Age-related energetic reprogramming in glial cells: possible correlations with Parkinson’s disease
Abstract
Glial cells populate the central nervous system and undertake indispensable roles in safeguarding and maintaining optimal neuronal performance. Throughout life, the brain undergoes inevitable changes that impact both neurons and glial cells. Concurrent with age-related neurodegenerative diseases, such as Alzheimer’s disease (AD) and Parkinson’s disease (PD), metabolic dysfunctions in glial cells are consistently observed. Though widely debated, the idea of treating neurodegenerative disorders by manipulating brain bioenergetics warrants further exploration. This review discusses the distinctive metabolic characteristics of central nervous system (CNS) glia, the metabolic deviations that occur in glial cells in the aging brain, and the ramifications of metabolic rewiring within glia on neurodegenerative disorders, specifically PD. We focus on astrocytes and microglia due to their substantial transformations under aging and diseased states, known as reactivation. Special attention is given to clarifying the complex relationships between dysregulated glial energy metabolism and brain disorders. By discussing both classic theories and current advances in this field, we aim to shed light on promising therapeutic horizons anchored in the strategic calibration of glial metabolic configurations.
Keywords
INTRODUCTION
The human brain represents one of the most intricately structured tissues in complex organisms, playing a fundamental role in cognition and behavior[1]. With advancing age, both the brain’s architecture and its microenvironment undergo transformations, culminating in a gradual decline in cognitive capacities and behavioral functions, including learning, memory, and motor coordination[2]. Neuronal degenerative diseases, characterized by neuronal loss and disruptions in network connections, are frequently associated with aging and exhibit shared underlying mechanisms[3].
Despite comprising just 2% of overall body mass, the human brain demands an estimated 20%-25% of the body’s entire energy expenditure, making it one of the most energetically costly organs within the human body[4]. In general, glucose serves as the principal energy substrate supporting brain function. Once across the blood-brain barrier, glucose undergoes transformation into adenosine triphosphate (ATP), a vital energy currency, via dual pathways - namely glycolysis and oxidative phosphorylation (OXPHOS)[2]. Notably, diverse cell types within the central nervous system exhibit markedly dissimilar energy metabolism profiles. Specifically, neurons primarily rely upon OXPHOS for generating ATP[5], whereas astrocytes predominantly employ glycolysis[6]. Moreover, neural cells possess the capacity to harness various substrates such as glutamate, glutamine, pyruvate, and lactate, thereby facilitating intricate intercellular exchange mechanisms among glial and neuronal populations[7,8]. Such complex interactions play pivotal roles in preserving physiological equilibrium and contributing to disease etiology. Another crucial aspect meriting careful attention pertains to the dialogue between neuronal dynamics and regulatory mechanisms governing brain energy balance. Mounting evidence reveals that fluctuations in metabolic rates correspond to disparate cognitive and behavioral modes - quiescent vs. activated states[9]. During synchronized neuronal operations, augmented demand for, as well as consumption of, oxygen and glucose ensues, collectively termed neurovascular and neurometabolic coupling. Integral players mediating this symphony of metabolic equilibrium maintenance are none other than glial cells, especially astrocytes, which occupy key positions in this complex regulatory network[10].
There is a growing body of research bolstering the hypothesis that disruptions in energy metabolism play a central role in the onset and advancement of neurological disorders. The present understanding concerning disrupted regional energy expenditure tied to neurodegenerative disorders is consolidated in Table 1[11-16]. Regional biochemical adaptations imply the significant impact of locally transformed microenvironments accompanying neurodegeneration[2]. Acting as a focal participant birthed from manifold metabolic avenues, reactive oxygen species (ROS) meaningfully add to this detrimental local environment. ROS have been identified as increasingly prevalent in the mammalian central nervous system during aging[17]. Mitochondria are among the primary sources of ROS, generating it through oxidative phosphorylation. Normally, ROS participates in physiological functions like inflammation and synaptic plasticity[18]. Nevertheless, an excess production of ROS within cells can trigger oxidative stress, disrupting redox homeostasis and resulting in mitochondrial dysfunction. This chain reaction could potentially exacerbate age-related neurological disorders, including Alzheimer’s disease (AD) and Parkinson’s disease (PD), as supported by animal research findings[17,19]. Studies have reported significant reductions in ATP synthesis capacity, pyruvate dehydrogenase activity, and calcium buffering abilities in aged animals compared to their younger counterparts[20]. Recent investigations also emphasize the impact of mitochondrial damage induced by ROS on several neurodegenerative illnesses, underscoring the critical significance of energetic malfunctions[21]. Accompanying diminished ATP generation, the amassed ROS foster a markedly proinflammatory setting. Within the aged brain, neurons endure prolonged exposure to this persistent inflammatory atmosphere, conceivably heightening neuronal loss and synaptic abnormalities, thus facilitating the evolution of the senescent brain toward pathological manifestations.
The brain regions associated with abnormal glucose metabolism in neurodegenerative diseases
Disorders | Metabolic change | Related brain area | Ref. |
AD | Glucose hypometabolism | Posterior cingulate cortex; precuneus; anterior hippocampus; parahippocampal gyrus | [11,12] |
PD | Glucose hypometabolism | Premotor; parieto-occipital cortex | [13] |
Glucose hypermetabolism | Cerebellum; pons; paracentral gyrus; lentiform nucleus | ||
HD | Glucose hypometabolism | Frontal lobe; temporal lobe; striatum | [14] |
ALS | Glucose hypometabolism | Prefrontal; cingulate cortex; caudate nuclei; rolandic operculum; thalamus | [15,16] |
Glucose hypermetabolism | Amygdalae; midbrain; pons; cerebellum |
Considering the substantial involvement of glial cells in shaping and managing the central nervous system’s microenvironment, it is logical to posit that the energy metabolic condition of these cells plays an essential role in the shift from health to disease. Indeed, reactivated astrocytes have emerged as a prominent source of ROS; despite primarily utilizing glycolysis for ATP production, they generate considerable quantities of mitochondrial ROS[22]. These accumulating astrocyte-derived ROS have downstream consequences on neuronal activity and animal behavior[23]. Additionally, it has been extensively established that astrocytes govern cerebral blood flow by fine-tuning the diameter of microvasculature, consequently modulating the delivery of both oxygen and glucose. In terms of other glial subtypes, microglia - given its intimate connection to the central nervous system’s inflammatory responses - have shown close ties between metabolic reprogramming and polarization[24]. Glial cells not only construct the microenvironment but are themselves influenced by variations in the metabolic landscape; their functional capabilities and survivability remain subject to these modifications[6]. For instance, the aged brain faces challenges related to the insufficient provision of energy substrates due to compromised local microcirculation, which can fundamentally transform glial cell metabolism, eventually inflicting detriment upon the integrated neuronal circuitry. Nevertheless, the ramifications of modified glial energy metabolism on brain aging and attendant diseases remain an active area of rigorous investigation.
In this review, we will provide a concise overview of established concepts while delving into recent advancements on three key topics: (1) The distinctive characteristics of glial energy metabolism in the central nervous system; (2) The metabolic alterations occurring in glial cells within the aging brain; (3) The impact of metabolic reprogramming in glial cells on neurodegeneration, particularly in PD. Our aim is to offer deeper insights into the association between dysfunction in glial energy metabolism and brain disorders, thereby illuminating the potential for novel therapeutic strategies centered around modulating glial metabolic states.
GLIA ENERGETICS AND THE NORMAL FUNCTION OF THE CENTER NERVE SYSTEM
In recent decades, there has been increasing focus on the study of glial energy metabolism, considering the distinct morphologies and functions of the three major subtypes of glial cells: astrocytes, microglia, and oligodendrocytes. While some similarities exist in their bioenergetic characteristics, each subtype serves different neuronal functions. For instance, both astrocytes and oligodendrocytes favor glycolysis and provide bioenergetic support to neurons[6,25]. However, oligodendrocytes primarily facilitate the propagation of action potentials and maintain a close relationship with axonal mitochondria[26], whereas the energy demands of synaptic transmission are more closely associated with astrocytes[27]. Undeniably, the harmonious bioenergetic collaboration between various glial cells and neurons forms an integral pillar upholding regular brain functions in humans [Figure 1].
While it has been reported that oligodendrocyte dysfunction and demyelination may contribute to neuronal death in neurodegenerative diseases[28], particularly AD[29], our current understanding of oligodendrocyte metabolic dysfunctions and their impact on the degeneration of the nigrostriatal pathway remains limited. Therefore, our discussion will primarily focus on astrocytes and microglia, examining their metabolic dysregulations in aging and degenerative brain conditions. We anticipate that future studies will provide deeper insights into oligodendrocyte metabolism and its role in neurodegeneration.
In physiological conditions, distinct metabolic profiles characterize different subtypes of brain cells to facilitate their respective functions. Neurons predominantly utilize mitochondrial oxidative phosphorylation, whereas astrocytes predominantly engage in aerobic glycolysis. Additionally, astrocytes metabolize glucose into lactate, which serves as a crucial metabolic substrate for neurons. Oligodendrocytes primarily rely on glycolysis, and the energy metabolism of microglial cells undergoes reprogramming in response to their specific states.
Normal bioenergetic characteristics of astrocytes
Comprising the majority of glial cells constituting the brain, astrocytes fulfill indispensable metabolic obligations benefitting neighboring neurons. Astrocytes possess distinctive cellular structures and occupy strategic positions that enable them to perceive environmental cues and adapt to associated fluctuations[6]. Positioned within the neurovascular unit, astrocytes consistently mediate the interface between neurons and capillaries. Their end feet establish connections with both blood vessels and synapses, fostering intricate interactions among these integral components[30]. Recently, Nippert et al. proposed that astrocytes can facilitate the increase in cerebral blood flow triggered by hypoglycemia via a calcium-dependent mechanism involving the release of vasodilators[31]. Their study employed insulin-treated mice to simulate hypoglycemia conditions. Under hypoglycemic conditions, arterioles undergo dilation, concomitant with heightened astrocytic Ca2+ signaling. Application of specific inhibitors targeting the production of astrocyte-derived vasodilators, such as prostaglandin and epoxyeicosatrienoic acids, resulted in substantial reductions of arterial dilation by 89% and 76%, respectively[31]. This observation suggests a potential mechanism by which astrocytes modulate cerebral blood flow to facilitate glucose transport from blood vessels to astrocytes, thereby generating metabolic substrates crucial for supporting neuronal activity.
Current research indicates that under normal circumstances, astrocytes primarily utilize glucose for energy production. Glucose is taken up into the cell via glucose transporter (GLUTs) proteins, particularly GLUT1, and is phosphorylated by hexokinase (HK) to form glucose-6-phosphate[32]. This glucose can then enter various metabolic pathways, including glycolysis, the pentose phosphate pathway (PPP), OXPHOS, and glycogen synthesis[33]. However, astrocytes predominantly metabolize glucose through aerobic glycolysis, which not only meets their lower energy demands but also produces lactate, a metabolic substrate crucial for neuronal support. As early as 1994, Pellerin et al. proposed the astrocyte-neuron lactate shuttle hypothesis[34]. The process through which lactate shuttles between neurons and astrocytes is as follows: following neuronal stimulation, adjacent astrocytes can uptake excess glutamate at the synapse. Subsequently, the entry of sodium into astrocytes activates Na+/K+ ATPase, the activation of which consumes ATP produced by glycolysis. Lactate produced by glycolysis is transported extracellularly by monocarboxylate transporters (MCT1 and MCT4), and then transported into neurons via MCT2 on the neuronal membrane, providing oxidative phosphorylation substrates for neurons[35,36]. Roumes et al. developed a rat model where they specifically downregulated cortical neuronal MCT2 or astrocytic MCT4. They then measured metabolic and hemodynamic responses to sensory stimulation using functional magnetic resonance spectroscopy and blood oxygen level-dependent (BOLD) functional magnetic resonance imaging (fMRI)[37]. Their results convey that the shuttle of lactate between astrocytes and neurons is indispensable for neuro-metabolic and neurovascular coupling. Furthermore, recent research by Muraleedharan et al. found that adenosine monophosphate-activated protein kinase (AMPK) is a key enzyme regulating the production of lactate by astrocytes and its transfer to neurons[38]. They generated AMPK-KO astrocytes in vitro and cultured them in a medium containing [U-13C] glucose. The results showed that AMPK-KO astrocytes exhibited a 50% reduction in lactate production, along with significantly decreased glucose uptake and membrane translocation of the glucose transporter GLUT1. However, the addition of lactate or wild-type astrocytes to the culture medium restored these functions. In animal experiments, the researchers found that the lactate content in the brains of AMPK knockout mice on postnatal day 28 decreased by 40% as measured by 1H magnetic resonance spectroscopy. Additionally, cortical thinning and neuronal loss were observed in these mice[38]. These findings underscore the importance of AMPK-regulated glycolytic metabolism in astrocytes for maintaining neuronal homeostasis.
Metabolic processes of lipids are intricately linked to energy production in astrocytes[39]. While neurons have limited lipid metabolism capabilities, astrocytes exhibit robust lipid metabolism enzyme activity, necessitating a high energy supply[40]. Astrocytes synthesize cholesterol in the brain and transport it via ApoE-containing lipoproteins[41]. Neurons absorb lipids derived from astrocytes to support synaptic formation and function[42]. When neurons become hyperactive, they produce peroxidized lipids, raising questions about how neurons manage lipid accumulation. Recent research has shed light on this process: hyperactive neurons generate toxic fatty acids (FAs), which are transferred to astrocytes via ApoE-positive lipid droplets. Astrocytes then metabolize FAs through mitochondrial β-oxidation and initiate detoxification gene expression programs[43]. This result highlights the dynamic interplay between neurons and astrocytes in maintaining brain lipid homeostasis. Hence, it becomes entirely justifiable to propose that impairment in astrocytic energy metabolism would likely compromise neuronal function and viability, given its adverse implications on lipid processing.
Microglia has distinct metabolic patterns in normal brain
Microglia serve as the central nervous system’s resident macrophages, maintaining homeostasis throughout brain development and adulthood[44]. They exhibit high plasticity, enabling them to adapt to diverse environmental cues through extensive phenotypic remodeling while surveilling the brain milieu[45]. This adaptability is closely linked to their ability to utilize various energy substrates, such as glucose, lipids, ketone bodies, lactate, and pyruvate[46,47]. Unlike astrocytes, microglia primarily rely on oxidative phosphorylation for ATP production using glucose[48]. FAs undergo ATP production by coupling with peroxisomes to generate acetyl-CoA, which then enters the tricarboxylic acid (TCA) cycle[49]. In their activated state, however, macrophages display diverse metabolic phenotypes. When stimulated by lipopolysaccharide (LPS), proinflammatory macrophages demonstrate heightened glycolytic metabolism alongside compromised mitochondrial oxidative phosphorylation[50,51]. Conversely, anti-inflammatory macrophages induced by interleukin 4 (IL-4) exhibit elevated mitochondrial oxidative phosphorylation[52]. The metabolic alterations of both astrocytes and microglia are depicted in Figure 2.
Astrocytes and microglia exist in two distinct states: resting and activated. In the resting state, astrocytes predominantly rely on glycolysis for energy production. However, upon activation, they primarily depend on OXPHOS. Microglia, on the other hand, exhibit a different metabolic profile. In their resting state, microglia primarily utilize OXPHOS to generate ATP from glucose. However, upon activation, their metabolic patterns vary depending on their physiological functions. Anti-inflammatory microglia show an increase in mitochondrial oxidative phosphorylation. Conversely, in proinflammatory microglia, there is an upregulation of glycolytic metabolism, while mitochondrial oxidative phosphorylation is impaired. Proinflammatory microglia are characterized by a rounded morphology, indicative of their hyperactive state, whereas anti-inflammatory microglia exhibit an elongated morphology.
Microglia possess crucial properties such as phagocytosis and inflammation. They play a significant role in synaptic pruning through phagocytosis, which fosters synapse formation and development, while also maintaining a normal neuronal count in the developing brain[53]. In pathological conditions like pathogen infections or acute ischemic strokes, microglia release cytokines and chemokines, such as interleukin-1 beta (IL-1β) and chemokine (C-X-C motif) ligand 1 (CXCL1), to safeguard the central nervous system by recruiting neutrophils[54,55]. A growing body of evidence suggests that altering the metabolism of microglia directly influences their phagocytic and inflammatory responses. In terms of inflammation, microglia can detect changes in brain metabolism and adjust their own metabolic processes to regulate neuronal activity. For instance, fluctuations in blood glucose levels have been linked to the inflammatory activation of microglia and an increased secretion of proinflammatory factors[56]. It is noteworthy that recent investigations utilizing single-cell RNA sequencing and spatial transcriptomic analysis have provided new insights into microglial subtypes. The traditional classification of microglia into proinflammatory (M1) and anti-inflammatory (M2) subtypes is now considered oversimplified[57]. More complex subtypes of microglia have been identified under specific conditions associated with distinct neurodegenerative disorders[57]. The extent to which metabolic plasticity contributes to the divergence of reactive microglia remains to be further studied.
Microglia not only possess metabolic plasticity but also engage in metabolic interactions with neurons. Triggering receptor expressed on myeloid cells 2 (Trem2), a myeloid cell-specific gene expressed in brain microglia, has been shown to regulate neuronal development by influencing the metabolic fitness of neurons in a region-specific manner[58]. Additionally, microglia may monitor and protect neurons by sensing changes in mitochondrial energy at the microglia-neuron somatic junction[59]. Impairment in mitochondrial dynamics is associated with pathological processes in neurodegenerative diseases like AD and PD[60]. Microglial metabolic plasticity also responds to metabolic changes in the brain and body. Glial-neuronal interactions form a circuit that senses internal signals and adjusts behavior and metabolism according to metabolic states[61]. For instance, obesity, an energy metabolism disorder, induces brain inflammation and activates microglia, contributing to cognitive impairment by aberrantly phagocytosing synaptic spines. However, the precise mechanisms underlying microglial activation during obesity remain incompletely understood[62].
ALTERED CELLULAR ENERGY METABOLISM IN THE AGING BRAIN
As humans age, notable structural remodeling occurs within the brain, a phenomenon readily detectable through computed tomography and magnetic resonance imaging modalities[63,64]. Prominent examples include the observable decreasing trends observed in both gray matter volume and white matter volume over time. A previous investigation revealed a significant reduction in the overall gray matter volume of the brain, with particularly pronounced atrophy noted in the frontal and temporal lobes[65]. This decrease in gray matter volume is closely linked to the deterioration of cognitive functions. For instance, the atrophy of gray matter in the prefrontal lobe correlates with a decline in executive function[66]. In contrast to gray matter, the alterations in white matter during the aging process exhibit distinct patterns. The integrity of white matter structure undergoes dynamic changes with age, showing a gradual increase before age 40, peaking around 50 years, and subsequently declining after 60 years[67]. Furthermore, the age-related deterioration in cognitive functions is closely linked to alterations in white matter structural integrity, which are implicated in neurodegenerative conditions. Subclinical mild cognitive impairment may elevate the susceptibility to developing such diseases because of these changes[68]. The alterations in brain structures can be attributed primarily to neuronal cell loss and impaired glial function. Given the critical functions of energy metabolism in maintaining central nervous system homeostasis, it is unsurprising that close connections exist between macroscale structural alterations in the brain and microscale functional modifications to cellular metabolic reprogramming[69-71].
Metabolic reprogramming of aging neuron
Progressing age engenders disruptions in neuronal energy metabolism, crystallized along two primary dimensions: (1) anomalous glucose usage patterns; and (2) compromised mitochondrial oxidative phosphorylation[72,73]. Age-induced insulin resistance, accompanied by deficient glucose transportation, materializes within neuronal communities, yielding substantially curtailed glucose uptake. According to a longitudinal population-based investigation, the incidence of insulin resistance correlates adversely with escalating cognitive deterioration throughout the aging journey[72]. Yarchoan et al. uncovered analogous outcomes after analyzing postmortem brain samples sourced from geriatric subjects, wherein elevated levels of serine 312 phosphorylated insulin receptor substrate 1 (IRS-1) were noted, suggesting enhanced insulin resistance[74]. This was paralleled by reduced neuronal glucose assimilation capacities. Over and above external determinants (e.g., hormonal imbalance), internally driven elements, specifically dysregulated glucose ingress attributable to lost GLUT3 transporters, encumber optimal neuronal metabolic efficiency as years accrue[75]. Thus, during aging, declines in both extrinsic (endocrine irregularities) and intrinsic components (neuronal nutrient absorption) potentiate diminished neuronal glucose metabolic efficiencies.
On the other hand, neuronal energy provision primarily relies on mitochondrial oxidative phosphorylation, underscoring the pivotal role of mitochondria in maintaining neuronal energy balance. Mitochondria are distributed throughout both axons and dendrites, serving to generate ATP for sustaining electrochemical neurotransmission, cellular upkeep, and repair processes, crucially supporting the heightened energy demands of axonal activity[76,77]. Nonetheless, aging renders axons particularly vulnerable to its effects, affecting energy metabolism through various mechanisms[78]. Firstly, with aging, there are discernible changes in both the quantity and morphology of mitochondria within axons. Vagnoni et al. observed a significant decrease in mitochondrial transport within the axons of fruit fly wing neurons during aging[73]. In advanced mice, the volume and length of individual mitochondria within the axons of the optic nerve notably increase[79]. Secondly, aging contributes to heightened hyperexcitability in cortical networks, consequently escalating the energy requirements for axonal propagation of action potentials and synaptic neurotransmitter release[80,81]. Furthermore, it is imperative to recognize that the elongation of axons reduces energy availability at their distal ends, a factor of significance in long-distance projections like the nigrostriatal pathway, implicated in PD.
Bioenergetic shifts of glial cells in aging brain
As previously discussed, the maintenance of normal neuronal activity relies on the efficient metabolic coordination between neurons and glial cells, with particular emphasis on the metabolic support provided by astrocytes. However, recent research indicates that aging is associated with a decline in glycolysis and an augmentation of mitochondrial oxidative metabolism in astrocytes[82,83]. Utilizing magnetic resonance spectroscopy to investigate mitochondrial metabolism in both neurons and astrocytes, a recent study revealed that, compared to younger individuals, the elderly population demonstrates diminished neuronal mitochondrial metabolism but heightened astrocytic mitochondrial metabolism within the brain[84]. The age-related metabolic transition observed in astrocytes could potentially jeopardize lactate production, consequently restricting the fuel supply available for neuronal OXPHOS. It is noteworthy that alterations in the intrinsic state of astrocytes, characterized by their transition into reactive astrocytes, induce changes in their energy metabolism profile[85]. In studies conducted on rodent models, researchers isolated primary cortical astrocytes from rats aged 7, 13, and 18 months. They observed age-associated shifts in the metabolic pathways of these cells, transitioning from glycolysis to mitochondrial oxidative phosphorylation, accompanied by elevated levels of reactive astrocytes [Figure 2][86]. These results suggest that the increased mitochondrial oxidative phosphorylation may promote a functional shift in astrocytes from a neuro-supportive state to a neurotoxic state. However, recent research has presented contrasting findings. McNair et al., employing stable isotope tracing in vitro, observed a significant reduction in astrocytic TCA cycle activity and oxidative phosphorylation metabolism in hippocampal slices of aged mice. Conversely, glycolytic activity and glutamate uptake exhibited a notable increase[87]. These findings suggest a hypothesis that aging could potentially impact the metabolic energy pathways of distinct subpopulations of astrocytes differently across various brain regions[88].
Among all brain cells, astrocytes serve as the primary sites for FA oxidation, and with brain aging, there is an observed increase in the utilization rate of free FAs[40]. Research by Boisvert et al. revealed a significant reduction in the expression of cholesterol synthesis genes in astrocytes of aged mouse brains compared to adult mice, indicating a shift in lipid metabolism in these cells[39]. Subsequent studies have demonstrated that during aging, astrocytes not only experience a decline in glycolytic capacity, impeding the effective provision of necessary energy substrates for neurons, but also exhibit an increase in FA oxidation, resulting in the production of acetyl-CoA, thereby further modulating mitochondrial metabolic functions[83]. This mechanism may contribute to the transition of astrocyte function from a resting to a reactive state.
Age-dependent metabolic reprogramming also affects microglial cells. Research by Mela et al. revealed that in the brains of elderly animals, microglial cells exhibit significantly elevated glycolysis levels and adopt a proinflammatory phenotype[89]. This underscores that the glucose metabolism activity of microglial cells is not only influenced by intrinsic changes but also undergoes metabolic reprogramming with advancing age. Evidently, the regulatory mechanism involving proinflammatory prostaglandin E2 (PGE2) signaling stands out as a potential candidate controlling the age-associated shift in microglial energy metabolism. Mechanistically, engagement between PGE2 and its cognate EP2 receptor prompts glucose routing into glycogen reserves, leading to activation of the protein kinase B (AKT)-glycogen synthase kinase 3 beta (GSK3β)-glycogen synthase 1 (GYS1) cascade; this eventuality suppresses incoming glucose fluxes destined for mitochondrial OXPHOS, thereby indirectly reducing ATP yields. Quite remarkably, amplified PGE2 signaling triggers direct reconfiguration of aged microglia, shifting them away from customary anti-inflammatory tendencies toward embracing a decidedly proinflammatory phenotype instead[90].
Furthermore, in addition to glucose metabolism, aging also impacts the lipid metabolism of microglial cells. Recent investigations have identified a notable increase in apolipoprotein E (APOE) mRNA expression within isolated microglial cells from aged mice[91,92]. Dysregulation of APOE has been linked to the formation of lipid droplets. Victor et al. demonstrated that microglial cells derived from induced pluripotent stem cells of individuals carrying APOE risk variants display abundant lipid droplets (LD)[93]. Transcriptomic analysis of lipid droplet-associated microglia unveiled severely compromised phagocytic activity in microglial cells, accompanied by heightened production of ROS and inflammatory cytokines, exhibiting characteristics partially mirroring those of lipopolysaccharide-treated mouse microglia[94]. Recent studies have also demonstrated that lipid-laden microglia in the aged brain exhibit exacerbated innate immune responses to stroke[95]. However, further investigation is required to elucidate the crosstalk between altered lipid metabolism and shifts in energy production patterns in aged microglia.
DYSREGULATED GLIAL ENERGY METABOLISM IN PD
PD represents the second most common neurodegenerative disorder worldwide, characterized by the selective loss of dopaminergic (DA) neurons in the midbrain. Despite extensive associations drawn between PD-linked DA neuronal death and α-synuclein, along with several influential factors, the precise molecular machinery of such lethal neurodegeneration remains elusive. Unquestionably, given the exceedingly high energy demands imposed upon the nigrostriatal system, it seems plausible that aging-associated energy failures might critically contribute to PD-associated neurotoxicity. Indubitably, numerous lines of evidence point convincingly toward pervasive bioenergetic defects embedded within the PD pathophysiology.
Dysfunctions of energy metabolism in dopaminergic neurons
Advanced age serves as a recognized risk factor for developing PD. Even in the absence of PD, the aging brain experiences gradual depletion of DA neurons located in the substantia nigra zona compacta (SNc), reaching approximately 30%-40%[96]. However, once afflicted with PD, the extent of DA neuronal loss in the SNc often surpasses 80%[97]. Morphologically speaking, residual healthy DA neurons display signs of hypertrophy during typical aging, whereas those affected by PD demonstrate marked atrophy[98]. Further exploration conducted by researchers such as Elstner et al. aimed to distinguish any discrepancies in gene expression changes exhibited by dopaminergic neurons between aging and PD cases. Utilizing laser capture microdissection, they extracted DA neurons from the SNc of PD patients and non-PD controls, followed by comparative expression profiling and subsequent pathway analyses. Findings indicated that PD-impacted DA neurons expressed genes strongly associated with mitochondrial dysfunction and profound energy metabolic distress[99]. Additional parallel observations made by Keeney et al. highlighted comparable perturbations in the SNc of PD brains, whereby impairment of electron transport chain (ETC) Complex I activity resulted in overwhelmingly increased production of ROS[100] and severe hindrances to proper ATP synthesis[101,102]. Collectively, these discoveries highlight the striking correlation existing between DA neuronal mortality in the SNc and underlying metabolic disturbances.
The higher energy demands of DA neurons can be attributed to their unique physiological characteristics[103]. Recent perspectives suggest that the activation of SNc DA neurons relies on a specific type of L-type voltage-gated calcium ion channel, facilitating calcium ion efflux that is ATP-dependent[104]. Moreover, SNc DA neuron axons are relatively large, primarily relying on oxidative phosphorylation for energy supply, resulting in higher levels of ROS at their axon terminals[103]. Additionally, SNc DA neurons exhibit another two distinct properties, which make them more vulnerable to bioenergetic dysregulation than other neurons: (1) Dopamine can produce the derivative tetrahydroisoquinoline (TIQ), which, in combination with methylglyoxal - a significant byproduct of glucose metabolism - forms 1-acetyl-6,7-dihydroxy-1,2,3,4-tetrahydroisoquinoline (ADTIQ), contributing to the degeneration of DA neurons[105]; (2) They contain elevated levels of iron, which can exacerbate oxidative stress, triggering the conversion of α-synuclein from an α-helix to a β-sheet conformation. This transformation promotes α-synuclein aggregation, thereby increasing the pathological risk of PD[106]. Growing evidence suggests that metabolic abnormalities in PD may contribute to disease progression by promoting α-synuclein aggregation. Hyperglycemia has been shown to influence the aggregation of α-synuclein, potentially impairing neuronal glycolytic capacity[107]. Metabolic dysfunctions in PD patients involve not only impaired glucose metabolism but also abnormalities in lipid metabolism. Altered lipid metabolism is closely associated with α-synuclein as well. For instance, oleic acid can induce the formation of α-synuclein aggregates in human neuronal cells[108]. A recent study identified a class of lipid molecules, specifically lysophosphatidylcholine (LPC), that selectively recognize and stabilize the monomeric form of α-synuclein. This research highlights LPC’s critical role in maintaining the natural conformation of α-synuclein and preventing its pathological aggregation, thus linking lipid metabolism disturbances to α-synuclein pathology in PD[109].
Although research evidence from patient studies suggests a close association between disrupted energy metabolism and PD, it remains uncertain whether bioenergetic dysfunction precedes or results from neuronal degeneration. Data from PD animal models may shed light on this inquiry. We summarized metabolic disruptions observed in several chemically induced and genetically engineered models in
The energy metabolism alternations in different PD models
PD model | Gene/compound | Metabolic change | Ref. |
Chemical models | MPP+ | Mitochondrial respiration↓ Glycolysis↑ | [110] |
Rotenone | Pentose phosphate pathway↑ Glycolysis↓ Mitochondrial respiration↓ | [112] | |
6-OHDA | Glycolysis↑ | [113,114] | |
Paraquat | Pentose phosphate pathway↑ Glycolysis↓ Mitochondrial respiration↓ | [114] | |
Genetic models | α-Synuclein | Lipid metabolism alteration Mitochondrial respiration↓ | [111,115,116] |
Parkin; DJ-1; LRRK2; PINK1 | Glycolysis↑ Mitochondrial respiration↓ | [117-120] |
Bioenergetic disruptions of glial cells in PD brain
Investigations centered on both patient populations and relevant genomic animal models reveal that many PD-linked mutated genes indeed find expression within astrocytes, influencing their energy metabolic landscapes accordingly[32]. Table 3 provides a condensed overview detailing the range of astrocytic energy metabolic traits germane to familiar PD pathogenic gene variants[121-127]. Remarkably, divergent pathogenic genes exhibit varying degrees of disruption to astrocytic energy homeostasis, with mutant α-synuclein playing a significant role in bioenergetic deregulation. Once internalized by astrocytes, mutant α-synuclein impairs lipid catabolism by reducing the metabolic turnover rates of arachidonic acid and palmitic acid, thereby skewing cellular energy balance[128,129]. Furthermore, α-synuclein intervention can impede the proper localization of aquaporin-4, undermining efficient glutamate uptake and leading to abnormal glutamate metabolism[124].
The energy metabolism characteristics of astrocytes associated with various PD mutations
Mutated genes | Characteristics of metabolic perturbations | Ref. |
PARK7 | Glutamate uptake↓ Lipid raft assembly↓ Mitochondrial function↓ | [121,122] |
SNCA | Glutamate uptake↓ TAG↑ Lipid raft assembly↓ | [123,124] |
PINK1 | ATP production↓ Oxygen consumption↓ ROS↑ Lipid raft assembly↓ | [125] |
PARK2 | Glycolysis↓ Lipid raft assembly↓ | [126] |
LRRK2 | Glycolysis↓ OXPHOS↓ Lipid raft assembly↓ | [127] |
Recent research conducted by Sonninen et al. has revealed that in PD, alterations in astrocytic metabolism primarily stem from changes in mitochondrial function[127]. Utilizing induced pluripotent stem cells (iPSCs) derived from PD patients and subsequent differentiation into astrocytes, they observed notable shifts. Specifically, both maximal and spare respiratory capacities of astrocytes were significantly diminished, accompanied by a marked attenuation in glycolysis. Additionally, analysis of mitochondrial DNA (mtDNA) indicated lower levels in astrocytes from PD patients. These findings were corroborated by Russ et al., who exposed human astrocytes to α-synuclein fibers, demonstrating a significant reduction in mitochondrial respiration within astrocytes[130]. Under conditions of aging, there is a decrease in glycolysis and an increase in oxidative phosphorylation within astrocytes. Conversely, in PD, both glycolysis and oxidative phosphorylation significantly decrease due to mitochondrial damage and reduced glucose uptake. Consequently, we propose a hypothesis: under normal circumstances, astrocytes predominantly rely on glycolysis for energy production, but as the organism ages and encounters heightened inflammation and oxidative stress, they transition toward mitochondrial oxidative phosphorylation to meet energy demands. This metabolic shift serves multiple purposes, including sustaining astrocytic energy requirements, facilitating the conversion of toxic substances like excitatory glutamate, and bolstering antioxidant capacity such as glutathione synthesis. However, in PD, dysfunctional mitochondrial metabolism in astrocytes compromises energy support, antioxidant function, and detoxification processes. Therefore, disruptions in mitochondrial metabolism may act as a pivotal “switch” in the metabolic reprogramming of astrocytes in PD. It is noteworthy that there are two subtypes of astrocytes in the substantia nigra pars compacta, namely protoplasmic astrocytes and fibrous astrocytes. However, studies suggest that only protoplasmic astrocytes contribute to the accumulation of mutated α-synuclein[131].
The energy metabolic reprogramming in the PD brain not only contributes to the demise of DA neurons but may also play a role in the emergence of non-motor symptoms. Our recent research indicates that there is a repression in mitochondrial transcription and function in cortical astrocytes of PD brain, coinciding with the activation of the hypoxia-inducible factor (HIF) pathway. This disruption in energy production within cortical astrocytes could worsen the dysfunction of the neuronal network responsible for mood regulation, potentially triggering the onset of depression associated with PD[132]. Previous studies have often focused on the switch from glycolysis to OXPHOS in disease-related astrocytes. However, our results underscore the importance of mitochondrial energy production and its role in regulating key gene expressions. Interestingly, our study also reveals distinct response patterns of cortical astrocytes to PD-associated neurodegeneration compared to midbrain astrocytes, potentially due to differences in energy metabolic signaling. Although the underlying mechanisms require further investigation, our findings emphasize the importance of studying the region-specific bioenergetics of astrocytes in PD and other neurodegenerative diseases[132].
The molecular regulators of metabolic reprogramming, which are crucial for developing novel therapeutic strategies, however, remain largely unknown. One of the key regulatory pathways is the WNT/β-catenin signaling pathway, implicated in many neurodegenerative diseases[133]. Vallée et al. investigated the thermodynamic implications of metabolic reprogramming in PD, discovering a downregulation of WNT/
The excessive activation of microglial cells plays a pivotal role in the progression of PD. Activated microglial cells are previously categorized into M1 and M2 phenotypes. These phenotypes exhibit distinct metabolic patterns in response to various environmental stimuli, enabling them to fulfill their respective functions[136]. In the PD brain, microglia are activated by several factors, including damage-associated molecular patterns (DAMPs) released by dying neurons, proinflammatory factors from astrocytes, and extracellular α-synuclein oligomers[137]. These stimuli promote the activation of M1 microglial cells, triggering neuroinflammation and leading to the loss of dopaminergic neurons[138]. In a recent study, Lavisse et al. directly observed activated microglial cells and elevated levels of proinflammatory factors in the brains of PD patients using
The metabolic reprogramming of microglial cells entails intricate modulation involving multiple signaling pathways and cytokines. Firstly, in vitro experiments have revealed a significant elevation in the mRNA levels of PKM2, glyceraldehyde 3-phosphate dehydrogenase (GAPDH), and GLUT1, alongside increased lactate production, following α-synuclein treatment of microglial cells[142]. This highlights that there are changes in the glucose metabolism of activated microglial cells. Secondly, upon activation of microglial cells, hypoxia-inducible factor 1 alpha (HIF-1α) stimulates the targets of rapamycin (mTOR), leading to the upregulation of glycolytic enzymes[143,144]. Previous studies have also found that in a mouse model of PD, microglia induce metabolic reshaping (transition from oxidative phosphorylation to aerobic glycolysis) through the mTOR/HIF-1α pathway, thereby exacerbating the pathophysiological phenotype of PD[145]. Consequently, the mTOR/HIF-1α pathway emerges as a promising targeted regulatory axis for modulating these metabolic alterations. Notably, the mTOR signaling pathway exerts a multifaceted regulatory role encompassing glucose, lipid, and other metabolic pathways, thereby influencing mitochondrial function and dynamics[146]. This suggests that metabolic reprogramming of microglial cells in the context of PD may involve multiple metabolic processes.
In addition to glucose metabolism, there is a growing focus on changes in the lipid metabolism of microglia in PD. Researchers have observed lipid accumulation in microglial cells in both animal models and PD patients, which, along with microglial cell damage, contributes to neuroinflammation and disruptions in neural circuits[147]. This suggests a potential pathogenic mechanism of microglial cells in PD progression. However, understanding of the mechanisms underlying microglial cell lipid metabolism and PD progression remains limited. Hence, further research is necessary to elucidate the metabolic characteristics of microglial cells in PD, facilitating more effective treatment and prevention strategies.
Metabolic analysis of human tissues and biological fluids reveals the complex interactions among individual genetics, proteins, and environmental factors[3]. We are optimistic about using metabolite analysis to monitor PD progression. Specifically, lipid metabolism-related products may serve as biomarkers for diagnosing PD. Current research indicates that α-synuclein is linked to FA uptake, cholesterol metabolism, and phospholipid activity. Recent population cohort studies have investigated lipid metabolism patterns in PD patients. For example, Choe et al. performed lipid profiling in 294 PD patients and 588 healthy individuals, finding that PD patients had lower levels of high-density lipoprotein cholesterol but higher levels of lipoprotein A compared to controls[148]. Additionally, Dahabiyeh et al. discovered that PD patients had higher serum levels of saturated lysophosphatidylcholine, unsaturated triglycerides, and hydroxyeicosatetraenoic acid, while levels of neuroamide, sphingomyelin, and phosphatidylserine were lower[149]. These findings suggest that lipid imbalances and dysfunctions in lipid pathways are significant biomarkers for PD diagnosis.
CONCLUSION
In conclusion, the accumulating evidence highlights the importance of dysregulated energy metabolism in glial cells in relation to aging and neurodegeneration. Nonetheless, substantial strides are necessary to devise novel therapeutic approaches for neurodegenerative ailments, including PD, through the manipulation of glial bioenergetics. Numerous pivotal inquiries remain unresolved and await comprehensive elucidation. Firstly, initial investigations on elderly individuals and patients primarily focus on observing correlations rather than establishing causation. Unraveling the metabolic alterations that either result from or lead to neuronal death poses a significant challenge. The substantial disparities between rodent models and human physiology limit the ability of animal studies to comprehensively address this issue. Innovative imaging techniques that integrate PET, computed tomography (CT), and magnetic resonance imaging (MRI) show promise in potentially unlocking insights into this complex problem. Secondly, the intricate molecular mechanisms governing the transition between different metabolic patterns in glial cells remain largely elusive. While the HIF-1α is a recognized key regulator, it fails to account for all observations, particularly the shift from glycolysis to mitochondrial oxidative phosphorylation in reactive astrocytes. Unraveling the regulatory network that underlies energy metabolic reprogramming is crucial for identifying novel druggable targets. Last but not least, the investigation into the interplay among diverse metabolic pathways in disease-associated glial cells is imperative, given that many patients with neurodegenerative disorders also present with metabolic dysregulations like hyperlipidemia and hyperglycemia. Ideally, a comprehensive patient management approach for neurodegenerative diseases should be devised to address metabolic disorders while safeguarding vulnerable neurons.
DECLARATIONS
Authors’ contributions
Literature search, writing, and original draft preparation: Chu B, Xiang H, Wang H, Lin Y, Li R
Conceptualization, review, revision, and editing: Qian H, Hu J
Availability of data and materials
Not applicable.
Financial support and sponsorship
This study was supported by the National Natural Science Foundation of China (82271276 to Qian H; 12102086 to Hu J) and Sichuan Provincial Science and Technology Department (Sichuan Provincial Science and Technology Project, Key R&D Program, 2022YFS0599 to Qian H).
Conflicts of interest
All authors declared that there are no conflicts of interest.
Ethical approval and consent to participate
Not applicable.
Consent for publication
Not applicable.
Copyright
© The Author(s) 2024.
REFERENCES
2. Mattson MP, Arumugam TV. Hallmarks of brain aging: adaptive and pathological modification by metabolic states. Cell Metab 2018;27:1176-99.
3. Emamzadeh FN, Surguchov A. Parkinson’s disease: biomarkers, treatment, and risk factors. Front Neurosci 2018;12:612.
5. Zheng X, Boyer L, Jin M, et al. Metabolic reprogramming during neuronal differentiation from aerobic glycolysis to neuronal oxidative phosphorylation. Elife 2016;5:e13374.
6. Bélanger M, Allaman I, Magistretti PJ. Brain energy metabolism: focus on astrocyte-neuron metabolic cooperation. Cell Metab 2011;14:724-38.
7. Sá JV, Kleiderman S, Brito C, et al. Quantification of metabolic rearrangements during neural stem cells differentiation into astrocytes by metabolic flux analysis. Neurochem Res 2017;42:244-53.
8. Weightman Potter PG, Vlachaki Walker JM, Robb JL, et al. Basal fatty acid oxidation increases after recurrent low glucose in human primary astrocytes. Diabetologia 2019;62:187-98.
9. Mann K, Deny S, Ganguli S, Clandinin TR. Coupling of activity, metabolism and behaviour across the Drosophila brain. Nature 2021;593:244-8.
10. Beard E, Lengacher S, Dias S, Magistretti PJ, Finsterwald C. Astrocytes as key regulators of brain energy metabolism: new therapeutic perspectives. Front Physiol 2021;12:825816.
11. Yakushev I, Schreckenberger M, Müller MJ, et al. Functional implications of hippocampal degeneration in early Alzheimer’s disease: a combined DTI and PET study. Eur J Nucl Med Mol Imaging 2011;38:2219-27.
12. Bateman RJ, Xiong C, Benzinger TLS, et al; Dominantly Inherited Alzheimer Network. Clinical and biomarker changes in dominantly inherited Alzheimer’s disease. N Engl J Med 2012;367:795-804.
13. Matthews DC, Lerman H, Lukic A, et al. FDG PET Parkinson’s disease-related pattern as a biomarker for clinical trials in early stage disease. Neuroimage Clin 2018;20:572-9.
14. Ciarmiello A, Cannella M, Lastoria S, et al. Brain white-matter volume loss and glucose hypometabolism precede the clinical symptoms of Huntington’s disease. J Nucl Med 2006;47:215-22.
15. Cistaro A, Valentini MC, Chiò A, et al. Brain hypermetabolism in amyotrophic lateral sclerosis: a FDG PET study in ALS of spinal and bulbar onset. Eur J Nucl Med Mol Imaging 2012;39:251-9.
16. Diehl-Schmid J, Licata A, Goldhardt O, et al; FTLDc Study Group. FDG-PET underscores the key role of the thalamus in frontotemporal lobar degeneration caused by C9ORF72 mutations. Transl Psychiatry 2019;9:54.
17. Ionescu-Tucker A, Cotman CW. Emerging roles of oxidative stress in brain aging and Alzheimer’s disease. Neurobiol Aging 2021;107:86-95.
18. Kishida KT, Klann E. Sources and targets of reactive oxygen species in synaptic plasticity and memory. Antioxid Redox Signal 2007;9:233-44.
19. Trist BG, Hare DJ, Double KL. Oxidative stress in the aging substantia nigra and the etiology of Parkinson’s disease. Aging Cell 2019;18:e13031.
20. Pandya JD, Grondin R, Yonutas HM, et al. Decreased mitochondrial bioenergetics and calcium buffering capacity in the basal ganglia correlates with motor deficits in a nonhuman primate model of aging. Neurobiol Aging 2015;36:1903-13.
21. Alqahtani T, Deore SL, Kide AA, et al. Mitochondrial dysfunction and oxidative stress in Alzheimer’s disease, and Parkinson’s disease, Huntington’s disease and Amyotrophic Lateral Sclerosis - an updated review. Mitochondrion 2023;71:83-92.
22. Murray TE, Richards CM, Robert-Gostlin VN, Bernath AK, Lindhout IA, Klegeris A. Potential neurotoxic activity of diverse molecules released by astrocytes. Brain Res Bull 2022;189:80-101.
23. Vicente-Gutierrez C, Bonora N, Bobo-Jimenez V, et al. Astrocytic mitochondrial ROS modulate brain metabolism and mouse behaviour. Nat Metab 2019;1:201-11.
24. Miao J, Chen L, Pan X, Li L, Zhao B, Lan J. Microglial metabolic reprogramming: emerging insights and therapeutic strategies in neurodegenerative diseases. Cell Mol Neurobiol 2023;43:3191-210.
25. Traxler L, Lagerwall J, Eichhorner S, Stefanoni D, D’Alessandro A, Mertens J. Metabolism navigates neural cell fate in development, aging and neurodegeneration. Dis Model Mech 2021;14:dmm048993.
26. Chamberlain KA, Sheng ZH. Mechanisms for the maintenance and regulation of axonal energy supply. J Neurosci Res 2019;97:897-913.
27. Shan L, Zhang T, Fan K, Cai W, Liu H. Astrocyte-neuron signaling in synaptogenesis. Front Cell Dev Biol 2021;9:680301.
28. Yang K, Wu Z, Long J, et al. White matter changes in Parkinson’s disease. NPJ Parkinsons Dis 2023;9:150.
29. Depp C, Sun T, Sasmita AO, et al. Myelin dysfunction drives amyloid-β deposition in models of Alzheimer’s disease. Nature 2023;618:349-57.
30. Takahashi S. Metabolic contribution and cerebral blood flow regulation by astrocytes in the neurovascular unit. Cells 2022;11:813.
31. Nippert AR, Chiang PP, Del Franco AP, Newman EA. Astrocyte regulation of cerebral blood flow during hypoglycemia. J Cereb Blood Flow Metab 2022;42:1534-46.
32. Chen Z, Yuan Z, Yang S, et al. Brain energy metabolism: astrocytes in neurodegenerative diseases. CNS Neurosci Ther 2023;29:24-36.
33. Briski KP, Ibrahim MMH, Mahmood ASMH, Alshamrani AA. Norepinephrine regulation of ventromedial hypothalamic nucleus astrocyte glycogen metabolism. Int J Mol Sci 2021;22:759.
34. Pellerin L, Magistretti PJ. Glutamate uptake into astrocytes stimulates aerobic glycolysis: a mechanism coupling neuronal activity to glucose utilization. Proc Natl Acad Sci U S A 1994;91:10625-9.
35. Benarroch EE. Neuron-astrocyte interactions: partnership for normal function and disease in the central nervous system. Mayo Clin Proc 2005;80:1326-38.
36. Cortes-Campos C, Elizondo R, Carril C, et al. MCT2 expression and lactate influx in anorexigenic and orexigenic neurons of the arcuate nucleus. PLoS One 2013;8:e62532.
37. Roumes H, Jollé C, Blanc J, et al. Lactate transporters in the rat barrel cortex sustain whisker-dependent BOLD fMRI signal and behavioral performance. Proc Natl Acad Sci U S A 2021;118:e2112466118.
38. Muraleedharan R, Gawali MV, Tiwari D, et al. AMPK-regulated astrocytic lactate shuttle plays a non-cell-autonomous role in neuronal survival. Cell Rep 2020;32:108092.
39. Boisvert MM, Erikson GA, Shokhirev MN, Allen NJ. The aging astrocyte transcriptome from multiple regions of the mouse brain. Cell Rep 2018;22:269-85.
40. Ebert D, Haller RG, Walton ME. Energy contribution of octanoate to intact rat brain metabolism measured by 13C nuclear magnetic resonance spectroscopy. J Neurosci 2003;23:5928-35.
41. Cashikar AG, Toral-Rios D, Timm D, et al. Regulation of astrocyte lipid metabolism and ApoE secretionby the microglial oxysterol, 25-hydroxycholesterol. J Lipid Res 2023;64:100350.
42. van Deijk AF, Camargo N, Timmerman J, et al. Astrocyte lipid metabolism is critical for synapse development and function in vivo. Glia 2017;65:670-82.
43. Ioannou MS, Jackson J, Sheu SH, et al. Neuron-astrocyte metabolic coupling protects against activity-induced fatty acid toxicity. Cell 2019;177:1522-35.e14.
44. Wright-Jin EC, Gutmann DH. Microglia as dynamic cellular mediators of brain function. Trends Mol Med 2019;25:967-79.
45. Bernier LP, York EM, Kamyabi A, Choi HB, Weilinger NL, MacVicar BA. Microglial metabolic flexibility supports immune surveillance of the brain parenchyma. Nat Commun 2020;11:1559.
46. Nagy AM, Fekete R, Horvath G, et al. Versatility of microglial bioenergetic machinery under starving conditions. Biochim Biophys Acta Bioenerg 2018;1859:201-14.
47. Sabogal-Guáqueta AM, Marmolejo-Garza A, Trombetta-Lima M, et al. Species-specific metabolic reprogramming in human and mouse microglia during inflammatory pathway induction. Nat Commun 2023;14:6454.
48. Dienel GA. Brain glucose metabolism: integration of energetics with function. Physiol Rev 2019;99:949-1045.
49. Tawbeh A, Gondcaille C, Trompier D, Savary S. Peroxisomal ABC transporters: an update. Int J Mol Sci 2021;22:6093.
50. Huang SC, Smith AM, Everts B, et al. Metabolic reprogramming mediated by the mTORC2-IRF4 signaling axis is essential for macrophage alternative activation. Immunity 2016;45:817-30.
51. Van den Bossche J, O’Neill LA, Menon D. Macrophage immunometabolism: where are we (going)? Trends Immunol 2017;38:395-406.
52. Vats D, Mukundan L, Odegaard JI, et al. Oxidative metabolism and PGC-1β attenuate macrophage-mediated inflammation. Cell Metab 2006;4:13-24.
53. Paolicelli RC, Bolasco G, Pagani F, et al. Synaptic pruning by microglia is necessary for normal brain development. Science 2011;333:1456-8.
54. Drummond RA, Swamydas M, Oikonomou V, et al. CARD9+ microglia promote antifungal immunity via IL-1β- and CXCL1-mediated neutrophil recruitment. Nat Immunol 2019;20:559-70.
55. Huang X, Guo M, Zhang Y, et al. Microglial IL-1RA ameliorates brain injury after ischemic stroke by inhibiting astrocytic CXCL1-mediated neutrophil recruitment and microvessel occlusion. Glia 2023;71:1607-25.
56. Hsieh CF, Liu CK, Lee CT, Yu LE, Wang JY. Acute glucose fluctuation impacts microglial activity, leading to inflammatory activation or self-degradation. Sci Rep 2019;9:840.
57. Paolicelli RC, Sierra A, Stevens B, et al. Microglia states and nomenclature: a field at its crossroads. Neuron 2022;110:3458-83.
58. Tagliatti E, Desiato G, Mancinelli S, et al. Trem2 expression in microglia is required to maintain normal neuronal bioenergetics during development. Immunity 2024;57:86-105.e9.
59. Cserép C, Pósfai B, Lénárt N, et al. Microglia monitor and protect neuronal function through specialized somatic purinergic junctions. Science 2020;367:528-37.
60. Joshi AU, Mochly-Rosen D. Mortal engines: mitochondrial bioenergetics and dysfunction in neurodegenerative diseases. Pharmacol Res 2018;138:2-15.
61. García-Cáceres C, Balland E, Prevot V, et al. Role of astrocytes, microglia, and tanycytes in brain control of systemic metabolism. Nat Neurosci 2019;22:7-14.
62. Henn RE, Guo K, Elzinga SE, et al. Single-cell RNA sequencing identifies hippocampal microglial dysregulation in diet-induced obesity. iScience 2023;26:106164.
63. Resnick SM, Pham DL, Kraut MA, Zonderman AB, Davatzikos C. Longitudinal magnetic resonance imaging studies of older adults: a shrinking brain. J Neurosci 2003;23:3295-301.
64. Driscoll I, Davatzikos C, An Y, et al. Longitudinal pattern of regional brain volume change differentiates normal aging from MCI. Neurology 2009;72:1906-13.
65. Fjell AM, McEvoy L, Holland D, Dale AM, Walhovd KB; Alzheimer’s Disease Neuroimaging Initiative. What is normal in normal aging? Effects of aging, amyloid and Alzheimer’s disease on the cerebral cortex and the hippocampus. Prog Neurobiol 2014;117:20-40.
66. Manard M, Bahri MA, Salmon E, Collette F. Relationship between grey matter integrity and executive abilities in aging. Brain Res 2016;1642:562-80.
67. Liu H, Wang L, Geng Z, et al. A voxel-based morphometric study of age- and sex-related changes in white matter volume in the normal aging brain. Neuropsychiatr Dis Treat 2016;12:453-65.
68. Kwak K, Giovanello KS, Bozoki A, Styner M, Dayan E; Alzheimer’s Disease Neuroimaging Initiative. Subtyping of mild cognitive impairment using a deep learning model based on brain atrophy patterns. Cell Rep Med 2021;2:100467.
69. Dienel GA, Rothman DL. Reevaluation of astrocyte-neuron energy metabolism with astrocyte volume fraction correction: impact on cellular glucose oxidation rates, glutamate-glutamine cycle energetics, glycogen levels and utilization rates vs. exercising muscle, and Na+/K+ pumping rates. Neurochem Res 2020;45:2607-30.
70. Boley N, Patil S, Garnett EO, et al. Association between gray matter volume variations and energy utilization in the brain: implications for developmental stuttering. J Speech Lang Hear Res 2021;64:2317-24.
71. Raiko JRH, Tuulari JJ, Saari T, et al. Associations between brain gray matter volumes and adipose tissue metabolism in healthy adults. Obesity 2021;29:543-9.
72. Thambisetty M, Beason-Held LL, An Y, et al. Impaired glucose tolerance in midlife and longitudinal changes in brain function during aging. Neurobiol Aging 2013;34:2271-6.
73. Vagnoni A, Hoffmann PC, Bullock SL. Reducing Lissencephaly-1 levels augments mitochondrial transport and has a protective effect in adult Drosophila neurons. J Cell Sci 2016;129:178-90.
74. Yarchoan M, Toledo JB, Lee EB, et al. Abnormal serine phosphorylation of insulin receptor substrate 1 is associated with tau pathology in Alzheimer’s disease and tauopathies. Acta Neuropathol 2014;128:679-89.
75. Mattson MP. Roles of the lipid peroxidation product 4-hydroxynonenal in obesity, the metabolic syndrome, and associated vascular and neurodegenerative disorders. Exp Gerontol 2009;44:625-33.
76. Delgado T, Petralia RS, Freeman DW, et al. Comparing 3D ultrastructure of presynaptic and postsynaptic mitochondria. Biol Open 2019;8:bio044834.
77. Faitg J, Lacefield C, Davey T, et al. 3D neuronal mitochondrial morphology in axons, dendrites, and somata of the aging mouse hippocampus. Cell Rep 2021;36:109509.
78. Salvadores N, Sanhueza M, Manque P, Court FA. Axonal degeneration during aging and its functional role in neurodegenerative disorders. Front Neurosci 2017;11:451.
79. Stahon KE, Bastian C, Griffith S, Kidd GJ, Brunet S, Baltan S. Age-related changes in axonal and mitochondrial ultrastructure and function in white matter. J Neurosci 2016;36:9990-10001.
80. Stargardt A, Swaab DF, Bossers K. Storm before the quiet: neuronal hyperactivity and Aβ in the presymptomatic stages of Alzheimer’s disease. Neurobiol Aging 2015;36:1-11.
81. Lockwood CT, Duffy CJ. Hyperexcitability in aging is lost in Alzheimer’s: what is all the excitement about? Cereb Cortex 2020;30:5874-84.
82. Goyal MS, Vlassenko AG, Blazey TM, et al. Loss of brain aerobic glycolysis in normal human aging. Cell Metab 2017;26:353-60.e3.
83. Cotto B, Natarajaseenivasan K, Langford D. Astrocyte activation and altered metabolism in normal aging, age-related CNS diseases, and HAND. J Neurovirol 2019;25:722-33.
84. Boumezbeur F, Mason GF, de Graaf RA, et al. Altered brain mitochondrial metabolism in healthy aging as assessed by in vivo magnetic resonance spectroscopy. J Cereb Blood Flow Metab 2010;30:211-21.
85. Clarke LE, Liddelow SA, Chakraborty C, Münch AE, Heiman M, Barres BA. Normal aging induces A1-like astrocyte reactivity. Proc Natl Acad Sci U S A 2018;115:E1896-905.
86. Jiang T, Cadenas E. Astrocytic metabolic and inflammatory changes as a function of age. Aging Cell 2014;13:1059-67.
87. McNair LM, Andersen JV, Waagepetersen HS. Stable isotope tracing reveals disturbed cellular energy and glutamate metabolism in hippocampal slices of aged male mice. Neurochem Int 2023;171:105626.
88. de Ceglia R, Ledonne A, Litvin DG, et al. Specialized astrocytes mediate glutamatergic gliotransmission in the CNS. Nature 2023;622:120-9.
89. Mela V, Mota BC, Milner M, et al. Exercise-induced re-programming of age-related metabolic changes in microglia is accompanied by a reduction in senescent cells. Brain Behav Immun 2020;87:413-28.
90. Minhas PS, Latif-Hernandez A, McReynolds MR, et al. Restoring metabolism of myeloid cells reverses cognitive decline in ageing. Nature 2021;590:122-8.
91. Hickman SE, Kingery ND, Ohsumi TK, et al. The microglial sensome revealed by direct RNA sequencing. Nat Neurosci 2013;16:1896-905.
92. Lee S, Devanney NA, Golden LR, et al. APOE modulates microglial immunometabolism in response to age, amyloid pathology, and inflammatory challenge. Cell Rep 2023;42:112196.
93. Victor MB, Leary N, Luna X, et al. Lipid accumulation induced by APOE4 impairs microglial surveillance of neuronal-network activity. Cell Stem Cell 2022;29:1197-212.e8.
94. Marschallinger J, Iram T, Zardeneta M, et al. Lipid-droplet-accumulating microglia represent a dysfunctional and proinflammatory state in the aging brain. Nat Neurosci 2020;23:194-208.
95. Yen JJ, Yu II. The role of ApoE-mediated microglial lipid metabolism in brain aging and disease. Immunometabolism 2023;5:e00018.
96. Ross GW, Petrovitch H, Abbott RD, et al. Parkinsonian signs and substantia nigra neuron density in decendents elders without PD. Ann Neurol 2004;56:532-9.
97. Vanitallie TB. Parkinson disease: primacy of age as a risk factor for mitochondrial dysfunction. Metabolism 2008;57 Suppl 2:S50-5.
98. Rudow G, O’Brien R, Savonenko AV, et al. Morphometry of the human substantia nigra in ageing and Parkinson’s disease. Acta Neuropathol 2008;115:461-70.
99. Elstner M, Morris CM, Heim K, et al. Expression analysis of dopaminergic neurons in Parkinson’s disease and aging links transcriptional dysregulation of energy metabolism to cell death. Acta Neuropathol 2011;122:75-86.
100. Keeney PM, Xie J, Capaldi RA, Bennett JP Jr. Parkinson’s disease brain mitochondrial complex I has oxidatively damaged subunits and is functionally impaired and misassembled. J Neurosci 2006;26:5256-64.
101. Sherer TB, Betarbet R, Testa CM, et al. Mechanism of toxicity in rotenone models of Parkinson’s disease. J Neurosci 2003;23:10756-64.
102. Requejo-Aguilar R, Lopez-Fabuel I, Fernandez E, Martins LM, Almeida A, Bolaños JP. PINK1 deficiency sustains cell proliferation by reprogramming glucose metabolism through HIF1. Nat Commun 2014;5:4514.
103. Pissadaki EK, Bolam JP. The energy cost of action potential propagation in dopamine neurons: clues to susceptibility in Parkinson’s disease. Front Comput Neurosci 2013;7:13.
104. Guzman JN, Sanchez-Padilla J, Wokosin D, et al. Oxidant stress evoked by pacemaking in dopaminergic neurons is attenuated by DJ-1. Nature 2010;468:696-700.
105. Xie B, Lin F, Ullah K, et al. A newly discovered neurotoxin ADTIQ associated with hyperglycemia and Parkinson’s disease. Biochem Biophys Res Commun 2015;459:361-6.
106. Chinta SJ, Andersen JK. Redox imbalance in Parkinson’s disease. Biochim Biophys Acta 2008;1780:1362-7.
107. Huang M, Lou D, Charli A, et al. Mitochondrial dysfunction-induced H3K27 hyperacetylation perturbs enhancers in Parkinson’s disease. JCI Insight 2021;6:e138088.
108. Fanning S, Haque A, Imberdis T, et al. Lipidomic analysis of α-synuclein neurotoxicity identifies stearoyl CoA desaturase as a target for parkinson treatment. Mol Cell 2019;73:1001-14.e8.
109. Zhao C, Tu J, Wang C, et al. Lysophosphatidylcholine binds α-synuclein and prevents its pathological aggregation. Natl Sci Rev 2024;11:nwae182.
110. Mazzio E, Soliman KF. The role of glycolysis and gluconeogenesis in the cytoprotection of neuroblastoma cells against 1-methyl 4-phenylpyridinium ion toxicity. Neurotoxicology 2003;24:137-47.
111. Fernandes HJR, Patikas N, Foskolou S, et al. Single-cell transcriptomics of Parkinson’s disease human in vitro models reveals dopamine neuron-specific stress responses. Cell Rep 2020;33:108263.
112. Powers R, Lei S, Anandhan A, et al. Metabolic investigations of the molecular mechanisms associated with Parkinson’s disease. Metabolites 2017;7:22.
113. Giordano S, Lee J, Darley-Usmar VM, Zhang J. Distinct effects of rotenone, 1-methyl-4-phenylpyridinium and 6-hydroxydopamine on cellular bioenergetics and cell death. PLoS One 2012;7:e44610.
114. Lei S, Zavala-Flores L, Garcia-Garcia A, et al. Alterations in energy/redox metabolism induced by mitochondrial and environmental toxins: a specific role for glucose-6-phosphate-dehydrogenase and the pentose phosphate pathway in paraquat toxicity. ACS Chem Biol 2014;9:2032-48.
115. Butler EK, Voigt A, Lutz AK, et al. The mitochondrial chaperone protein TRAP1 mitigates α-synuclein toxicity. PLoS Genet 2012;8:e1002488.
116. Rothman SM, Griffioen KJ, Fishbein KW, et al. Metabolic abnormalities and hypoleptinemia in α-synuclein A53T mutant mice. Neurobiol Aging 2014;35:1153-61.
117. Müftüoglu M, Elibol B, Dalmizrak O, et al. Mitochondrial complex I and IV activities in leukocytes from patients with parkin mutations. Mov Disord 2004;19:544-8.
118. Wang HL, Chou AH, Wu AS, et al. PARK6 PINK1 mutants are defective in maintaining mitochondrial membrane potential and inhibiting ROS formation of substantia nigra dopaminergic neurons. Biochim Biophys Acta 2011;1812:674-84.
119. Niu J, Yu M, Wang C, Xu Z. Leucine-rich repeat kinase 2 disturbs mitochondrial dynamics via dynamin-like protein. J Neurochem 2012;122:650-8.
120. Requejo-Aguilar R, Lopez-Fabuel I, Jimenez-Blasco D, Fernandez E, Almeida A, Bolaños JP. DJ1 represses glycolysis and cell proliferation by transcriptionally up-regulating Pink1. Biochem J 2015;467:303-10.
121. Larsen NJ, Ambrosi G, Mullett SJ, Berman SB, Hinkle DA. DJ-1 knock-down impairs astrocyte mitochondrial function. Neuroscience 2011;196:251-64.
122. Kim JM, Cha SH, Choi YR, Jou I, Joe EH, Park SM. DJ-1 deficiency impairs glutamate uptake into astrocytes via the regulation of flotillin-1 and caveolin-1 expression. Sci Rep 2016;6:28823.
123. Cole NB, Murphy DD, Grider T, Rueter S, Brasaemle D, Nussbaum RL. Lipid droplet binding and oligomerization properties of the Parkinson’s disease protein alpha-synuclein. J Biol Chem 2002;277:6344-52.
124. Kim S, Pajarillo E, Nyarko-Danquah I, Aschner M, Lee E. Role of astrocytes in Parkinson’s disease associated with genetic mutations and neurotoxicants. Cells 2023;12:622.
125. Choi I, Kim J, Jeong HK, et al. PINK1 deficiency attenuates astrocyte proliferation through mitochondrial dysfunction, reduced AKT and increased p38 MAPK activation, and downregulation of EGFR. Glia 2013;61:800-12.
126. Cha SH, Choi YR, Heo CH, et al. Loss of parkin promotes lipid rafts-dependent endocytosis through accumulating caveolin-1: implications for Parkinson’s disease. Mol Neurodegener 2015;10:63.
127. Sonninen TM, Hämäläinen RH, Koskuvi M, et al. Metabolic alterations in Parkinson’s disease astrocytes. Sci Rep 2020;10:14474.
128. Castagnet PI, Golovko MY, Barceló-Coblijn GC, Nussbaum RL, Murphy EJ. Fatty acid incorporation is decreased in astrocytes cultured from alpha-synuclein gene-ablated mice. J Neurochem 2005;94:839-49.
129. Alecu I, Bennett SAL. Dysregulated lipid metabolism and its role in α-synucleinopathy in Parkinson’s disease. Front Neurosci 2019;13:328.
130. Russ K, Teku G, Bousset L, et al. TNF-α and α-synuclein fibrils differently regulate human astrocyte immune reactivity and impair mitochondrial respiration. Cell Rep 2021;34:108895.
131. Halliday GM, Stevens CH. Glia: initiators and progressors of pathology in Parkinson’s disease. Mov Disord 2011;26:6-17.
132. Peng Y, He J, Xiang H, et al. Potential impact of hypoxic astrocytes on the aggravation of depressive symptoms in Parkinson’s disease. J Mol Neurosci 2024;74:28.
133. Libro R, Bramanti P, Mazzon E. The role of the Wnt canonical signaling in neurodegenerative diseases. Life Sci 2016;158:78-88.
134. Vallée A, Lecarpentier Y, Vallée JN. Circadian rhythms and energy metabolism reprogramming in Parkinson’s disease. Curr Issues Mol Biol 2019;31:21-44.
135. L’episcopo F, Serapide MF, Tirolo C, et al. A Wnt1 regulated Frizzled-1/β-Catenin signaling pathway as a candidate regulatory circuit controlling mesencephalic dopaminergic neuron-astrocyte crosstalk: Therapeutical relevance for neuron survival and neuroprotection. Mol Neurodegener 2011;6:49.
136. Yang S, Qin C, Hu ZW, et al. Microglia reprogram metabolic profiles for phenotype and function changes in central nervous system. Neurobiol Dis 2021;152:105290.
137. Pajares M, I Rojo A, Manda G, Boscá L, Cuadrado A. Inflammation in Parkinson’s disease: mechanisms and therapeutic implications. Cells 2020;9:1687.
138. Colonna M, Butovsky O. Microglia function in the central nervous system during health and neurodegeneration. Annu Rev Immunol 2017;35:441-68.
139. Lavisse S, Goutal S, Wimberley C, et al. Increased microglial activation in patients with Parkinson disease using [18F]-DPA714 TSPO PET imaging. Parkinsonism Relat Disord 2021;82:29-36.
140. Gu R, Zhang F, Chen G, et al. Clk1 deficiency promotes neuroinflammation and subsequent dopaminergic cell death through regulation of microglial metabolic reprogramming. Brain Behav Immun 2017;60:206-19.
141. Tu D, Gao Y, Yang R, Guan T, Hong JS, Gao HM. The pentose phosphate pathway regulates chronic neuroinflammation and dopaminergic neurodegeneration. J Neuroinflammation 2019;16:255.
142. Qiao H, He X, Zhang Q, et al. Alpha-synuclein induces microglial migration via PKM2-dependent glycolysis. Int J Biol Macromol 2019;129:601-7.
143. Cheng SC, Quintin J, Cramer RA, et al. mTOR- and HIF-1α-mediated aerobic glycolysis as metabolic basis for trained immunity. Science 2014;345:1250684.
144. Baik SH, Kang S, Lee W, et al. A breakdown in metabolic reprogramming causes microglia dysfunction in Alzheimer’s disease. Cell Metab 2019;30:493-507.e6.
145. Lu J, Wang C, Cheng X, et al. A breakdown in microglial metabolic reprogramming causes internalization dysfunction of α-synuclein in a mouse model of Parkinson’s disease. J Neuroinflammation 2022;19:113.
146. Szwed A, Kim E, Jacinto E. Regulation and metabolic functions of mTORC1 and mTORC2. Physiol Rev 2021;101:1371-426.
147. Brekk OR, Honey JR, Lee S, Hallett PJ, Isacson O. Cell type-specific lipid storage changes in Parkinson’s disease patient brains are recapitulated by experimental glycolipid disturbance. Proc Natl Acad Sci U S A 2020;117:27646-54.
148. Choe CU, Petersen E, Lezius S, et al. Association of lipid levels with motor and cognitive function and decline in advanced Parkinson’s disease in the Mark-PD study. Parkinsonism Relat Disord 2021;85:5-10.
Cite This Article
How to Cite
Download Citation
Export Citation File:
Type of Import
Tips on Downloading Citation
Citation Manager File Format
Type of Import
Direct Import: When the Direct Import option is selected (the default state), a dialogue box will give you the option to Save or Open the downloaded citation data. Choosing Open will either launch your citation manager or give you a choice of applications with which to use the metadata. The Save option saves the file locally for later use.
Indirect Import: When the Indirect Import option is selected, the metadata is displayed and may be copied and pasted as needed.
About This Article
Special Issue
Copyright
Data & Comments
Data
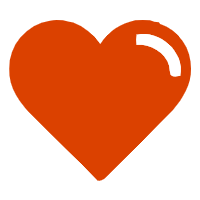
Comments
Comments must be written in English. Spam, offensive content, impersonation, and private information will not be permitted. If any comment is reported and identified as inappropriate content by OAE staff, the comment will be removed without notice. If you have any queries or need any help, please contact us at [email protected].