significance of TIM3 expression in cancer: from biology to the clinic. Semin Oncol 2019;46:372-9.
96. Frankiw L, Singh A, Peters C, et al. Immunotherapy resistance driven by loss of NY-ESO-1 expression in response to transgenic adoptive cellular therapy with PD-1 blockade. J Immunother Cancer 2023;11:e006930.
97. Shalabi H, Kraft IL, Wang HW, et al. Sequential loss of tumor surface antigens following chimeric antigen receptor T-cell therapies in diffuse large B-cell lymphoma. Haematologica 2018;103:e215-8.
98. Baba T, Shiota H, Kuroda K, et al. Clinical significance of human leukocyte antigen loss and melanoma-associated antigen 4 expression in smokers of non-small cell lung cancer patients. Int J Clin Oncol 2013;18:997-1004.
99. Dhatchinamoorthy K, Colbert JD, Rock KL. Cancer immune evasion through loss of MHC class I antigen presentation. Front Immunol 2021;12:636568.
100. Wculek SK, Cueto FJ, Mujal AM, Melero I, Krummel MF, Sancho D. Dendritic cells in cancer immunology and immunotherapy. Nat Rev Immunol 2020;20:7-24.
101. Hilligan KL, Ronchese F. Antigen presentation by dendritic cells and their instruction of CD4+ T helper cell responses. Cell Mol Immunol 2020;17:587-99.
102. Hargadon KM, Bishop JD, Brandt JP, Hand ZC, Ararso YT, Forrest OA. Melanoma-derived factors alter the maturation and activation of differentiated tissue-resident dendritic cells. Immunol Cell Biol 2016;94:24-38.
103. Dolina JS, Van Braeckel-Budimir N, Thomas GD, Salek-Ardakani S. CD8+ T cell exhaustion in cancer. Front Immunol 2021;12:715234.
104. Peng DH, Rodriguez BL, Diao L, et al. Collagen promotes anti-PD-1/PD-L1 resistance in cancer through LAIR1-dependent CD8+ T cell exhaustion. Nat Commun 2020;11:4520.
105. Plaschka M, Benboubker V, Grimont M, et al. ZEB1 transcription factor promotes immune escape in melanoma. J Immunother Cancer 2022;10:e003484.
106. Galbo PM, Zang X, Zheng D. Molecular features of cancer-associated fibroblast subtypes and their implication on cancer pathogenesis, prognosis, and immunotherapy resistance. Clin Cancer Res 2021;27:2636-47.
107. Tian J, Chen JH, Chao SX, et al. Combined PD-1, BRAF and MEK inhibition in BRAFV600E colorectal cancer: a phase 2 trial. Nat Med 2023;29:458-66.
108. Cabrita R, Mitra S, Sanna A, et al. The role of PTEN loss in immune escape, melanoma prognosis and therapy response. Cancers 2020;12:742.
109. Yu J, Wu X, Song J, et al. Loss of MHC-I antigen presentation correlated with immune checkpoint blockade tolerance in MAPK inhibitor-resistant melanoma. Front Pharmacol 2022;13:928226.
110. Gao J, Shi LZ, Zhao H, et al. Loss of IFN-γ pathway genes in tumor cells as a mechanism of resistance to anti-CTLA-4 therapy. Cell 2016;167:397-404.e9.
111. Yen I, Shanahan F, Lee J, et al. ARAF mutations confer resistance to the RAF inhibitor belvarafenib in melanoma. Nature 2021;594:418-23.
112. Kim TK, Vandsemb EN, Herbst RS, Chen L. Adaptive immune resistance at the tumour site: mechanisms and therapeutic opportunities. Nat Rev Drug Discov 2022;21:529-40.
113. Tímár J, Ladányi A. Molecular pathology of skin melanoma: epidemiology, differential diagnostics, prognosis and therapy prediction. Int J Mol Sci 2022;23:5384.
114. Teixido C, Castillo P, Martinez-Vila C, Arance A, Alos L. Molecular markers and targets in melanoma. Cells 2021;10:2320.
115. Nebhan CA, Johnson DB. Predictive biomarkers of response to immune checkpoint inhibitors in melanoma. Expert Rev Anticancer Ther 2020;20:137-45.
116. Paver EC, Cooper WA, Colebatch AJ, et al. Programmed death ligand-1 (PD-L1) as a predictive marker for immunotherapy in solid tumours: a guide to immunohistochemistry implementation and interpretation. Pathology 2021;53:141-56.
117. Herbst RS, Soria JC, Kowanetz M, et al. Predictive correlates of response to the anti-PD-L1 antibody MPDL3280A in cancer patients. Nature 2014;515:563-7.
118. Powles T, Eder JP, Fine GD, et al. MPDL3280A (anti-PD-L1) treatment leads to clinical activity in metastatic bladder cancer. Nature 2014;515:558-62.
119. Yang Z, Wang Y, Liu S, et al. Enhancing PD-L1 degradation by ITCH during MAPK inhibitor therapy suppresses acquired resistance. Cancer Discov 2022;12:1942-59.
120. Doroshow DB, Bhalla S, Beasley MB, et al. PD-L1 as a biomarker of response to immune-checkpoint inhibitors. Nat Rev Clin Oncol 2021;18:345-62.
121. Lawlor RT, Mattiolo P, Mafficini A, et al. Tumor mutational burden as a potential biomarker for immunotherapy in pancreatic cancer: systematic review and still-open questions. Cancers 2021;13:3119.
122. Hellmann MD, Paz-Ares L, Bernabe Caro R, et al. Nivolumab plus ipilimumab in advanced non-small-cell lung cancer. N Engl J Med 2019;381:2020-31.
123. Chae YK, Davis AA, Raparia K, et al. Association of tumor mutational burden with DNA repair mutations and response to anti-PD-1/PD-L1 therapy in non-small-cell lung cancer. Clin Lung Cancer 2019;20:88-96.e6.
124. Dedeurwaerdere F, Claes KB, Van Dorpe J, et al. Comparison of microsatellite instability detection by immunohistochemistry and molecular techniques in colorectal and endometrial cancer. Sci Rep 2021;11:12880.
125. Shimozaki K, Hayashi H, Tanishima S, et al. Concordance analysis of microsatellite instability status between polymerase chain reaction based testing and next generation sequencing for solid tumors. Sci Rep 2021;11:20003.
126. Le DT, Durham JN, Smith KN, et al. Mismatch repair deficiency predicts response of solid tumors to PD-1 blockade. Science 2017;357:409-13.
128. Fridman WH, Meylan M, Petitprez F, Sun CM, Italiano A, Sautès-Fridman C. B cells and tertiary lymphoid structures as determinants of tumour immune contexture and clinical outcome. Nat Rev Clin Oncol 2022;19:441-57.
129. Cabrita R, Lauss M, Sanna A, et al. Tertiary lymphoid structures improve immunotherapy and survival in melanoma. Nature 2020;577:561-5.
130. Blanchard L, Girard J. High endothelial venules (HEVs) in immunity, inflammation and cancer. Angiogenesis 2021;24:719-53.
131. Asrir A, Tardiveau C, Coudert J, et al. Tumor-associated high endothelial venules mediate lymphocyte entry into tumors and predict response to PD-1 plus CTLA-4 combination immunotherapy. Cancer Cell 2022;40:318-34.e9.
132. Xiong D, Wang Y, You M. A gene expression signature of TREM2hi macrophages and γδ T cells predicts immunotherapy response. Nat Commun 2020;11:5084.
133. Liu Y, Liu Z, Yang Y, Cui J, Sun J, Liu Y. The prognostic and biology of tumour-infiltrating lymphocytes in the immunotherapy of cancer. Br J Cancer 2023;129:1041-9.
134. Denkert C, von Minckwitz G, Darb-Esfahani S, et al. Tumour-infiltrating lymphocytes and prognosis in different subtypes of breast cancer: a pooled analysis of 3771 patients treated with neoadjuvant therapy. Lancet Oncol 2018;19:40-50.
135. Loi S, Drubay D, Adams S, et al. Tumor-infiltrating lymphocytes and prognosis: a pooled individual patient analysis of early-stage triple-negative breast cancers. J Clin Oncol 2019;37:559-69.
136. Huang R, Mao M, Lu Y, Yu Q, Liao L. A novel immune-related genes prognosis biomarker for melanoma: associated with tumor microenvironment. Aging 2020;12:6966-80.
137. Chilakapati SR, Ricciuti J, Zsiros E. Microbiome and cancer immunotherapy. Curr Opin Biotechnol 2020;65:114-7.
138. Gopalakrishnan V, Spencer CN, Nezi L, et al. Gut microbiome modulates response to anti-PD-1 immunotherapy in melanoma patients. Science 2018;359:97-103.
139. Gunjur A, Shao Y, Rozday T, et al. A gut microbial signature for combination immune checkpoint blockade across cancer types. Nat Med 2024;30:797-809.
140. Hoy AJ, Nagarajan SR, Butler LM. Tumour fatty acid metabolism in the context of therapy resistance and obesity. Nat Rev Cancer 2021;21:753-66.
141. Lang X, Huang C, Cui H. Prognosis analysis and validation of fatty acid metabolism-related lncRNAs and tumor immune microenvironment in cervical cancer. J Immunol Res 2022;2022:4954457.
142. Xu Y, Chen Y, Jiang W, et al. Identification of fatty acid metabolism-related molecular subtype biomarkers and their correlation with immune checkpoints in cutaneous melanoma. Front Immunol 2022;13:967277.
143. Zhao R, Chinai JM, Buhl S, et al. HHLA2 is a member of the B7 family and inhibits human CD4 and CD8 T-cell function. Proc Natl Acad Sci U S A 2013;110:9879-84.
144. Xu G, Shi Y, Ling X, et al. HHLA2 predicts better survival and exhibits inhibited proliferation in epithelial ovarian cancer. Cancer Cell Int 2021;21:252.
145. Huang F, Wu J, Cheng X, et al. HHLA2 predicts improved prognosis of anti-PD-1/PD-L1 immunotherapy in patients with melanoma. Front Immunol 2022;13:902167.
146. Gassenmaier M, Lenders MM, Forschner A, et al. Serum S100B and LDH at baseline and during therapy predict the outcome of metastatic melanoma patients treated with BRAF inhibitors. Target Oncol 2021;16:197-205.
147. Long GV, Grob JJ, Nathan P, et al. Factors predictive of response, disease progression, and overall survival after dabrafenib and trametinib combination treatment: a pooled analysis of individual patient data from randomised trials. Lancet Oncol 2016;17:1743-54.
148. Knispel S, Gassenmaier M, Menzies AM, et al. Outcome of melanoma patients with elevated LDH treated with first-line targeted therapy or PD-1-based immune checkpoint inhibition. Eur J Cancer 2021;148:61-75.
149. Capone M, Giannarelli D, Mallardo D, et al. Baseline neutrophil-to-lymphocyte ratio (NLR) and derived NLR could predict overall survival in patients with advanced melanoma treated with nivolumab. J Immunother Cancer 2018;6:74.
150. Mallardo D, Fordellone M, White A, et al. CD39 and LDHA affects the prognostic role of NLR in metastatic melanoma patients treated with immunotherapy. J Transl Med 2023;21:610.
151. Lauwyck J, Beckwée A, Santens A, et al. C-reactive protein as a biomarker for immune-related adverse events in melanoma patients treated with immune checkpoint inhibitors in the adjuvant setting. Melanoma Res 2021;31:371-7.
152. Yoshida T, Ichikawa J, Giuroiu I, et al. C reactive protein impairs adaptive immunity in immune cells of patients with melanoma. J Immunother Cancer 2020;8:e000234.
153. Valpione S, Galvani E, Tweedy J, et al. Immune-awakening revealed by peripheral T cell dynamics after one cycle of immunotherapy. Nat Cancer 2020;1:210-21.
154. Valpione S, Mundra PA, Galvani E, et al. The T cell receptor repertoire of tumor infiltrating T cells is predictive and prognostic for cancer survival. Nat Commun 2021;12:4098.
155. Kao CY, Papoutsakis ET. Extracellular vesicles: exosomes, microparticles, their parts, and their targets to enable their biomanufacturing and clinical applications. Curr Opin Biotechnol 2019;60:89-98.
156. Buzas EI. The roles of extracellular vesicles in the immune system. Nat Rev Immunol 2023;23:236-50.
157. Dou X, Hua Y, Chen Z, Chao F, Li M. Extracellular vesicles containing PD-L1 contribute to CD8+ T-cell immune suppression and predict poor outcomes in small cell lung cancer. Clin Exp Immunol 2022;207:307-17.
158. Serratì S, Guida M, Di Fonte R, et al. Circulating extracellular vesicles expressing PD1 and PD-L1 predict response and mediate resistance to checkpoint inhibitors immunotherapy in metastatic melanoma. Mol Cancer 2022;21:20.
159. Splendiani E, Besharat ZM, Covre A, Maio M, Di Giacomo AM, Ferretti E. Immunotherapy in melanoma: can we predict response to treatment with circulating biomarkers? Pharmacol Ther 2024;256:108613.
160. Wu F, Fan J, He Y, et al. Single-cell profiling of tumor heterogeneity and the microenvironment in advanced non-small cell lung cancer. Nat Commun 2021;12:2540.
161. Tarighati E, Keivan H, Mahani H. A review of prognostic and predictive biomarkers in breast cancer. Clin Exp Med 2023;23:1-16.
162. Sechidis K, Papangelou K, Metcalfe PD, Svensson D, Weatherall J, Brown G. Distinguishing prognostic and predictive biomarkers: an information theoretic approach. Bioinformatics 2018;34:4139.
163. Montaner J, Ramiro L, Simats A, et al. Multilevel omics for the discovery of biomarkers and therapeutic targets for stroke. Nat Rev Neurol 2020;16:247-64.
164. Miñoza JMA, Rico JA, Zamora PRF, et al. Biomarker discovery for meta-classification of melanoma metastatic progression using transfer learning. Genes 2022;13:2303.
165. Chan L, Nadkarni GN, Fleming F, et al. Derivation and validation of a machine learning risk score using biomarker and electronic patient data to predict progression of diabetic kidney disease. Diabetologia 2021;64:1504-15.
166. Chang CH, Lin CH, Lane HY. Machine learning and novel biomarkers for the diagnosis of Alzheimer’s disease. Int J Mol Sci 2021;22:2761.
Cite This Article
Export citation file: BibTeX | EndNote | RIS
OAE Style
Song L, Yang Y, Tian X. Current knowledge about immunotherapy resistance for melanoma and potential predictive and prognostic biomarkers. Cancer Drug Resist 2024;7:17. http://dx.doi.org/10.20517/cdr.2023.150
AMA Style
Song L, Yang Y, Tian X. Current knowledge about immunotherapy resistance for melanoma and potential predictive and prognostic biomarkers. Cancer Drug Resistance. 2024; 7: 17. http://dx.doi.org/10.20517/cdr.2023.150
Chicago/Turabian Style
Lanni Song, Yixin Yang, Xuechen Tian. 2024. "Current knowledge about immunotherapy resistance for melanoma and potential predictive and prognostic biomarkers" Cancer Drug Resistance. 7: 17. http://dx.doi.org/10.20517/cdr.2023.150
ACS Style
Song, L.; Yang Y.; Tian X. Current knowledge about immunotherapy resistance for melanoma and potential predictive and prognostic biomarkers. Cancer Drug Resist. 2024, 7, 17. http://dx.doi.org/10.20517/cdr.2023.150
About This Article
Special Issue
Copyright
Data & Comments
Data
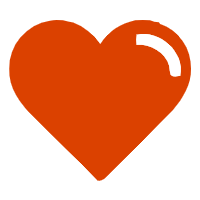
Comments
Comments must be written in English. Spam, offensive content, impersonation, and private information will not be permitted. If any comment is reported and identified as inappropriate content by OAE staff, the comment will be removed without notice. If you have any queries or need any help, please contact us at support@oaepublish.com.