Beyond dopamine: exploring endocannabinoids in Parkinson’s disease
Abstract
Parkinson’s disease (PD) is a prevalent degenerative movement disorder largely attributed to the dysfunction of dopamine transmission in the basal ganglia. However, the role of the endocannabinoid (eCB) system (ECS) in PD pathology and symptomatology is often overlooked in discussions. Recent research, including our own, has identified multiple homozygous loss-of-function variants in diacylglycerol lipase β (DAGLB), an enzyme involved in the synthesis of 2-arachidonoyl-glycerol (2-AG) - the most abundant eCB in the brain - in individuals with early-onset autosomal recessive Parkinsonism. These genetic findings strongly link eCB deficiency with the etiopathogenesis of PD. Exploring the roles of DAGLB and 2-AG signaling in PD and dopamine transmission could provide a new perspective on PD treatments, focusing on the function of the ECS and the pathophysiological implications of its disruption.
Keywords
INTRODUCTION
Parkinson’s disease (PD) ranks as the second most common degenerative neurological disorder after Alzheimer’s disease[1]. As one of the fastest-growing neurological conditions[2], PD affects millions of elderly people worldwide. Persons with PD display progressive motor symptoms, such as resting tremor, slowed movement, impaired posture and balance, and rigid muscles[3]. Additionally, they frequently experience non-motor symptoms including chronic pain, depression, dementia, sleep and autonomic dysfunction, and others[4]. While medications and surgical interventions can enhance motor performance in PD persons, treatments for non-motor symptoms remain limited[5]. Furthermore, long-term medication usage can lead to severe side effects like dyskinesia and impulse control disorders[6,7]. Therefore, there is an ongoing need for new mechanistic insights and therapeutic agents to improve the treatment outcomes for the growing number of PD persons[8].
The nigrostriatal dopaminergic neurons (DANs) play a crucial role in regulating the vigor of movement[9] and motor learning[10]. The activity of nigrostriatal DANs and dopamine release can be dynamically regulated by diverse presynaptic inputs, of which the dopamine receptor D1-expressing direct pathway spiny projection neurons (dSPNs) in dorsal striatum being the major inhibitory contributors[10-14]. The axon terminals of dSPNs are rich in cannabinoid receptor 1 (CB1R)[15,16], which may interact with 2-arachidonoyl-glycerol (2-AG) and anandamide (AEA) released from the nigrostriatal DANs. As retrograde neuromodulators, eCBs suppress presynaptic neurotransmitter release through the G protein-coupled receptor (GPCR) CB1Rs and regulate a variety of physiological processes, such as motor learning, stress response, and memory[17-21]. Midbrain DANs can produce and release eCBs from soma and dendrites[22]. Both diacylglycerol lipase α (DAGLA) and its homolog diacylglycerol lipase β (DAGLB) mediate the biosynthesis of 2-AG in the brain[23]. While DAGLA catalyzes most of the 2-AG production in the brain[24-26], DAGLB appears to be the main 2-AG synthase in nigrostriatal DANs[27].
Confounding upregulation and downregulation of eCBs and receptors have been observed in individuals with PD and related animal models, including genetically modified mice containing PD-related α-synuclein and Parkin mutations, as well as neurotoxin-induced dopamine depletion in rodents and non-human primates, as extensively reviewed previously[20,28,29]. For example, individuals with PD had lower plasma and cerebrospinal fluid (CSF) levels of 2-AG and higher CSF levels of AEA compared to healthy controls[30]. Consistently, overexpression of α-synuclein in rodent DANs also led to a reduction in the 2-AG levels[31]. Therefore, the abnormal reduction of 2-AG levels in the plasma and CSF of individuals with PD could be used as biomarkers for the early detection of PD. However, whether these changes in eCB signaling contribute to the disease or represent compensatory responses remains debated.
Recently, multiple homozygous loss-of-function variants in the DAGLB gene have been associated with early-onset autosomal recessive Parkinsonism[27,32]. These genetic findings provide compelling evidence supporting the role of eCB deficiency in PD pathogenesis. Understanding how the eCB system modulates dopaminergic transmission in motor control and safeguards against DAN degeneration could offer novel insights into the pathogenic mechanisms and treatment strategies for PD.
ENDOCANNABINOID SYSTEM IN THE BRAIN
The endocannabinoid (eCB) system (ECS), which includes eCBs, their receptors, and synthetic and degradation enzymes, stands as one of the most important neuromodulatory systems in the mammalian brain [Figure 1]. The eCBs represent a distinct class of lipid signaling molecules widely distributed in the human body[33,34]. The primary eCB in the central nervous system (CNS) is 2-AG. 2-AG is synthesized from 1-acyl-2-arachidonoyl-sn-glycerol [diacylglycerol (DAG)] through hydrolysis by DAGLA and DAGLB[35]. The degradation of 2-AG mainly occurs through the hydrolysis by monoacylglycerol lipase (MGLL) to arachidonic acid (AA) and glycerol. A small portion is degraded by α/β-hydrolase 6 and α/β-hydrolase 12[36]. The physiological effects of 2-AG are primarily mediated by CB1R and cannabinoid receptor 2 (CB2R)[37,38]. CB1Rs are abundant in the CNS, especially in the basal ganglia, cortex, and hippocampus, predominantly distributed in axon terminals[39,40]. CB2Rs are expressed at very low levels in the brain, limited to specific neurons, and are abundant in activated microglia and astrocytes[41,42].
Figure 1. DAGLB and eCB signaling in DANs. (A) The cartoon illustrates the 3D protein structure of DAGLB predicted by AlphaFold; (B) DAGLB is suggested to be the main synthase of the eCB 2-AG in DANs[27]. The 2-AG produced in the postsynaptic DANs by DAGLB and DAGLA are released into the synaptic cleft upon neuronal activation. They then retrogradely bind to CB1R in the presynaptic sites of DRD1-expressing dSPNs, leading to the suppression of neurotransmitter GABA release. GABA suppresses the activity of DANs and DA release. MGLL, associated with the presynaptic sites, mediates the degradation of 2-AG into AA and glycerol, thereby terminating the
Unlike conventional neurotransmitters and modulators, 2-AG acts as a retrograde messenger and can provide feedback regulation of neurotransmitter release from presynaptic neurons [Figure 1][43]. The production and release of 2-AG are distinctively different from the well-studied amino acid neurotransmitters, such as glutamate and dopamine. Instead of being synthesized, stored, and released,
When CB1Rs on the presynaptic membrane are activated, they inhibit the activity of adenylate cyclase via the inhibitory GPCR-mediated intracellular signaling transduction, resulting in reduced neurotransmitter release from the presynaptic terminals[45]. This regulation exists in various brain regions, such as the cortex, hippocampus, basal ganglia, and cerebellum, participating in synaptic plasticity, regulating learning and memory, drug addiction, and motor learning. Behavioral studies in CB1R knockout mice show that CB1Rs are crucially involved in various forms of learning, including spatial learning[46], fear regulation[47], eyeblink conditioning[48], and habit formation[49,50] through modulating the synaptic transmission and plasticity in hippocampus, amygdala, cerebellum, and basal ganglia.
ECS IN BASAL GANGLIA
In the dorsal striatum, CB1Rs are highly expressed in both dSPN and dopamine receptor D2-expressing indirect pathway projection neurons (iSPNs)[51]. These neurons project to the substantia nigra (SN) and globus pallidus in basal ganglia. The axon terminals of dSPNs, particularly those from the patch (also known as striosome) compartments, form a distinctive striosome-dendron bouquet structure[16]. This structure interacts with the dendrites of DANs that extend into the SNr region[13,16]. Within these striosome-dendron bouquets, CB1Rs are notably enriched in the axon terminals of dSPNs[15]. Moreover, genetic deletion of CB1R in dSPNs affects the synaptic formation with DANs during development[52], suggesting a critical involvement of eCB signaling in the establishment of neural circuitry.
Ex vivo brain slice electrophysiological recordings have found that after depolarization of DANs in the substantia nigra pars compacta (SNc), inhibitory postsynaptic currents decrease, leading to depolarization-induced suppression of inhibition (DSI)[53]. When given a CB1R antagonist AM251, this DSI effect disappears. It is possible that after depolarization of DANs, 2-AG is released to the presynaptic membrane, activating CB1Rs, which reduces gamma-aminobutyric acid (GABA) released by dSPNs, resulting in DSI. When given a CB1R antagonist, 2-AG cannot activate CB1Rs, and DSI is disrupted[53]. Additionally, in vivo experiments in mice have shown that intraperitoneal administration of a CB1R agonist can increase the firing frequency of DANs in the SNc and ventral tegmental area (VTA)[54,55], as well as dopamine release[55,56]. Moreover, in brain slice experiments, local application of a CB1R agonist can increase the single spike firing and burst rate of DANs[57,58], indicating that CB1Rs can regulate the excitability of DANs by modulating presynaptic inputs in the midbrain.
DAGLA AND DAGLB IN THE BRAIN
While both DAGLA and DAGLB contribute to the production of 2-AG, the specific functions of these two enzymes in different cell populations under varying conditions remain an active area of research. In neurons, DAGLA protein is predominantly localized to the postsynaptic sites of dendritic spines, likely due to the interaction between its C-terminal PPxxF domain and the postsynaptic density protein Homer[24,25,59]. The deletion of the Homer-binding domain does not impact the enzymatic activity of DAGLA but disrupts its cell surface and postsynaptic targeting[59]. Furthermore, genetic variants in the DAGLA gene that lead to the truncation of the C-terminal Homer-binding domain have been associated with neuro-ocular abnormalities in affected children[60]. This underscores the importance of proper subcellular targeting for the normal functioning of DAGLA. The DAGLB protein does not possess the homer-binding domain in its
Studies featuring Dagla and Daglb knockout mice have been critical in deciphering which of these two enzymes serves as the main 2-AG synthase across various tissue and cell types. These investigations consistently identify DAGLA as the predominant 2-AG synthase of the brain. Specifically, Dagla knockout mice have been shown to exhibit an 80%-90% reduction in brain 2-AG levels[24]. Additionally, these studies reveal that 2-AG-mediated retrograde feedback inhibition is completely abolished in the cerebellum, hippocampus, and striatum of Dagla knockout mice, as well as stimulus-induced 2-AG production[25]. In contrast, Daglb knockout mice do not show significant differences from control mice in these aspects. Therefore, these early studies do not support a critical role for DAGLB-mediated 2-AG synthesis during synaptic transmission. However, it is worth noting that DAGLB has been shown to regulate axonal outgrowth during neuronal development[65].
DAGLA AND DAGLB IN THE SN AND PARKINSONISM
Although DAGLA is commonly recognized as the main 2-AG synthase in the CNS, both bulk and single-nuclei transcriptomic analyses have revealed that DAGLB mRNA is more abundant than DAGLA in nigrostriatal DANs and other cell types within the SN[27,66,67] [Figure 2]. Furthermore, genetic deletion of Daglb selectively in mouse nigrostriatal DANs markedly reduces the production of 2-AG in the SN[27], supporting the notion that DAGLB is not only enriched in the DANs but also functionally serves as the primary 2-AG synthase in SN.
Figure 2. Expression of DAGLA, DAGLB, and MGLL in human SN region. Dot plot of a previously published single-nuclei transcriptomic dataset from the human SN region[66]. It compares the gene expression levels of DAGLA, DAGLB, and MGLL in type 1 and type 2 astrocytes, DANs, endothelial cells, GABAergic neurons, microglia, and type 1-3 oligodendrocytes, as well as OPCs. The size of each circle indicates the percentage of cells expressing a given gene within the cell population. The color intensity, ranging from light to dark red, represents the increase in gene expression level. DAGLA: Diacylglycerol lipase α; DAGLB: diacylglycerol lipase β; MGLL: monoacylglycerol lipase; SN: substantia nigra; DANs: nigrostriatal dopaminergic neurons; GABA: gamma-aminobutyric acid; OPCs: oligodendrocyte precursor cells.
The expression and functional significance of DAGLB in nigrostriatal DANs may help to explain why multiple loss-of-function variants in DAGLB have been associated with early-onset Parkinsonism[27,32]. These genetic variants either disrupt DAGLB transcription or impair DAGLB protein stability[27], resulting in a complete loss of DAGLB function. It is plausible to suggest that these Parkinsonism-associated DAGLB variants could lead to decreased 2-AG production by nigrostriatal DANs, providing a previously unrecognized pathogenic mechanism for the disease.
This proposed pathogenic mechanism focuses on the reduced activity of nigrostriatal DANs due to the loss of 2-AG-mediated disinhibition of the dSPNs-induced inhibitory inputs to the DANs[27]. The dSPNs exert inhibitory GABAergic inputs onto the nigrostriatal DANs, suppressing their activity and dopamine release[10,11,13]. The axon terminals of the dSPNs are rich in CB1R expression and are susceptible to binding by 2-AG produced and released from the postsynaptic sites of nigrostriatal DANs, which provide negative feedback to reduce the dSPN activity while enhancing the activity of nigrostriatal DANs[15,27].
In the context of DAGLB dysfunction, impaired 2-AG synthesis in nigrostriatal DANs would prevent this disinhibition, potentially contributing to the development of PD-like symptoms. Conversely, augmentation of 2-AG levels by pharmacologically inhibiting its hydrolysis boosts DAN activity and dopamine release, thereby alleviating motor abnormalities[27]. Given the genetic and pathophysiological evidence suggesting that deficiency in DAGLB-mediated 2-AG production contributes to the etiopathogenesis in PD, enhancing 2-AG transmission within the SN may present a viable therapeutic approach for the disease.
ANIMAL MODELS FOR STUDYING THE ECS IN THE BASAL GANGLIA AND PD
While there are a limited number of animal models currently available for exploring the role of eCB signaling in PD pathogenesis, numerous models have been established to investigate the roles of various ECS components in different basal ganglia neuronal populations. For example, the genetic deletion of CB1R specifically in mouse dSPNs resulted in motor impairments like those observed in mice with selective deletion of the Daglb gene in adult nigrostriatal DANs[27]. This finding supports the notion that DAGLB-mediated 2-AG signaling between nigrostriatal DANs and dSPNs plays an important role in motor function, especially the retention of newly acquired motor skills[27]. However, Daglb germline knockout mice, which lack functional DAGLB from the embryonic stage and mimic the loss-of-function genetic variants in PD persons, do not display any noticeable motor phenotypes[24,26,27,35]. This discrepancy highlights the need for further exploration into the roles of DAGLB and 2-AG in motor control, learning, and PD pathogenesis.
In addition to the role of DAGLB-dependent 2-AG production in nigrostriatal DANs and its implication in PD pathogenesis, there are intriguing insights into the interplay between DAGLA-mediated 2-AG and dopamine within the basal ganglia, and their influence on specific behaviors. Targeted knockdown of Dagla gene expression in mouse dSPNs leads to reduced 2-AG levels in the striatum. This results in the loss of
ECS-BASED TREATMENTS IN PRECLINIC PD ANIMAL MODELS
PD treatments that target ECS are relatively limited. Understanding the current state of these therapies can shed light on how DAGLB might enhance these existing methods. Notably, research has explored the potential of another eCB ligand, AEA, for PD treatment. Results have been mixed: while inhibiting AEA hydrolysis in mouse PD models showed no effect on the nigrostriatal pathway or respiratory deficits[71,72], it preserved motor function[71]. However, given our focus on 2-AG, we will concentrate on therapies that are based on modulating 2-AG levels. Many therapies accomplish this through the manipulation of MGLL [Table 1].
List of ECS-based treatments in PD-related animal models
Model | What was administered to the model? | How did the treatment reverse PD-like symptoms in the model? |
Daglb knockdown mouse | JZL-184 (MGLL inhibitor) | Rescued nigral dopamine release and motor skill learning[27] |
MPTPmouse | JZL-184 | Prevented nigrostriatal degeneration by curbing inflammatory responses in astroglia and microglia[76-78]. Preserved motor function as well[78] |
MPTP mouse | URB602 (MGLL inhibitor) | Prevented MPTP-induced nigrostriatal degeneration[76] |
MPTP mouse | DFU (COX-2 inhibitor) | Prevented MPTP-induced nigrostriatal degeneration. Led to an increase in 2-AG levels[76] |
Mgll knockout mouse | LPS | Prevented LPS-induced DAN terminal loss and DA reductions in the SN and striatum[77] |
6-OHDA mouse | KML-29 (MGLL inhibitor) | Prevented 6-OHDA-induced DAN cell death[78] |
MPTP mouse | KML-29 | Prevented MPTP-induced striatal dopamine depletion. Enhanced GDNF mRNA expression[90] |
6-OHDA rat | D9-THC | Prevented 6-OHDA-induced DAN cell death[91] |
MGLL accounts for 85% of 2-AG hydrolysis in the brain and acts as a limiting factor in 2-AG signaling pathways[73,74]. This MGLL-dependent 2-AG hydrolysis serves two primary functions: eCB signaling and neuroinflammation. In terms of eCB signaling, it is important to note that MGLL and the DAGLs are distinct in their expression patterns and subcellular localizations. While MGLL is mainly restricted to presynaptic sites, DAGLs often reside in the postsynaptic density[26,73]. Upon depolarization, postsynaptic DAGLs synthesize 2-AG until it reaches the levels required for 2-AG-mediated synaptic transmission and plasticity. Since MGLL is confined to the presynaptic sites, it does not interfere with this postsynaptic elevation of 2-AG levels. However, MGLL can rapidly hydrolyze 2-AG that arrives at the presynaptic sites, enhancing the temporal specificity of the eCB signals.
MGLL is commonly characterized by its role in neuroinflammatory pathways. It hydrolyzes 2-AG into AA, a precursor for proinflammatory prostaglandins and eicosanoids implicated in the degeneration of nigrostriatal DANs associated with canonical PD neuropathology. That metabolic linkage between MGLL and proinflammatory prostaglandins forms the basis for discussions regarding MGLL as a potential target for innovative PD treatments. For instance, in 1-methyl-4-phenyl-1,2,3,6-tetrahydropyridine (MPTP) mouse models of PD, treatment with MGLL inhibitors such as JZL-184 and URB602 has been shown to offer neuroprotective effects by preventing the MPTP-induced degeneration of dopaminergic neurons in the midbrain[75,76]. This protective effect was also observed in Mgll knockout mice[77]. Likewise, the
Preventing 2-AG hydrolysis undoubtedly elevates overall 2-AG levels throughout the brain[27]. Consequently, it is pertinent to inquire whether this increased 2-AG contributes to the anti-inflammatory effects observed when targeting MGLL. Lipopolysaccharide (LPS)-treated mice demonstrate an increase in brain eicosanoids and prostaglandins that promote inflammation, an increase that was predictably attenuated in both JZL-184-treated and Mgll knockout mice[77]. Disruption to 2-AG signaling, such as using 2-AG antagonists or genetic inactivating CB1R and CB2R receptors, had minimal effect on reducing the levels of proinflammatory agents[77]. Thus, the neuroprotective effects of MGLL inactivation appear to be independent of 2-AG elevation. These effects are more accurately attributed to the reduction in AA and the consequent decrease in proinflammatory prostaglandins, rather than any enhancement of 2-AG signaling pathways.
While 2-AG may not be actively involved in the neuroprotective effects of MGLL inactivation, our research indicates that 2-AG-mediated signaling within the striatonigral pathway is integral to motor function[27]. We demonstrated that viral knockdown of the Daglb gene in nigrostriatal DANs in mice led to reduced 2-AG release from the SN and deficits in motor skill learning. Subsequently, we administered JZL-184 to these mice, anticipating that it would inhibit 2-AG degradation in the SN and alleviate PD-like symptoms. Remarkably, JZL-184 treatment restored nigral dopamine release to levels comparable to control mice and reversed the observed impairments in motor skill learning[27]. In this context, our primary focus is on the impaired ability of DAGLB to synthesize 2-AG, compromising 2-AG signaling pathways essential for regulating nigrostriatal DAN activity through presynaptic inhibition of GABAergic inputs. Inhibiting MGLL with JZL-184 elevates 2-AG levels, contributing to the restoration of SN dopamine release and the recovery of motor skill learning. Therefore, gaining a deeper understanding of DAGLB and its role in regulating nigrostriatal DAN activity and dopamine release could pave the way for novel cannabinoid-based therapies for PD, aiming to restore lost 2-AG signaling pathways to alleviate its symptoms.
It is worth noting that the beneficial effects observed following JZL-184 administration are primarily attributed to the improvement of nigrostriatal DAN activity, since there was no degeneration of DANs in the Daglb-deficient mice[27]. While therapies aimed at restoring lost 2-AG signaling pathways may not be as effective in PD persons with extensive nigrostriatal DAN degeneration, they still hold promise for alleviating symptoms in persons with disease-causing DAGLB variants. Additionally, we speculate that these therapies have the potential to enhance 2-AG signals in the remaining nigrostriatal DANs.
Beyond inhibiting the hydrolysis of 2-AG, various eCB-like compounds and specific CB1R and CBR2 agonists have been explored for their potential beneficial effects in alleviating PD-related motor and non-motor symptoms in clinical trials and preclinical models[79-81]. These efforts have produced mixed results. However, an exploratory clinical trial of eCB-like cannabidiol seems to improve the mobility and mental states of individuals with PD[82].
CONCLUSIONS AND FUTURE PERSPECTIVES
In conclusion, recent advancements in identifying genetic variants in DAGLB causally associated with early-onset autosomal recessive Parkinsonism have illuminated the role of DAGLB-mediated 2-AG biosynthesis in the etiopathogenesis of PD. Compared to the studies on DAGLA, the normal physiological functions of DAGLB in neurons remain less explored. The limited study of DAGLB may be due to its lower expression levels in many neuronal populations compared to DAGLA. However, the increasing availability of single-cell transcriptomic profiling has revealed distinct neuronal subpopulations and non-neuronal cell types in the brain with higher levels of DAGLB expression than DAGLA, including nigrostriatal DANs [Figure 2]. This could help explain how DAGLB deficiency contributes to PD-related motor symptoms. Enhancing the production of 2-AG by nigrostriatal DANs could serve as a potential mechanistic-based therapeutic intervention to boost dopamine release and neuronal activity in persons with preserved nigrostriatal DANs. Indeed, an exploratory clinical trial of an eCB-like cannabidiol has shown promise in improving the mobility and mental states of persons with PD[82].
DAGLA, although a minor 2-AG synthase in nigrostriatal DANs, may also contribute to the 2-AG production in Daglb-deficient DANs. Genetic deletion of both Dagla and Daglb in nigrostriatal DANs may provide a critical means to evaluate the pathophysiological role of DAN-derived 2-AG in motor and non-motor behaviors in PD. Additionally, since 2-AG is implicated in inflammation[83], it is important to further investigate the roles of DAGLB in microglia, oligodendrocytes, and astrocytes by selectively deleting Daglb in these glial cells.
Beyond the brain, eCB signaling has been implicated in inflammatory responses in various parts of the body[83]. For example, 2-AG in myeloid promotes vascular inflammation and atherogenesis[84]. Daglb inactivation in mouse peritoneal macrophages attenuates lipopolysaccharide-induced release of proinflammatory cytokine tumor necrosis factor-a[85]. The underlying molecular and cellular mechanisms may be shared by the immune cells in the brain and provide clues to investigate potential neuroinflammatory reactions. However, given that the inhibition of DAGLB activity counteracts inflammatory responses[85], we posit that DAGLB deficiency is less likely to directly induce the harmful neuroinflammation implicated in the pathogenesis of PD. Nonetheless, future studies will be needed to further elucidate the role of DAGLB and its regulation in microglia or other non-neuronal cells in PD.
Furthermore, recent clinical trials have suggested the potential benefits of cannabinoids in improving the non-motor symptoms of individuals with PD, including mood and sleep[86-88]. Notably, an increasing amount of evidence has indicated that the ECS is associated with the circadian system and sleep, with both ECS ligands and receptors showing diurnal variations and regulating the activity of suprachiasmatic nucleus during the sleep-wake cycle[80]. The underlying neural circuit mechanisms by which ECS modulation improves the mental state and sleep of individuals with PD are of interest for future research.
Compared to PD, there are much fewer studies on the involvement of ECS in other synucleinopathies such as multiple system atrophy (MSA) and dementia with Lewy body, as well as atypical PD, including progressive supranuclear palsy and corticobasal syndrome. A recent study indicates the involvement of ECS in MSA pathology[89]. However, more research is needed to document the changes in the ECS in these disorders.
DECLARATIONS
Authors’ contributions
Provided the conceptual outline, wrote and edited the manuscript, and created the figures: Cai H
Contributed to the writing of the manuscript: Basu D, Yang N
Performed the gene expression analyses and created the graph: Ding J
Edited the manuscript: Wang L, Liu Z, Tang B
Availability of data and materials
Not applicable.
Financial support and sponsorship
This work is supported by the Intramural Research Programs of National Institute on Aging, NIH (HC, ZIA AG000944, AG000928).
Conflicts of interest
All authors declared that there are no conflicts of interest.
Ethical approval and consent to participate
Not applicable.
Consent for publication
Not applicable.
Copyright
© The Author(s) 2024.
REFERENCES
1. Carmichael K, Sullivan B, Lopez E, Sun L, Cai H. Diverse midbrain dopaminergic neuron subtypes and implications for complex clinical symptoms of Parkinson’s disease. Ageing Neurodegener Dis 2021;1:4.
2. Dorsey ER, Sherer T, Okun MS, Bloem BR. The emerging evidence of the Parkinson pandemic. J Parkinsons Dis 2018;8:S3-8.
3. Parkinson J. An essay on the shaking palsy. 1817. J Neuropsychiatry Clin Neurosci 2002;14:223-36; discussion 222.
4. Sveinbjornsdottir S. The clinical symptoms of Parkinson’s disease. J Neurochem 2016;139 Suppl 1:318-24.
6. Vijayakumar D, Jankovic J. Drug-induced dyskinesia, part 1: treatment of levodopa-induced dyskinesia. Drugs 2016;76:759-77.
7. Bastide MF, Meissner WG, Picconi B, et al. Pathophysiology of L-dopa-induced motor and non-motor complications in Parkinson’s disease. Prog Neurobiol 2015;132:96-168.
8. Emamzadeh FN, Surguchov A. Parkinson’s disease: biomarkers, treatment, and risk factors. Front Neurosci 2018;12:612.
9. Dudman JT, Krakauer JW. The basal ganglia: from motor commands to the control of vigor. Curr Opin Neurobiol 2016;37:158-66.
10. Wu J, Kung J, Dong J, et al. Distinct connectivity and functionality of aldehyde dehydrogenase 1a1-positive nigrostriatal dopaminergic neurons in motor learning. Cell Rep 2019;28:1167-81.e7.
11. Watabe-Uchida M, Zhu L, Ogawa SK, Vamanrao A, Uchida N. Whole-brain mapping of direct inputs to midbrain dopamine neurons. Neuron 2012;74:858-73.
12. McGregor MM, McKinsey GL, Girasole AE, Bair-Marshall CJ, Rubenstein JLR, Nelson AB. Functionally distinct connectivity of developmentally targeted striosome neurons. Cell Rep 2019;29:1419-28.e5.
13. Evans RC, Twedell EL, Zhu M, Ascencio J, Zhang R, Khaliq ZM. Functional Dissection of basal ganglia inhibitory inputs onto substantia nigra dopaminergic neurons. Cell Rep 2020;32:108156.
14. Carmichael K, Evans RC, Lopez E, et al. Function and regulation of ALDH1A1-positive nigrostriatal dopaminergic neurons in motor control and Parkinson’s disease. Front Neural Circuits 2021;15:644776.
15. Davis MI, Crittenden JR, Feng AY, et al. The cannabinoid-1 receptor is abundantly expressed in striatal striosomes and striosome-dendron bouquets of the substantia nigra. PLoS One 2018;13:e0191436.
16. Crittenden JR, Tillberg PW, Riad MH, et al. Striosome-dendron bouquets highlight a unique striatonigral circuit targeting dopamine-containing neurons. Proc Natl Acad Sci U S A 2016;113:11318-23.
17. Pacher P, Bátkai S, Kunos G. The endocannabinoid system as an emerging target of pharmacotherapy. Pharmacol Rev 2006;58:389-462.
19. Morena M, Patel S, Bains JS, Hill MN. Neurobiological interactions between stress and the endocannabinoid system. Neuropsychopharmacology 2016;41:80-102.
20. Lovinger DM, Mathur BN. Endocannabinoids in striatal plasticity. Parkinsonism Relat Disord 2012;18 Suppl 1:S132-4.
21. Kano M, Ohno-Shosaku T, Hashimotodani Y, Uchigashima M, Watanabe M. Endocannabinoid-mediated control of synaptic transmission. Physiol Rev 2009;89:309-80.
22. Mátyás F, Urbán GM, Watanabe M, et al. Identification of the sites of 2-arachidonoylglycerol synthesis and action imply retrograde endocannabinoid signaling at both GABAergic and glutamatergic synapses in the ventral tegmental area. Neuropharmacology 2008;54:95-107.
23. Bisogno T, Howell F, Williams G, et al. Cloning of the first sn1-DAG lipases points to the spatial and temporal regulation of endocannabinoid signaling in the brain. J Cell Biol 2003;163:463-8.
24. Gao Y, Vasilyev DV, Goncalves MB, et al. Loss of retrograde endocannabinoid signaling and reduced adult neurogenesis in diacylglycerol lipase knock-out mice. J Neurosci 2010;30:2017-24.
25. Tanimura A, Yamazaki M, Hashimotodani Y, et al. The endocannabinoid 2-arachidonoylglycerol produced by diacylglycerol lipase alpha mediates retrograde suppression of synaptic transmission. Neuron 2010;65:320-7.
26. Reisenberg M, Singh PK, Williams G, Doherty P. The diacylglycerol lipases: structure, regulation and roles in and beyond endocannabinoid signalling. Philos Trans R Soc Lond B Biol Sci 2012;367:3264-75.
27. Liu Z, Yang N, Dong J, et al. Deficiency in endocannabinoid synthase DAGLB contributes to early onset Parkinsonism and murine nigral dopaminergic neuron dysfunction. Nat Commun 2022;13:3490.
28. Pisani V, Madeo G, Tassone A, et al. Homeostatic changes of the endocannabinoid system in Parkinson’s disease. Mov Disord 2011;26:216-22.
29. Cristino L, Bisogno T, Di Marzo V. Cannabinoids and the expanded endocannabinoid system in neurological disorders. Nat Rev Neurol 2020;16:9-29.
30. Marchioni C, Santos-Lobato BL, Queiroz MEC, Crippa JAS, Tumas V. Endocannabinoid levels in patients with Parkinson’s disease with and without levodopa-induced dyskinesias. J Neural Transm 2020;127:1359-67.
31. Kelly R, Bemelmans AP, Joséphine C, Brouillet E, McKernan DP, Dowd E. Time-course of alterations in the endocannabinoid system after viral-mediated overexpression of α-synuclein in the rat brain. Molecules 2022;27:507.
32. Tesson C, Bouchetara MS, Ferrien M, Lesage S, Brice A. Identification of a DAGLB mutation in a non-Chinese patient with Parkinson’s disease. Mov Disord 2023;38:1756-7.
33. Castillo PE, Younts TJ, Chávez AE, Hashimotodani Y. Endocannabinoid signaling and synaptic function. Neuron 2012;76:70-81.
34. Katona I, Freund TF. Endocannabinoid signaling as a synaptic circuit breaker in neurological disease. Nat Med 2008;14:923-30.
35. Oudin MJ, Hobbs C, Doherty P. DAGL-dependent endocannabinoid signalling: roles in axonal pathfinding, synaptic plasticity and adult neurogenesis. Eur J Neurosci 2011;34:1634-46.
36. Blankman JL, Simon GM, Cravatt BF. A comprehensive profile of brain enzymes that hydrolyze the endocannabinoid 2-arachidonoylglycerol. Chem Biol 2007;14:1347-56.
37. Muccioli GG. Endocannabinoid biosynthesis and inactivation, from simple to complex. Drug Discov Today 2010;15:474-83.
38. Howlett AC, Barth F, Bonner TI, et al. International Union of Pharmacology. XXVII. Classification of cannabinoid receptors. Pharmacol Rev 2002;54:161-202.
39. Mackie K. Distribution of cannabinoid receptors in the central and peripheral nervous system. In: Pertwee RG, editor. Cannabinoids. Berlin/Heidelberg: Springer-Verlag; 2005. pp. 299-325.
40. Nyíri G, Cserép C, Szabadits E, Mackie K, Freund TF. CB1 cannabinoid receptors are enriched in the perisynaptic annulus and on preterminal segments of hippocampal GABAergic axons. Neuroscience 2005;136:811-22.
41. Ramirez SH, Haskó J, Skuba A, et al. Activation of cannabinoid receptor 2 attenuates leukocyte-endothelial cell interactions and blood-brain barrier dysfunction under inflammatory conditions. J Neurosci 2012;32:4004-16.
42. Stella N. Cannabinoid and cannabinoid-like receptors in microglia, astrocytes, and astrocytomas. Glia 2010;58:1017-30.
43. Lu HC, Mackie K. An introduction to the endogenous cannabinoid system. Biol Psychiatry 2016;79:516-25.
44. Albarran E, Sun Y, Liu Y, et al. Postsynaptic synucleins mediate endocannabinoid signaling. Nat Neurosci 2023;26:997-1007.
45. Covey DP, Mateo Y, Sulzer D, Cheer JF, Lovinger DM. Endocannabinoid modulation of dopamine neurotransmission. Neuropharmacology 2017;124:52-61.
46. Varvel SA, Lichtman AH. Evaluation of CB1 receptor knockout mice in the Morris water maze. J Pharmacol Exp Ther 2002;301:915-24.
47. Marsicano G, Wotjak CT, Azad SC, et al. The endogenous cannabinoid system controls extinction of aversive memories. Nature 2002;418:530-4.
48. Kishimoto Y, Kano M. Endogenous cannabinoid signaling through the CB1 receptor is essential for cerebellum-dependent discrete motor learning. J Neurosci 2006;26:8829-37.
49. Hilário MR, Clouse E, Yin HH, Costa RM. Endocannabinoid signaling is critical for habit formation. Front Integr Neurosci 2007;1:6.
50. Sagheddu C, Muntoni AL, Pistis M, Melis M. Chapter Seven - Endocannabinoid signaling in motivation, reward, and addiction: influences on mesocorticolimbic dopamine function. Int Rev Neurobiol 2015;125:257-302.
52. Crittenden JR, Yoshida T, Venu S, Mahar A, Graybiel AM. Cannabinoid receptor 1 is required for neurodevelopment of striosome-dendron bouquets. eNeuro 2022;9:ENEURO.0318-21.2022.
53. Yanovsky Y, Mades S, Misgeld U. Retrograde signaling changes the venue of postsynaptic inhibition in rat substantia nigra. Neuroscience 2003;122:317-28.
54. French ED, Dillon K, Wu X. Cannabinoids excite dopamine neurons in the ventral tegmentum and substantia nigra. Neuroreport 1997;8:649-52.
55. Cheer JF, Kendall DA, Mason R, Marsden CA. Differential cannabinoid-induced electrophysiological effects in rat ventral tegmentum. Neuropharmacology 2003;44:633-41.
56. Tanda G, Pontieri FE, Di Chiara G. Cannabinoid and heroin activation of mesolimbic dopamine transmission by a common mu1 opioid receptor mechanism. Science 1997;276:2048-50.
57. Cheer JF, Wassum KM, Heien ML, Phillips PE, Wightman RM. Cannabinoids enhance subsecond dopamine release in the nucleus accumbens of awake rats. J Neurosci 2004;24:4393-400.
58. Cheer JF, Wassum KM, Sombers LA, et al. Phasic dopamine release evoked by abused substances requires cannabinoid receptor activation. J Neurosci 2007;27:791-5.
59. Jung KM, Astarita G, Zhu C, Wallace M, Mackie K, Piomelli D. A key role for diacylglycerol lipase-alpha in metabotropic glutamate receptor-dependent endocannabinoid mobilization. Mol Pharmacol 2007;72:612-21.
60. Bainbridge MN, Mazumder A, Ogasawara D, et al. Rady Children’s Institute for Genomic Medicine; Undiagnosed Disease Network. Endocannabinoid dysfunction in neurological disease: neuro-ocular DAGLA-related syndrome. Brain 2022;145:3383-90.
61. Dong A, He K, Dudok B, et al. A fluorescent sensor for spatiotemporally resolved endocannabinoid dynamics in vitro and in vivo. bioRxiv. [Preprint] Oct 20, 2020 [accessed on 2024 Aug 1]. Available from: https://doi.org/10.1101/2020.10.08.329169.
62. Farrell JS, Colangeli R, Dong A, et al. In vivo endocannabinoid dynamics at the timescale of physiological and pathological neural activity. Neuron 2021;109:2398-403.e4.
63. Malheiro RF, Carmo H, Carvalho F, Silva JP. Cannabinoid-mediated targeting of mitochondria on the modulation of mitochondrial function and dynamics. Pharmacol Res 2023;187:106603.
64. Hempel B, Crissman M, Pari S, et al. PPARα and PPARγ are expressed in midbrain dopamine neurons and modulate dopamine- and cannabinoid-mediated behavior in mice. Mol Psychiatry 2023;28:4203-14.
65. Davies AK, Alecu JE, Ziegler M, et al. AP-4-mediated axonal transport controls endocannabinoid production in neurons. Nat Commun 2022;13:1058.
66. Agarwal D, Sandor C, Volpato V, et al. A single-cell atlas of the human substantia nigra reveals cell-specific pathways associated with neurological disorders. Nat Commun 2020;11:4183.
67. Nichterwitz S, Chen G, Aguila Benitez J, et al. Laser capture microscopy coupled with Smart-seq2 for precise spatial transcriptomic profiling. Nat Commun 2016;7:12139.
68. Shonesy BC, Parrish WP, Haddad HK, et al. Role of striatal direct pathway 2-arachidonoylglycerol signaling in sociability and repetitive behavior. Biol Psychiatry 2018;84:304-15.
69. Augustin SM, Gracias AL, Luo G, Anumola RC, Lovinger DM. Striatonigral direct pathway 2-arachidonoylglycerol contributes to ethanol effects on synaptic transmission and behavior. Neuropsychopharmacology 2023;48:1941-51.
70. Luján MÁ, Covey DP, Young-Morrison R, et al. Mobilization of endocannabinoids by midbrain dopamine neurons is required for the encoding of reward prediction. Nat Commun 2023;14:7545.
71. Escamilla-Ramírez A, García E, Palencia-Hernández G, et al. URB597 and the cannabinoid WIN55,212-2 reduce behavioral and neurochemical deficits induced by MPTP in mice: possible role of redox modulation and NMDA receptors. Neurotox Res 2017;31:532-44.
72. Batista LA, Cabral LM, Moreira TS, Takakura AC. Inhibition of anandamide hydrolysis does not rescue respiratory abnormalities observed in an animal model of Parkinson’s disease. Exp Physiol 2022;107:161-74.
73. Dinh TP, Carpenter D, Leslie FM, et al. Brain monoglyceride lipase participating in endocannabinoid inactivation. Proc Natl Acad Sci U S A 2002;99:10819-24.
74. Makara JK, Mor M, Fegley D, et al. Selective inhibition of 2-AG hydrolysis enhances endocannabinoid signaling in hippocampus. Nat Neurosci 2005;8:1139-41.
75. Fernández-Suárez D, Celorrio M, Riezu-Boj JI, et al. Monoacylglycerol lipase inhibitor JZL184 is neuroprotective and alters glial cell phenotype in the chronic MPTP mouse model. Neurobiol Aging 2014;35:2603-16.
76. Mounsey RB, Mustafa S, Robinson L, et al. Increasing levels of the endocannabinoid 2-AG is neuroprotective in the 1-methyl-4-phenyl-1,2,3,6-tetrahydropyridine mouse model of Parkinson’s disease. Exp Neurol 2015;273:36-44.
77. Nomura DK, Morrison BE, Blankman JL, et al. Endocannabinoid hydrolysis generates brain prostaglandins that promote neuroinflammation. Science 2011;334:809-13.
78. Zanfirescu A, Ungurianu A, Mihai DP, Radulescu D, Nitulescu GM. Targeting monoacylglycerol lipase in pursuit of therapies for neurological and neurodegenerative diseases. Molecules 2021;26:5668.
79. Fernandez-Espejo E, Caraballo I, de Fonseca FR, et al. Cannabinoid CB1 antagonists possess antiparkinsonian efficacy only in rats with very severe nigral lesion in experimental parkinsonism. Neurobiol Dis 2005;18:591-601.
80. Bassi M, Sancesario A, Morace R, Centonze D, Iezzi E. Cannabinoids in Parkinson’s disease. Cannabis Cannabinoid Res 2017;2:21-9.
81. Wang M, Liu H, Ma Z. Roles of the cannabinoid system in the basal ganglia in Parkinson’s disease. Front Cell Neurosci 2022;16:832854.
82. Chagas MHN, Zuardi AW, Tumas V, et al. Effects of cannabidiol in the treatment of patients with Parkinson’s disease: an exploratory double-blind trial. J Psychopharmacol 2014;28:1088-98.
83. Kelly R, Joers V, Tansey MG, McKernan DP, Dowd E. Microglial phenotypes and their relationship to the cannabinoid system: therapeutic implications for Parkinson’s disease. Molecules 2020;25:453.
84. Jehle J, Hoyer FF, Schöne B, et al. Myeloid-specific deletion of diacylglycerol lipase α inhibits atherogenesis in ApoE-deficient mice. PLoS One 2016;11:e0146267.
85. Hsu KL, Tsuboi K, Adibekian A, Pugh H, Masuda K, Cravatt BF. DAGLβ inhibition perturbs a lipid network involved in macrophage inflammatory responses. Nat Chem Biol 2012;8:999-1007.
86. Finseth TA, Hedeman JL, Brown RP 2nd, Johnson KI, Binder MS, Kluger BM. Self-reported efficacy of cannabis and other complementary medicine modalities by Parkinson’s disease patients in colorado. Evid Based Complement Alternat Med 2015;2015:874849.
87. Lotan I, Treves TA, Roditi Y, Djaldetti R. Cannabis (medical marijuana) treatment for motor and non-motor symptoms of Parkinson disease: an open-label observational study. Clin Neuropharmacol 2014;37:41-4.
88. Chagas MHN, Eckeli AL, Zuardi AW, et al. Cannabidiol can improve complex sleep-related behaviours associated with rapid eye movement sleep behaviour disorder in Parkinson’s disease patients: a case series. J Clin Pharm Ther 2014;39:564-6.
89. Yan S, Lu J, Duan B, et al. Quantitative susceptibility mapping of multiple system atrophy and Parkinson’s disease correlates with neurotransmitter reference maps. Neurobiol Dis 2024;198:106549.
90. Pasquarelli N, Porazik C, Bayer H, et al. Contrasting effects of selective MAGL and FAAH inhibition on dopamine depletion and GDNF expression in a chronic MPTP mouse model of Parkinson’s disease. Neurochem Int 2017;110:14-24.
Cite This Article
How to Cite
Download Citation
Export Citation File:
Type of Import
Tips on Downloading Citation
Citation Manager File Format
Type of Import
Direct Import: When the Direct Import option is selected (the default state), a dialogue box will give you the option to Save or Open the downloaded citation data. Choosing Open will either launch your citation manager or give you a choice of applications with which to use the metadata. The Save option saves the file locally for later use.
Indirect Import: When the Indirect Import option is selected, the metadata is displayed and may be copied and pasted as needed.
About This Article
Copyright
Data & Comments
Data
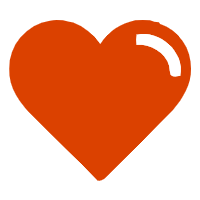
Comments
Comments must be written in English. Spam, offensive content, impersonation, and private information will not be permitted. If any comment is reported and identified as inappropriate content by OAE staff, the comment will be removed without notice. If you have any queries or need any help, please contact us at [email protected].