Advantages and differences among various animal models of Huntington’s disease
Abstract
Treating and preventing neurodegenerative diseases such as Alzheimer’s disease, Parkinson’s disease, dementia with Lewy bodies, amyotrophic lateral sclerosis, frontotemporal dementia, and Huntington’s disease have become significant challenges in the field of neurological research. In the early stages of neurodegenerative disease research, scientists often choose appropriate animal models to delve deeper into their molecular pathological mechanisms and macroscopic clinical manifestations. Selecting the right animal model is a crucial step in initiating and advancing this research process. This article focuses on analyzing various animal models used in the field of neurodegenerative diseases in recent years, with a particular focus on Huntington’s disease. It discusses in detail the advantages and disadvantages of different animal models in experimental research, as well as the pathological features and clinical manifestations they exhibit.
Keywords
INTRODUCTION
Animal models have played a pivotal role in the investigation of various neurodegenerative diseases, offering insights into disease pathogenesis, progression, and potential therapeutic targets. The selection of an appropriate animal model is crucial as different models possess distinct advantages and limitations. It is imperative to carefully consider the characteristics of each model, encompassing similarities in genetic mutations, phenotypic expression, and disease progression, to ensure that the chosen model accurately recapitulates the human disease and is suitable for the specific research objectives. This paper conducts an in-depth analysis of the merits and drawbacks of the diverse animal models extensively employed in Huntington’s disease (HD) research over recent years, encompassing a comprehensive evaluation spanning from large to small animal models. Our endeavor is to equip novices entering the realm of HD research with a visionary perspective by meticulously elucidating the current landscape of animal model utilization in HD studies. Utilizing specialized biological terminology and adjusting phrasing for enhanced professionalism, we have constructed a systematic and comprehensive theoretical framework. This framework aims to serve as a robust theoretical foundation and guiding principles for future research endeavors, enabling them to accurately select the most apt animal model tailored to their specific research objectives.
CHARACTERISTICS OF HD
HD is a dominant genetic disorder caused by mutations in the Huntington gene located on chromosome 4, clinically manifesting as choreiform movements accompanied by progressive cognitive and psychiatric dysfunction, ultimately leading to dementia, and in severe cases, even asphyxia and death. The pathogenic gene Huntingtin (HTT) in HD encodes the HTT protein, and its first exon contains repeated CAG triplets. In HD, the number of CAG triplet repeats abnormally increases, and individuals with more than 36 CAG repeat triplets will develop the disease. The N-terminal fragment of the HTT gene, containing more than 36 polyglutamine (poly Q) repeats in the CAG sequence, forms inclusions within the nucleus and aggregations in nerve fibers. A prominent hallmark of this condition is the formation of aggregates by the mutant HTT (mHTT) protein, which leads to striatal atrophy and significant loss of medium spiny neurons in patients. Currently, there is no effective treatment for HD, and clinical management is primarily focused on symptomatic relief. This ultimately leads to the patient’s demise, occurring approximately 10 to 15 years after the initial signs of the disease manifest[1-3].
The acknowledgment of polyQ’s significance evolved gradually over time. By the late 1990s, researchers had already noted the instability of HTT CAG repeats in human brain tissue post-mortem, as well as in a substantial proportion of rodent models. As investigations deepened, it emerged that the phenotype was primarily dictated by the length of the CAG repeat, rather than the length of the polyQ tract within the Huntington protein[4]. This revelation also sheds light on why certain rodent models, notably YAC128 and early BACHD mice, failed to fully replicate the observed phenotype (owing to the presence of CAA interruptions within the CAG repeats in the original YAC and BAC constructs). These interruptions mitigated the somatic instability of CAG repeats, while both CAA and CAG code for the same amino acid, glutamic acid. A recent pre-print paper from Steve McCarroll’s lab similarly supports the idea that somatic instability plays a key role in HD pathogenesis[5]. Therefore, paying attention to the instability characterization of animal models of different animals is an important aspect in selecting suitable animal models for research.
While scientists have made significant strides in understanding HD, finding effective treatments to halt or slow its progression remains a distant goal. The gradual nature of the disease necessitates extensive and costly clinical trials. Additionally, the limited availability of HD patients for such trials restricts the number of biochemical components that can be tested at any given time. Consequently, animal models occupy a pivotal position in HD research. They serve as a vital gateway before embarking on human clinical trials and as a tool to identify potential therapeutic compounds with promising outcomes.
Animal models of HD have a long history, and existing animal models encompass rhesus monkey, crab-eating macaque, marmoset, sheep, miniature pig, dog, rat, mouse, song bird, frog, zebrafish, fruit fly, roundworm, amoeba, bacteria, budding yeast, etc.[6]. The classification of animal models includes higher mammals, rodents, birds, amphibians and fish, invertebrates, and unicellular microorganisms. These animal models provide researchers with diverse perspectives. It is impractical to provide a comprehensive overview of every animal model in a single article, so we have selected a variety of common large and small animal models for a brief overview, which should meet the needs of most researchers [Figure 1].
Figure 1. Various animal models and modeling methods used in Huntington’s disease research. Common animal models include both large and small animals, which are often created using viruses or plasmids as vectors through injection. The targets of the injection can range from fertilized eggs to the brain.
EARLY ANIMAL MODELS
In the early stages of this disease research, the modeling of HD primarily relied on acute striatal atrophy induced by neurotoxic agents[7-9]. Although striatal atrophy is considered a key neuropathological feature of HD, mHTT is widely expressed throughout the central nervous system and can also be found in peripheral tissues[10,11]. Therefore, models centered solely on acute striatal damage are insufficient to comprehensively reveal the complex manifestations of the disease in these aspects. The limited pathological scope of striatal lesions makes it difficult to replicate many HD neurological symptoms caused by lesions in other brain regions in current disease models. In recognition of this, researchers have begun to shift their modeling focus toward the development of genetic animal models, aiming to delve deeper into the essence of the disease.
CAENORHABDITIS ELEGANS MODELS
The Caenorhabditis elegans (C. elegans) nematode, a non-parasitic species that primarily feeds on bacteria, boasts a nervous system that has been comprehensively and meticulously described. Each neuron’s position and connectivity within this system have been accurately mapped, providing a detailed understanding of its neurobiological structure[12]. Remarkably, C. elegans maintains its transparency throughout its entire lifecycle, enabling the utilization of fluorescent markers for precise tracking and analysis. Its small size further facilitates non-invasive optical monitoring and manipulation techniques, enabling researchers to delicately observe and manipulate its biological processes[13]. Moreover, the fully sequenced genome of
In the experiment, the edited progeny of C. elegans can be obtained by injecting edited gene fragments into the gonads of C. elegans with plasmids as carriers[15]. In addition, a diverse group of scientists have made concerted efforts to unpack the molecular biology behind HD by expressing polyQ tracts with varying repeat lengths in specific neuronal subtypes of C. elegans. A pivotal aspect of their methodology involves integrating amino acids sourced from both standard and amplified human HTT with fluorescently tagged proteins in the touch receptor neurons of C. elegans. This enables them to corroborate the formation of protein aggregates and other pertinent clinical manifestations[16-18].
Even though there is no HTT orthologue present in C. elegans, HTT can be expressed in C. elegans using a transgenic formula to induce age-related mechanosensory impairment, neuronal dysfunction, and neurodegeneration under sensitized conditions[19]. C. elegans has been widely used to model several aspects of polyQ cytotoxicity and to identify novel disease-modifying factors. Not only that, they are also used in classical genetic screening. In the study by Faber et al., through genetic screening techniques, the C. elegans model was used to identify proteins that protect neurons from the toxic effects of extended polyQ tracts[20]. These models have also been extensively employed to delve into the underlying mechanisms of HD and rigorously evaluate the therapeutic promise of diverse compounds. Voisine et al. used an invertebrate model instead of a mammalian model to shorten the testing time from months to days, revealing the neuroprotective effects of lithium chloride and mithramycin in HD[21]. Other substances found to help prevent HD based on the model include rutin[22-24], xyloketal B[25], and diphenyl diselenide[26].
Indeed, the C. elegans serves as an outstanding research platform at the molecular level. Nevertheless, the drawbacks of these models are quite evident. Chief among them is the inability to replicate identical clinical symptoms observed in humans, given that C. elegans lacks peripheral tissues resembling those of humans and possesses a central nervous system vastly different from humans. Consequently, it is infeasible to inform a patient that a potential therapy deemed effective in a nematode can be directly translated into human therapy.
DROSOPHILA MELANOGASTER MODELS
The Drosophila melanogaster (D. melanogaster) has served as a genetic model system for scientific research for over a century. Due to its low maintenance and rapid reproduction costs, researchers have developed numerous genetic techniques over the years[27]. The high degree of genetic homology between
Despite the absence of an expanded polyQ sequence in the amino-terminal domain of D. melanogaster’s HTT protein, there exists a remarkable sequence conservation with the human protein, exhibiting approximately 49% positive homology and 27% identity[39]. This conserved sequence predicts a shared folding pattern between the two proteins. As a result, Drosophila serves as a valuable model organism for studying HD. By introducing mutated human HTT genes into Drosophila, researchers can explore the consequences of these mutations on protein function and interactions within the cell. When constructing
A pivotal stage in the development of HD involves the cleavage of the full-length HTT protein. This process liberates N-terminal fragments of varying lengths, containing an elongated polyQ tract that ultimately becomes neurotoxic in neuronal cells, contributing significantly to the pathogenesis of the disease[43]. The production of both N-terminal and C-terminal fragments, the latter arising specifically from double proteolysis, significantly augments toxicity mechanisms in HD. These byproducts contribute to the dilation of the endoplasmic reticulum (ER) and intensify ER stress, thereby impairing the crucial activity of dynamin 1 protein and ultimately triggering cell death. It was experimentally demonstrated that the N-terminal and C-terminal metabolites produced by HTT protein hydrolysis in Drosophila model exhibited toxicity in the organism[39].
Transgenic D. melanogaster has proven effective in simulating some of the core features of HD, including a significantly shortened lifespan, the late-onset nature of the illness, motor dysfunction, and the gradual accumulation of aggregates within neurons, axons, and the cytoplasm. Chongtham et al. investigated the role of changes in gut microbiota composition in the pathogenesis of several neurodegenerative disorders, with a particular focus on the progression of HD. Using transgenic D. melanogaster models expressing full-length or N-terminal mutant fragments of human HTT protein, the study aimed to explore how gut bacteria influence the progression of HD[44]. The drosophila model has also been used to evaluate potential treatments in HD, with a 2018 study showing that Rhodiola rosea improved several measures in flies expressing HTTQ93[45].
These models enable researchers to gain insights into the disease’s pathogenesis and progression, thereby facilitating the development of potential therapeutic strategies. Moreover, this approach allows researchers to investigate the effects of different regions of the HTT protein and their contribution to the pathogenesis of HD. By studying these models, scientists can gain insights into the molecular mechanisms underlying the disease and potentially develop new therapeutic strategies.
ZEBRAFISH MODELS
With its unique advantages of large embryonic size, short developmental time, and transparency, the zebrafish has emerged as a popular model organism in vertebrate developmental studies. The large size of its embryos facilitates ease of observation and manipulation, while the short developmental cycle allows for the rapid exhibition of various changes throughout development[46]. Furthermore, the transparency of the zebrafish embryo enables the direct observation of internal structures and cellular dynamics without the need for special treatments. These characteristics collectively contribute to the zebrafish's status as an ideal choice for studying the developmental mechanisms of vertebrates, providing scientists with valuable experimental materials and observation windows[47].
The HTT homolog in zebrafish is similar in size to human HTT and exhibits a high degree of identity, reaching up to 70% in amino acid sequence[48]. From a holistic perspective, the absence of HTT has a significant impact on the developmental process of zebrafish embryos[49]. This discovery further emphasizes the crucial role of HTT in vertebrate development and provides strong support for utilizing zebrafish as a model for studying neurodegenerative diseases such as HD. In the zebrafish system, expressing normal and expanded polyQ fragments of HTT through gene editing techniques has become a commonly adopted research method[50]. In these cell lines, the length of the polyQ fragment is closely associated with its misfolding, oligomerization, aggregation, and toxic manifestations, which are highly consistent with observations in other animal models and patients. Insufficient expression of HTT in zebrafish can lead to various developmental defects and disruption of iron homeostasis[49]. Furthermore, recent studies have demonstrated that phosphodiesterase 5 (PDE5) inhibitors upregulate intracellular cGMP levels, resulting in a reduction of aggregates with 71 glutamine repeats in zebrafish larvae expressing human HTT[51].
When exploring the complex pathological mechanism of HD, a crucial molecule, brain-derived neurotrophic factor (BDNF), has entered the field of view of scientists, and several research teams have tried to unravel the mechanism of BDNF in HD through zebrafish models. BDNF, an integral member of the neurotrophin family, exhibits a notable diversity in its isoforms, with three distinct variants identified in zebrafish[52]. The initiation of BDNF signaling, a pivotal process in neuronal health, commences with BDNF transcription, which is disrupted in HD. This transcriptional disruption stems from a decreased BDNF level, a consequence of the CAG repeat expansion in the HTT. Specifically, the CAG repeat in HTT disrupts the normal interaction between BDNF and RE1-silencing transcription factor (REST). This disruption facilitates REST’s nuclear translocation, leading to a suppression of BDNF expression[53]. This downregulation of BDNF, in turn, has detrimental effects on spiny projection neurons, a crucial neuronal population in HD. Furthermore, HTT interferes with the axonal transport of BDNF-containing vesicles. This interference, likely due to altered binding dynamics between dynactin, microtubules, and other components of the transport machinery[54], further exacerbates the deficit in BDNF signaling within HD-affected neurons [Figure 2]. Thus, multiple mechanisms converge to impair BDNF signaling in HD, underlining its central role in disease progression and highlighting potential therapeutic targets.
Figure 2. mHTT competitively inhibits the release of BDNF, which causes nerve damage. mHTT: Mutant Huntingtin; BDNF: brain-derived neurotrophic factor.
Zebrafish model has been playing an important role in the field of HD research, and scientists expect to further study the pathogenesis and development model of HD through this model. However, due to the lack of some important human tissues and organs, resulting in a very different drug delivery route from humans, researchers usually choose rodent models when studying the clinical manifestations and pathological characteristics of HD.
RODENT MODELS
For a long time, and even now, many scientists have created rodent models of HD by injecting acute striatal damage caused by chemical toxins. Intraventricular injection of quinolinic acid, an NMDA receptor agonist, can replicate the striatal histopathology of HD, making it one of the most popular methods for HD modeling. However, the nature of the pathogenesis of this modeling method is not the same; perhaps we can observe similar clinical manifestations with HD, but can not deeply study the pathogenesis of HD at the molecular level.
With the completion of the Human Genome Project and the sequencing of the Mus musculus genome, researchers have discovered significant genetic homology between these species. Concurrently, the rapid advancements in biotechnology and the successful breeding of numerous knockout and transgenic mouse strains have established mice as the most widely used model organisms for studying human diseases. Furthermore, from practical and economic perspectives, mice are favored by scientists due to their small size, rapid reproduction rate, and ease of manipulation, making them important experimental subjects. Currently, the rodent models used in HD research are mainly divided into Truncated N-terminal fragment models and Full-length HD models. The latter is divided into knock-in models and transgenic models [Table 1].
Application of a partial rodent model to HD
Model | Transgene product | Phenotype | Survival | Ref. | |
Neuropathology | Survival and general observations | ||||
Truncated N-terminal fragment models | |||||
N171-82Q | 171 amino acids of N-terminal fragment | Internal inclusion bodies and neuritis aggregates | Progressive weight loss, as well as tremor and hypokinesis, and clasping, progressive accelerated rotation | 20-24 weeks | [55] |
R6/1 | 67 amino acids of N-terminal fragment (116 CAG) | Progressive brain atrophy Decline in the number of neurons | Late stage weight loss Progressively asymmetric growth. Less anxiety phenotype. Gait abnormalities | 32-40 weeks | [56,57] |
R6/2 | 67 amino acids of N-terminal fragment (144 CAG) | Decreased striatal and brain size | Accelerated rotation deficits, increased limb movement, decreased grip strength, hovering behavior, cognitive deficits, progressive weight loss | 12-15 weeks | [57,58] |
HD51 | N-terminal fragment (22% of rat HTT gene) | Significant nuclear aggregates and inclusion bodies | Weight loss, reduced anxiety-like behavior, cognitive decline and progressive motor impairment. Involuntary movements of the head defined by abrupt, rapid, brief, and unsustained irregular movements of the neck | 98 weeks | [59-61] |
N118-82Q | 118 amino acids of N-terminal fragment | \ | Premature death and impaired locomotor coordination | 28-40 weeks | [62,63] |
N586-82Q | 586 amino acids of N-terminal fragment | \ | Progressive decline | 32-36 weeks | [64,65] |
Full-length HD models: transgenic models | |||||
HD89 | Full-length human HTT | Neuronal loss and astrocytoma | Clasping, hyperactive, unilateral rotation, excessive grooming. Decrease in exploratory and locomotor activity | Normal lifespan | [66] |
YAC128 | Full-length human HTT | Reduced striatum and cortex volume, Neuron loss and dysfunction | Hyperkinetic, accelerated rotarod deficit, progressive cognitive deficits | Normal lifespan | [67-71] |
BACHD | Full-length human HTT | Delayed atrophy of the lamina and striatum | Weight gain of 20%-30%, subtle but significant motor impairment initially | Normal lifespan | [60] |
Full-length HD models: knock-in models | |||||
Hdh4/Q72, Hdh4/Q80 | Full-length chimeric human HTT exon 1:mouse HTT | Increased levels of benzodiazepine receptor binding sites in the striatum and cerebral cortex | Increased male aggression and a lesser extent in females | Normal lifespan | [72,73] |
HdhQ111 | Full-length chimeric human HTT exon 1:mouse HTT | Changes in striatal neurons | Decrease in locomotor activity, hyperactivity. Gait abnormalities | Normal lifespan | [74-76] |
HdhQ140 | Full-length chimeric human HTT exon 1: mouse HTT | Striatal HTT nuclear staining. Nuclear and neuropil aggregates prominent in the striatum, nucleus accumbens, and olfactory tubercle, as well as widespread nuclear staining including cortical layers II/III, and V., hippocampus, and regions of the olfactory system | Progressive decrease in motor activity, Abnormal gait | Normal lifespan | [74] |
HdhQ150 | Full-length chimeric human HTT exon 1: mouse HTT | Striatal nuclear HTT immunoreactivity. Striatal NIIs. Reactive astrocytic gliosis. Robust striatal NIIs and striatal neuron receptor loss. Loss of striatal perikarya and volume. Decrease in striatal dopamine D1 and D2 receptors and dopamine transporters | Gradual weight loss, decreased motor activity, motor impairment on the accelerated rotarod, abnormal gait, clasping | Normal lifespan | [77-79] |
Compared to toxin-induced models, genetic models offer a more specific and in-depth understanding of the behavioral and physiological progression of HD phenotypes[80]. Among them, the R6/2 transgenic mouse model stands out as one of the most commonly used, characterized by its aggressive and rapidly progressing phenotype with a short lifespan of approximately 15 weeks[57]. These mice begin to exhibit symptoms such as irregular gait, clasping of hind limbs, weight loss, increased grooming behavior, and cognitive decline at around 5 weeks of age. Furthermore, as transgenic mice age, they become increasingly prone to seizures, further mimicking the complex pathological processes of HD. The N171-82Q model incorporates specific fragments of the HD gene’s
The above two rodent models are N-terminal models. One of the limitations of utilizing N-terminal models is their inability to be studied over an extended period due to their relatively short lifespan. Additionally, they do not encompass the full-length gene, thereby lacking crucial regulatory elements that may contribute to a comprehensive understanding of the disease. Full-length models, such as YAC128 or BACHD, offer new perspectives on disease research by introducing complete human mHTT transgene. The YAC128 mouse model is particularly unique in that it carries 128 CAG repeats from the human HTT gene[67], which more accurately simulates the disease state. These mice usually begin to show abnormal behavior at about 6 months of age, and by about
Knock-in models differ from transgenic models mainly in that they can express specific mutations in endogenous genomic locus rather than through the introduction of foreign genes. Multiple KI models based on CAG repeat length have been successfully created by replacing alleles in the first exon of the mouse HTT gene with human mutated variants. Because KI models are able to more accurately simulate the genetic background of human HD, they exhibit a high degree of phenotypic similarity to human HD. In addition, KI models typically have a longer lifespan and are capable of progressively exhibiting disease-related symptoms, which makes them extremely valuable in HD research, especially as they provide an ideal platform for evaluating the potential of long-term stem cell transplantation therapy.
In rodent models of different genotypes, scientists made a number of key discoveries. Geraldine Kong et al. confirmed the presence of gut dysbiosis in HD by observing the intestinal microbiome composition characteristics of the R6/1 transgenic mouse model of HD[83]. By studying the symptoms of R6/2 and Q175 HD mouse models, Tong et al. revealed that astrocytes and Kir4.1 channels were therapeutic targets[84]. Abjean et al. studied the symptoms of Hdh140 mice, confirming that the JAK2-STAT3 pathway controls the beneficial protein-homeostasis response of reactive astrocytes in HD[85]. HD animal models have also played a huge role in the field of therapeutic environments and drug interventions. Ona et al. found that inhibiting caspase-1 slowed disease progression in a mouse model of Huntington’s disease[86]. van Dellen et al. used HD R6/1 mice to demonstrate that exposure to a stimulation-rich environment from an early age helps prevent brain volume loss and delays the onset of dyskinesia[87]. Ferrante et al. proposed that creatine has a neuroprotective effect on transgenic mouse models of HD[88]. The utilization of the YAC128 model has demonstrated the persistence of mHTT inclusions even after the manifestation of behavioral and neuropathological alterations[67].
Transgenic full-length models, transgenic fragments, and knock-in models have all demonstrated significant effectiveness in their construction, effectively expressing the expanded polyQ repeat domain, which is a core factor in neurodegeneration in HD and other polyQ diseases. Additionally, the high similarity between the huntingtin protein and its homolog in mice suggests that they likely share similar functional properties. To increase the likelihood of predicting human outcomes from model results, the selection of appropriate rodent genetic HD models can be evaluated using the three criteria proposed by Paul Willner[89]. When planning an investigation, in addition to clarifying the research objectives, it is crucial to carefully consider the duration of the study, the achievable sample size, and the degree of relevance between the content studied and human HD in biochemical and behavioral aspects. Furthermore, the issues of consistency and stability arising from genetic variations introduced by breeding and background strains are also important considerations that cannot be overlooked.
SHEEP MODELS
Despite the numerous advantages of rodent models, their brains do not exhibit apoptosis or significant neurodegenerative changes, which are crucial pathological features in human diseases. This is one of the key issues that cannot be avoided when discussing whether to choose rodent models as the subject of research. Therefore, scientists have gradually shifted their focus to larger animal models.
Jacobsen et al. developed a transgenic sheep model of HD to demonstrate the preclinical stage of the disease, aid in the study of early molecular and neuropathological changes occurring in Huntington’s chorea, and test potential therapeutic approaches. The HD sheep were successfully developed by inserting the full-length human HTT cDNA containing 73 CAG repeats into a pronucleus, and then implanting this pronucleus into surrogate sheep[90]. The transgenic sheep G0/5 strain exhibited significant expression of mutant HTT mRNA and protein throughout their entire brain. Clinically, this model primarily manifests as early-onset abnormalities in circadian rhythm, despite the absence of significant pathological changes in the brain[91,92].
However, the transgenic sheep model exhibits only mild neuropathological changes and lacks significant clinical phenotypic features, making it difficult to delve deeper into the impact of observed HTT reduction on disease progression. Additionally, due to the long gestation period (approximately 4. 7 to 5 months) and lifespan of sheep, as well as their seasonal breeding habits, which limit the number of offspring produced each year to 1 to 3, the time required to develop and study sheep populations and collect relevant data is significantly longer compared to smaller experimental animals. This not only increases the pressure on research funding and publication, but also results in a longer time frame for making significant contributions to the field, posing significant challenges to research progress and output.
NON-HUMAN PRIMATE (NHP) MODELS
By utilizing lentiviral vector technology, the team successfully introduced a transgenic fragment of human HTT exon 1 containing 84 CAG repeats into monkeys, establishing the first transgenic monkey model of HD. All five live-born neonates carried the transgenic mutant HTT and GFP genes. Their pathogenesis closely resembled that of humans: monkeys carrying a CAG repeat sequence below the threshold exhibited normal health, while those with a higher number of CAG repeats developed symptoms that positively correlated with the degree of repeat expansion. The main clinical manifestations included motor dysfunction and premature death[93].
Subsequent in-depth research revealed that this monkey model not only spontaneously exhibited pathological manifestations such as motor dysfunction and epileptic seizures, but also gradually developed progressive cognitive decline. These features closely resemble the chronic course of adult HD, and the model exhibited particularly prominent HTT aggregates and axonal degeneration[94,95]. Compared to rodent models, the monkey model exhibited more severe phenotypic characteristics and a significantly shorter survival time. This phenomenon may stem from the higher sensitivity of primate neurons to toxic HTT protein fragments[96]. Therefore, this transgenic monkey model not only provides us with a research platform that is closer to the pathological process of human HD, but also offers valuable insights for our understanding of the pathogenesis of HD and the exploration of potential treatment strategies.
Similar to the sheep model, the monkey model also has a long gestation period, and even worse, they typically only have one offspring per pregnancy[97]. Coupled with the incomplete development of transgenic technology in monkeys, the creation of a monkey model is inefficient. These factors significantly increase the economic cost and research cycle of the study. Unlike other experimental animals, ethical considerations for non-human primates are much stricter, which greatly limits our ability to conduct more in-depth research on HD using monkey models[98].
PIG MODELS
In order to solve the problems in the above animal models, pig models have gradually entered the field of view of researchers. The neural anatomical structure of pigs is similar to that of humans. For example, they have a gyrus structure similar to that of humans. The dorsal striatum of pig brain is divided into two different structures by the internal capsule, just like that of humans. The sensory cortex of pigs, such as the motor cortex and somatosensory cortex, is arranged according to the position of the body, which is not found in rodent models [Figure 3]. Pigs also have many genetic and reproductive advantages that do not exist in primates, including a relatively short gestation period, multiple births, and a short time to sexual maturity. In addition, as an important part of the world's livestock industry, pigs are relatively easy to raise and the cost is relatively low.
In 2010, Yang et al. collaborated to establish the first transgenic HD pig model with the N-208-105Q mutation. This groundbreaking work revealed for the first time that the same mutant protein can induce cell apoptosis in the brains of pigs, a phenomenon that had not been observed in mouse brains previously. Furthermore, the model exhibited notable symptoms such as convulsions and choreiform movements. Unfortunately, transgenic pigs previously obtained through specific methods faced the challenge of extremely short survival times due to the severity of their clinical symptoms, greatly limiting further in-depth research[99]. Given this, the aforementioned research team once again collaborated to successfully construct an HD-KI gene knock-in pig model in an effort to overcome this challenge. This HD-KI pig model exhibited specific neuronal death in the striatum, highly similar to the pathological changes observed in HD patients. Additionally, researchers observed a clear process of cell apoptosis. Notably, the HD-KI pigs also exhibited clinical symptoms such as abnormal gait and respiratory difficulties, which are consistent with the manifestations seen in human HD patients[100]. Crucially, the gene knock-in pig model possesses the ability to stably transmit genetic and phenotypic traits to its offspring, maximizing the reproductive and genetic advantages of the pig model. This provides us with a consistent and stable research platform to further explore the pathogenesis of HD and potential treatment strategies. The HD-KI pig, with its remarkably similar clinical manifestations to humans and its low-cost feeding model, is poised to become one of the essential platforms for translating experimental research into clinical applications in the treatment of HD.
In addition, among numerous HD animal models, especially in pigs, longer CAG repeat lengths may be required to maintain severe disease phenotypes, which are less common in human cases. Despite the fact that genomic editing techniques, particularly CRISPR-Cas9, have revolutionized the field of animal modeling, including HD research, they also exhibit certain limitations. One notable concern is the potential for off-target effects, leading to unintended genomic alterations. Furthermore, CRISPR/Cas9 may also induce mosaicism, which is particularly significant in large animal models and studies that involve founder animals. These limitations have the potential to confound the interpretation of phenotypes observed in generated animal models.
PIG MODELS ARE BECOMING INCREASINGLY IMPORTANT
Different animals provide unique advantages as research platforms for HD, but their limitations cannot be ignored. While models such as C. elegans, D. melanogaster, and zebrafish allow us to easily observe molecular-level manifestations and pathological features similar to humans, such as premature death and progressive neurodegeneration, researchers are unable to observe any clinical symptoms that appear in humans, such as choreiform movements [Table 2]. Observing clinical manifestations in rodent and large animal models is generally a better choice because they possess highly homologous genes to humans and have more complex nervous systems. However, it is important to note that the choice of animal as the research subject, as well as the decision to use gene knockout or transgenic methods to create the model, can significantly affect the clinical symptoms observed.
Comparison of various animal models in HD
Species | Molding method | Pathological and clinical symptoms | Disadvantage | Ref. |
C. elegans | Expressing fluorescently labeled polyQ proteins in neurons or muscle cells | Motility defects or ASH neurodegeneration | No pathologic processes and clinical symptoms; No orthogonal HTT | [17,101] |
D. melanogaster | Using the GAL4/UAS (upstream activating sequence) system | Cytopathia caused by polyQ; The course of the disease is delayed and progressive; Loss of motor function, metabolic imbalance and premature death | A winged arthropod; differences in morphological development and movement patterns | [102-109] |
Zebrafish | Genetic model (HTT) | Morphological abnormalities and necrosis of the central nervous system | Lack of certain vital human tissues and organs;Controlled route of administration | [110,111] |
In HD, we have to stress the importance of pig models in current research progress. As a model with the advantages of low-cost feeding, high litter size, and short gestation period, its advantages are particularly prominent in the brain gyrus structure similar to that of humans, and it has the same progressive characteristics, premature death, and various behavioral disorders as humans. In the widely used HD-KI model, we can achieve efficient gene editing by injecting adenoviral vectors carrying gene fragments into the brains of Cas9-expressing pigs. This method perfectly reproduces the genetic manifestations of autosomal dominant inherited diseases. Because their offspring have a high survival rate and can be stably transmitted, researchers can more easily formulate reliable long-term experimental plans for behavioral analysis and the like.
PERSPECTIVES
Regardless of the animal model chosen for research, the ultimate goal is always to serve clinical practice, aiming to provide beneficial insights and methods for the treatment of human diseases. Particularly in the study of HD, monkey and pig models have become increasingly popular and widely adopted due to their significant physiological similarities to humans. Utilizing these stable and reliable animal models to delve deeper into the pathophysiological mechanisms of HD from a developmental perspective holds immense research promise, undoubtedly ushering in a new era for HD research.
Furthermore, the rapid advancements in gene therapy and editing techniques have increasingly unlocked their potential to correct genetic defects during embryogenesis. As gene therapy gains wider acceptance, these technological advancements have sparked hope for correcting inherited diseases before birth. Therefore, by exploring the role of early HD neurodevelopmental disorders in the progression of the disease, we can not only pave new research directions for a more comprehensive understanding of HD, but also establish a solid foundation for exploring novel therapeutic strategies for HD.
DECLARATIONS
Authors’ contributions
Wrote the review paper: Yu Z
Revised manuscript: Yang W, Yan S
Conceived and designed experiments: Yan S, Yu Z
All authors read and approved the final manuscript.
Availability of data and materials
Not applicable.
Financial support and sponsorship
This work was supported by the National Key Research and Development Program of China (2021YFA0805300) and the National Natural Science Foundation of China (82171244). Sincerely acknowledges the support of K. C. Wong Education Foundation.
Conflicts of interest
Yan S is a guest editor of the journal Ageing and Neurodegenerative Diseases, while the other authors have declared that they have no conflicts of interest.
Ethical approval and consent to participate
Not applicable.
Consent for publication
Not applicable.
Copyright
© The Author(s) 2024.
REFERENCES
4. Genetic Modifiers of Huntington’s Disease (GeM-HD) Consortium. CAG repeat not polyglutamine length determines timing of Huntington’s disease onset. Cell 2019;178:887-900.e14.
5. Handsaker RE, Kashin S, Reed NM, et al. Long somatic DNA-repeat expansion drives neurodegeneration in Huntington disease. bioRXiv. [Preprint.] May 20, 2024 [accessed 2024 Aug 6]. Available from: https://www.biorxiv.org/content/10.1101/2024.05.17.592722v1.
6. DiFiglia M, Leavitt BR, Macdonald D, Thompson LM; Huntington’s Disease Nomenclature Working Group. Towards standardizing nomenclature in Huntington’s disease research. J Huntingtons Dis 2024;13:119-31.
7. Ferrante RJ, Kowall NW, Cipolloni PB, Storey E, Beal MF. Excitotoxin lesions in primates as a model for Huntington’s disease: histopathologic and neurochemical characterization. Exp Neurol 1993;119:46-71.
8. DiFiglia M. Excitotoxic injury of the neostriatum: a model for Huntington’s disease. Trends Neurosci 1990;13:286-9.
10. Chuang CL, Demontis F. Systemic manifestation and contribution of peripheral tissues to Huntington’s disease pathogenesis. Ageing Res Rev 2021;69:101358.
11. Weiss A, Träger U, Wild EJ, et al. Mutant huntingtin fragmentation in immune cells tracks Huntington’s disease progression. J Clin Invest 2012;122:3731-6.
12. Sulston JE. Neuronal cell lineages in the nematode Caenorhabditis elegans. Cold Spring Harb Symp Quant Biol 1983;48:443-52.
13. Gonzalez-Moragas L, Roig A, Laromaine A. C. elegans as a tool for in vivo nanoparticle assessment. Adv Colloid Interface Sci 2015;219:10-26.
14. The C. elegans Sequencing Consortium. Genome sequence of the nematode C. elegans: a platform for investigating biology. Science 1998;282:2012-8.
15. Rieckher M, Tavernarakis N. Generation of Caenorhabditis elegans transgenic animals by DNA microinjection. Bio Protoc 2017;7:e2565.
16. Parker JA, Connolly JB, Wellington C, Hayden M, Dausset J, Neri C. Expanded polyglutamines in Caenorhabditis elegans cause axonal abnormalities and severe dysfunction of PLM mechanosensory neurons without cell death. Proc Natl Acad Sci U S A 2001;98:13318-23.
17. Morley JF, Brignull HR, Weyers JJ, Morimoto RI. The threshold for polyglutamine-expansion protein aggregation and cellular toxicity is dynamic and influenced by aging in Caenorhabditis elegans. Proc Natl Acad Sci U S A 2002;99:10417-22.
18. Satyal SH, Schmidt E, Kitagawa K, et al. Polyglutamine aggregates alter protein folding homeostasis in Caenorhabditis elegans. Proc Natl Acad Sci U S A 2000;97:5750-5.
19. Faber PW, Alter JR, MacDonald ME, Hart AC. Polyglutamine-mediated dysfunction and apoptotic death of a Caenorhabditis elegans sensory neuron. Proc Natl Acad Sci U S A 1999;96:179-84.
20. Faber PW, Voisine C, King DC, Bates EA, Hart AC. Glutamine/proline-rich PQE-1 proteins protect Caenorhabditis elegans neurons from huntingtin polyglutamine neurotoxicity. Proc Natl Acad Sci U S A 2002;99:17131-6.
21. Voisine C, Varma H, Walker N, Bates EA, Stockwell BR, Hart AC. Identification of potential therapeutic drugs for huntington’s disease using Caenorhabditis elegans. PLoS One 2007;2:e504.
22. Cordeiro LM, Machado ML, da Silva AF, et al. Rutin protects Huntington’s disease through the insulin/IGF1 (IIS) signaling pathway and autophagy activity: study in Caenorhabditis elegans model. Food Chem Toxicol 2020;141:111323.
23. Cordeiro LM, Soares MV, da Silva AF, et al. Neuroprotective effects of rutin on ASH neurons in Caenorhabditis elegans model of Huntington’s disease. Nutr Neurosci 2022;25:2288-301.
24. Cordeiro LM, Soares MV, da Silva AF, et al. Toxicity of copper and zinc alone and in combination in Caenorhabditis elegans model of Huntington’s disease and protective effects of rutin. Neurotoxicology 2023;97:120-32.
25. Zeng Y, Guo W, Xu G, et al. Xyloketal-derived small molecules show protective effect by decreasing mutant Huntingtin protein aggregates in Caenorhabditis elegans model of Huntington’s disease. Drug Des Devel Ther 2016;10:1443-51.
26. Bicca Obetine Baptista F, Arantes LP, Machado ML, et al. Diphenyl diselenide protects a Caenorhabditis elegans model for Huntington’s disease by activation of the antioxidant pathway and a decrease in protein aggregation. Metallomics 2020;12:1142-58.
27. Venken KJ, Simpson JH, Bellen HJ. Genetic manipulation of genes and cells in the nervous system of the fruit fly. Neuron 2011;72:202-30.
28. Pfeiffer BD, Jenett A, Hammonds AS, et al. Tools for neuroanatomy and neurogenetics in Drosophila. Proc Natl Acad Sci U S A 2008;105:9715-20.
29. Ugur B, Chen K, Bellen HJ. Drosophila tools and assays for the study of human diseases. Dis Model Mech 2016;9:235-44.
30. Chan HY, Bonini NM. Drosophila models of human neurodegenerative disease. Cell Death Differ 2000;7:1075-80.
31. Thaker HM, Kankel DR. Mosaic analysis gives an estimate of the extent of genomic involvement in the development of the visual system in Drosophila melanogaster. Genetics 1992;131:883-94.
32. Masse NY, Turner GC, Jefferis GS. Olfactory information processing in Drosophila. Curr Biol 2009;19:R700-13.
33. Amrein H, Thorne N. Gustatory perception and behavior in Drosophila melanogaster. Curr Biol 2005;15:R673-84.
34. Kamikouchi A, Inagaki HK, Effertz T, et al. The neural basis of Drosophila gravity-sensing and hearing. Nature 2009;458:165-71.
35. Song W, Onishi M, Jan LY, Jan YN. Peripheral multidendritic sensory neurons are necessary for rhythmic locomotion behavior in Drosophila larvae. Proc Natl Acad Sci U S A 2007;104:5199-204.
36. McGurk L, Berson A, Bonini NM. Drosophila as an in vivo model for human neurodegenerative disease. Genetics 2015;201:377-402.
37. Yamamoto S, Jaiswal M, Charng WL, et al. A drosophila genetic resource of mutants to study mechanisms underlying human genetic diseases. Cell 2014;159:200-14.
38. Lessing D, Bonini NM. Maintaining the brain: insight into human neurodegeneration from Drosophila melanogaster mutants. Nat Rev Genet 2009;10:359-70.
39. Rosas-Arellano A, Estrada-Mondragón A, Piña R, Mantellero CA, Castro MA. The tiny drosophila melanogaster for the biggest answers in Huntington’s disease. Int J Mol Sci 2018;19:2398.
40. Romero E, Cha GH, Verstreken P, et al. Suppression of neurodegeneration and increased neurotransmission caused by expanded full-length huntingtin accumulating in the cytoplasm. Neuron 2008;57:27-40.
41. Steffan JS, Bodai L, Pallos J, et al. Histone deacetylase inhibitors arrest polyglutamine-dependent neurodegeneration in Drosophila. Nature 2001;413:739-43.
42. Xu F, Kula-Eversole E, Iwanaszko M, Hutchison AL, Dinner A, Allada R. Circadian clocks function in concert with heat shock organizing protein to modulate mutant huntingtin aggregation and toxicity. Cell Rep 2019;27:59-70.e4.
43. Wang CE, Tydlacka S, Orr AL, et al. Accumulation of N-terminal mutant huntingtin in mouse and monkey models implicated as a pathogenic mechanism in Huntington’s disease. Hum Mol Genet 2008;17:2738-51.
44. Chongtham A, Yoo JH, Chin TM, et al. Gut bacteria regulate the pathogenesis of Huntington’s disease in Drosophila model. Front Neurosci 2022;16:902205.
45. Arabit JGJ, Elhaj R, Schriner SE, Sevrioukov EA, Jafari M. Rhodiola rosea improves lifespan, locomotion, and neurodegeneration in a Drosophila melanogaster model of Huntington’s disease. Biomed Res Int 2018;2018:6726874.
47. Bandmann O, Burton EA. Genetic zebrafish models of neurodegenerative diseases. Neurobiol Dis 2010;40:58-65.
48. Karlovich CA, John RM, Ramirez L, Stainier DY, Myers RM. Characterization of the Huntington’s disease (HD) gene homologue in the zebrafish Danio rerio. Gene 1998;217:117-25.
49. Lumsden AL, Henshall TL, Dayan S, Lardelli MT, Richards RI. Huntingtin-deficient zebrafish exhibit defects in iron utilization and development. Hum Mol Genet 2007;16:1905-20.
50. Miller VM, Nelson RF, Gouvion CM, et al. CHIP suppresses polyglutamine aggregation and toxicity in vitro and in vivo. J Neurosci 2005;25:9152-61.
51. VerPlank JJS, Tyrkalska SD, Fleming A, Rubinsztein DC, Goldberg AL. cGMP via PKG activates 26S proteasomes and enhances degradation of proteins, including ones that cause neurodegenerative diseases. Proc Natl Acad Sci U S A 2020;117:14220-30.
52. Lucini C, D’Angelo L, Cacialli P, Palladino A, de Girolamo P. BDNF, Brain, and regeneration: insights from zebrafish. Int J Mol Sci 2018;19:3155.
53. Conforti P, Mas Monteys A, Zuccato C, Buckley NJ, Davidson B, Cattaneo E. In vivo delivery of DN:REST improves transcriptional changes of REST-regulated genes in HD mice. Gene Ther 2013;20:678-85.
54. Yu C, Li CH, Chen S, Yoo H, Qin X, Park H. Decreased BDNF release in cortical neurons of a knock-in mouse model of Huntington’s disease. Sci Rep 2018;8:16976.
55. Schilling G, Becher MW, Sharp AH, et al. Intranuclear inclusions and neuritic aggregates in transgenic mice expressing a mutant N-terminal fragment of Huntingtin. Hum Mol Genet 1999;8:397-407.
56. Rattray I, Smith EJ, Crum WR, et al. Correlations of behavioral deficits with brain pathology assessed through longitudinal MRI and histopathology in the R6/1 mouse model of Huntington’s disease. PLoS One 2013;8:e84726.
57. Mangiarini L, Sathasivam K, Seller M, et al. Exon 1 of the HD gene with an expanded CAG repeat is sufficient to cause a progressive neurological phenotype in transgenic mice. Cell 1996;87:493-506.
58. Carter RJ, Lione LA, Humby T, et al. Characterization of progressive motor deficits in mice transgenic for the human Huntington’s disease mutation. J Neurosci 1999;19:3248-57.
59. Cheng PH, Li CL, Her LS, et al. Significantly differential diffusion of neuropathological aggregates in the brain of transgenic mice carrying N-terminal mutant huntingtin fused with green fluorescent protein. Brain Struct Funct 2013;218:283-94.
60. Gray M, Shirasaki DI, Cepeda C, et al. Full-length human mutant huntingtin with a stable polyglutamine repeat can elicit progressive and selective neuropathogenesis in BACHD mice. J Neurosci 2008;28:6182-95.
61. Kotliarova S, Jana NR, Sakamoto N, et al. Decreased expression of hypothalamic neuropeptides in Huntington disease transgenic mice with expanded polyglutamine-EGFP fluorescent aggregates. J Neurochem 2005;93:641-53.
62. Tebbenkamp AT, Xu G, Siemienski ZB, et al. Experimental mutagenesis of huntingtin to map cleavage sites: different outcomes in cell and mouse models. J Huntingtons Dis 2014;3:73-86.
63. Tebbenkamp AT, Swing D, Tessarollo L, Borchelt DR. Premature death and neurologic abnormalities in transgenic mice expressing a mutant huntingtin exon-2 fragment. Hum Mol Genet 2011;20:1633-42.
64. Stricker-Shaver J, Novati A, Yu-Taeger L, Nguyen HP. Genetic rodent models of huntington disease. Adv Exp Med Biol 2018;1049:29-57.
65. Tebbenkamp AT, Green C, Xu G, et al. Transgenic mice expressing caspase-6-derived N-terminal fragments of mutant huntingtin develop neurologic abnormalities with predominant cytoplasmic inclusion pathology composed largely of a smaller proteolytic derivative. Hum Mol Genet 2011;20:2770-82.
66. Reddy PH, Williams M, Charles V, et al. Behavioural abnormalities and selective neuronal loss in HD transgenic mice expressing mutated full-length HD cDNA. Nat Genet 1998;20:198-202.
67. Slow EJ, van Raamsdonk J, Rogers D, et al. Selective striatal neuronal loss in a YAC128 mouse model of Huntington disease. Hum Mol Genet 2003;12:1555-67.
68. Benn CL, Slow EJ, Farrell LA, et al. Glutamate receptor abnormalities in the YAC128 transgenic mouse model of Huntington’s disease. Neuroscience 2007;147:354-72.
69. Hodgson JG, Agopyan N, Gutekunst CA, et al. A YAC mouse model for Huntington’s disease with full-length mutant huntingtin, cytoplasmic toxicity, and selective striatal neurodegeneration. Neuron 1999;23:181-92.
70. Van Raamsdonk JM, Murphy Z, Slow EJ, Leavitt BR, Hayden MR. Selective degeneration and nuclear localization of mutant huntingtin in the YAC128 mouse model of Huntington disease. Hum Mol Genet 2005;14:3823-35.
71. Van Raamsdonk JM, Pearson J, Slow EJ, Hossain SM, Leavitt BR, Hayden MR. Cognitive dysfunction precedes neuropathology and motor abnormalities in the YAC128 mouse model of Huntington’s disease. J Neurosci 2005;25:4169-80.
72. Kennedy L, Shelbourne PF, Dewar D. Alterations in dopamine and benzodiazepine receptor binding precede overt neuronal pathology in mice modelling early Huntington disease pathogenesis. Brain Res 2005;1039:14-21.
73. Shelbourne PF, Killeen N, Hevner RF, et al. A Huntington’s disease CAG expansion at the murine Hdh locus is unstable and associated with behavioural abnormalities in mice. Hum Mol Genet 1999;8:763-74.
74. Menalled LB, Sison JD, Dragatsis I, Zeitlin S, Chesselet MF. Time course of early motor and neuropathological anomalies in a knock-in mouse model of Huntington’s disease with 140 CAG repeats. J Comp Neurol 2003;465:11-26.
75. Wheeler VC, Gutekunst CA, Vrbanac V, et al. Early phenotypes that presage late-onset neurodegenerative disease allow testing of modifiers in Hdh CAG knock-in mice. Hum Mol Genet 2002;11:633-40.
76. Wheeler VC, White JK, Gutekunst CA, et al. Long glutamine tracts cause nuclear localization of a novel form of huntingtin in medium spiny striatal neurons in HdhQ92 and HdhQ111 knock-in mice. Hum Mol Genet 2000;9:503-13.
77. Heng MY, Tallaksen-Greene SJ, Detloff PJ, Albin RL. Longitudinal evaluation of the Hdh(CAG)150 knock-in murine model of Huntington’s disease. J Neurosci 2007;27:8989-98.
78. Tallaksen-Greene SJ, Crouse AB, Hunter JM, Detloff PJ, Albin RL. Neuronal intranuclear inclusions and neuropil aggregates in HdhCAG(150) knockin mice. Neuroscience 2005;131:843-52.
79. Lin CH, Tallaksen-Greene S, Chien WM, et al. Neurological abnormalities in a knock-in mouse model of Huntington’s disease. Hum Mol Genet 2001;10:137-44.
80. Levine MS, Cepeda C, Hickey MA, Fleming SM, Chesselet MF. Genetic mouse models of Huntington’s and Parkinson’s diseases: illuminating but imperfect. Trends Neurosci 2004;27:691-7.
81. Yu ZX, Li SH, Evans J, Pillarisetti A, Li H, Li XJ. Mutant huntingtin causes context-dependent neurodegeneration in mice with Huntington’s disease. J Neurosci 2003;23:2193-202.
82. Gardian G, Browne SE, Choi DK, et al. Neuroprotective effects of phenylbutyrate in the N171-82Q transgenic mouse model of Huntington’s disease. J Biol Chem 2005;280:556-63.
83. Kong G, Cao KL, Judd LM, Li S, Renoir T, Hannan AJ. Microbiome profiling reveals gut dysbiosis in a transgenic mouse model of Huntington’s disease. Neurobiol Dis 2020;135:104268.
84. Tong X, Ao Y, Faas GC, et al. Astrocyte Kir4.1 ion channel deficits contribute to neuronal dysfunction in Huntington’s disease model mice. Nat Neurosci 2014;17:694-703.
85. Abjean L, Ben Haim L, Riquelme-Perez M, et al. Reactive astrocytes promote proteostasis in Huntington’s disease through the JAK2-STAT3 pathway. Brain 2023;146:149-66.
86. Ona VO, Li M, Vonsattel JP, et al. Inhibition of caspase-1 slows disease progression in a mouse model of Huntington’s disease. Nature 1999;399:263-7.
87. van Dellen A, Blakemore C, Deacon R, York D, Hannan AJ. Delaying the onset of Huntington’s in mice. Nature 2000;404:721-2.
88. Ferrante RJ, Andreassen OA, Jenkins BG, et al. Neuroprotective effects of creatine in a transgenic mouse model of Huntington’s disease. J Neurosci 2000;20:4389-97.
89. Willner P. Methods for assessing the validity of animal models of human psychopathology. In: Boulton AA, Baker GB, Martin-Iverson MT, editors. Animal models in psychiatry, I. Neuromethods, vol 18. Humana Press; 1991.
90. Jacobsen JC, Bawden CS, Rudiger SR, et al. An ovine transgenic Huntington’s disease model. Hum Mol Genet 2010;19:1873-82.
91. Huntington’s Disease Sheep Collaborative Research Group, Reid SJ, Patassini S, Handley RR, et al. Further molecular characterisation of the OVT73 transgenic sheep model of Huntington’s disease identifies cortical aggregates. J Huntingtons Dis 2013;2:279-95.
92. Morton AJ, Rudiger SR, Wood NI, et al. Early and progressive circadian abnormalities in Huntington’s disease sheep are unmasked by social environment. Hum Mol Genet 2014;23:3375-83.
93. Yang SH, Cheng PH, Banta H, et al. Towards a transgenic model of Huntington’s disease in a non-human primate. Nature 2008;453:921-4.
94. Snyder BR, Chan AWS. Progress in developing transgenic monkey model for Huntington’s disease. J Neural Transm 2018;125:401-17.
95. Chan AW, Jiang J, Chen Y, et al. Progressive cognitive deficit, motor impairment and striatal pathology in a transgenic Huntington disease monkey model from infancy to adulthood. PLoS One 2015;10:e0122335.
96. Yan S, Li S, Li XJ. Use of large animal models to investigate Huntington’s diseases. Cell Regen 2019;8:9-11.
97. Coe CL, Lubach GR. Maternal determinants of gestation length in the rhesus monkey. Trends Dev Biol 2021;14:63-72.
98. Tian CY. China is facing serious experimental monkey shortage during the COVID-19 lockdown. J Med Primatol 2021;50:225-7.
99. Yang D, Wang CE, Zhao B, et al. Expression of Huntington’s disease protein results in apoptotic neurons in the brains of cloned transgenic pigs. Hum Mol Genet 2010;19:3983-94.
100. Yan S, Tu Z, Liu Z, et al. A huntingtin knockin pig model recapitulates features of selective neurodegeneration in Huntington’s disease. Cell 2018;173:989-1002.e13.
101. Brignull HR, Moore FE, Tang SJ, Morimoto RI. Polyglutamine proteins at the pathogenic threshold display neuron-specific aggregation in a pan-neuronal Caenorhabditis elegans model. J Neurosci 2006;26:7597-606.
102. Nagai Y, Fujikake N, Ohno K, et al. Prevention of polyglutamine oligomerization and neurodegeneration by the peptide inhibitor QBP1 in Drosophila. Hum Mol Genet 2003;12:1253-9.
103. Marsh JL, Walker H, Theisen H, et al. Expanded polyglutamine peptides alone are intrinsically cytotoxic and cause neurodegeneration in Drosophila. Hum Mol Genet 2000;9:13-25.
104. Le Bourg E, Lints FA. Hypergravity and aging in Drosophila melanogaster. 4. Climbing activity: 4. Climbing Activity. Gerontology 1992;38:59-64.
105. Chongtham A, Agrawal N. Curcumin modulates cell death and is protective in Huntington’s disease model. Sci Rep 2016;6:18736.
106. Ganetzky B, Flanagan JR. On the relationship between senescence and age-related changes in two wild-type strains of Drosophila melanogaster. Exp Gerontol 1978;13:189-96.
107. Jackson GR, Salecker I, Dong X, et al. Polyglutamine-expanded human huntingtin transgenes induce degeneration of Drosophila photoreceptor neurons. Neuron 1998;21:633-42.
108. Brand AH, Perrimon N. Targeted gene expression as a means of altering cell fates and generating dominant phenotypes. Development 1993;118:401-15.
109. Muqit MM, Feany MB. Modelling neurodegenerative diseases in Drosophila: a fruitful approach? Nat Rev Neurosci 2002;3:237-43.
110. Tomasiewicz HG, Flaherty DB, Soria JP, Wood JG. Transgenic zebrafish model of neurodegeneration. J Neurosci Res 2002;70:734-45.
Cite This Article

How to Cite
Download Citation
Export Citation File:
Type of Import
Tips on Downloading Citation
Citation Manager File Format
Type of Import
Direct Import: When the Direct Import option is selected (the default state), a dialogue box will give you the option to Save or Open the downloaded citation data. Choosing Open will either launch your citation manager or give you a choice of applications with which to use the metadata. The Save option saves the file locally for later use.
Indirect Import: When the Indirect Import option is selected, the metadata is displayed and may be copied and pasted as needed.
About This Article
Special Issue
Copyright
Data & Comments
Data
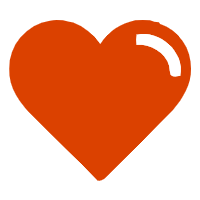
Comments
Comments must be written in English. Spam, offensive content, impersonation, and private information will not be permitted. If any comment is reported and identified as inappropriate content by OAE staff, the comment will be removed without notice. If you have any queries or need any help, please contact us at [email protected].