胆管癌对葡萄糖的依赖:治疗发展的机会(translate by chatgpt 4.0)
摘要 (translate by chatgpt 4.0)
关键词 (translate by chatgpt 4.0)
INTRODUCTION
Cholangiocarcinoma (CCA), or malignancy of bile duct epithelia, is one of the emerging cancers whose incidence is increasing worldwide[1]. It is the second most prevalent primary liver cancer and accounts for 3% of all gastrointestinal tract cancers[2]. CCA can be anatomically classified into intrahepatic and extrahepatic types, in which the latter can be classified into perihilar type when the tumor is located between the second order of the biliary tree to the cystic duct and distal type when the tumor is distal to the cystic duct until the ampulla of Vater. Considering rare malignancy in Western countries, a high incidence rate has been found in East and Southeast Asian countries, up to 85 cases per 100,000 population in Thailand[1,2]. Due to late presentation and the aggressive nature of the disease, CCA leads to high mortality each year[3]. A lot of efforts have been made to address the biological background of this cancer to get a better understanding, which might lead to the development of effective therapeutic modalities[4-8]. One of the approaches that has garnered attention in the research field is metabolic reprogramming[9-11].
Like other cancers, metabolic reprogramming in CCA has been found important for cancer aggression and advancement[9,11,12]. CCA cells modify metabolic pathways to benefit their survival and compete with the surrounding normal tissues. The reprogramming of energetic nutrients such as glucose[12,13], amino acids[14,15], and fatty acids[16,17] is reported for their involvement with CCA carcinogenesis and progression. In addition, many metabolic factors have been experimentally reported that may be involved in the advancement of CCA and thus lead to poor prognosis of patients. Metabolic disorders, e.g., diabetes mellitus (DM)[18,19] and obesity[20,21], have also been studied for their promoting effects on CCA biology. The abnormal metabolic processes in these diseases may favor the metabolism of CCA cells and thus promote cancer progression. Especially in DM, in which patients usually experience chronic hyperglycemia or high blood glucose levels[22,23], which can be utilized in a higher glucose-desired state of aerobic glycolysis or the Warburg effect[24]. DM and hyperglycemia are then the emerging factors that might directly play roles in the aggression and progression of CCA.
DM has been studied for decades for its associations with the increased risk of CCA development and also poor survival of CCA patients[23,25,26]. It is hypothesized that the effects on CCA risk are probably due to the mitogenic effects of insulin in a state of compensatory hyperinsulinemia[22,27]. However, only one study shows an association between using exogenous insulin and the increased risk of extrahepatic CCA to date[28]. The effects of other anti-diabetic medications involving increased insulin secretion, such as insulin secretagogue or incretin-based therapy, have not been agreed upon regarding the risk of CCA development[22,27]. Thus, the effects of high glucose on CCA progression are suspicious and have been investigated later. CCA cells have been shown to have higher glucose consumption; thus, the glucose transporters (GLUTs) in this cancer are usually upregulated and associated with poor survival of patients[29-31]. As mentioned, glucose is a supplier for Warburg effects and, thereby, may straightforwardly increase the glycolysis of CCA cells to meet the high energy requirements. However, glucose has not only been utilized as a nutrient or precursor for glycolysis in CCA cells. High glucose levels have been reported for their regulatory roles in GLUTs[32] and glycolytic gene expressions[18]. It can also be shunted to the other metabolic pathways, e.g., pentose phosphate pathways (PPP) and hexosamine biosynthetic pathways (HBP), that might also support the aggressive phenotypes of CCA cells[33-36]. Moreover, high glucose also activates pro-tumorigenic pathways, resulting in CCA cell aggression[37-40]. Therefore, this review aims to update and discuss the increased requirement of glucose in CCA cells for energy metabolisms and other aspects. The opportunity to develop a treatment based on the dysregulation of glucose metabolism is also discussed.
METABOLIC REPROGRAMMING IN CCA
The metabolic reprogramming of cancers has been recognized since Warburg noticed that cancer cells have an increased rate of glycolysis even if they are in an adequate oxygen environment[41]. It is primarily hypothesized that this aerobic glycolysis is a result of impaired mitochondria, which compromise the cells for ineffective oxidative phosphorylation (OXPHOS). However, later studies revealed that although the mitochondria of cancer cells are intact, the rates of glycolysis in some specific cancers are still high[42]. This led to several “next-generation” studies based on the hypotheses that metabolic alterations in cancer are beneficial and are a choice of cancer cells, not because of the inability to utilize nutrients due to defective mitochondrial machinery[43]. This concept also shifts a paradigm of study and expands the discovery of cancer metabolism from glucose in glycolysis to the other metabolic pathways, namely amino acid and fatty acid metabolism.
Metabolic reprogramming has been recognized as one of cancer hallmarks[44,45]. In CCA, mutations of genes directly and indirectly involving energy metabolism are reported as driver oncogenes, such as IDH1, IDH2, MYC, and KRAS[6,7,46,47]. Targeting genes or proteins in the metabolic pathways becomes a promising strategy for cancer treatments[48]. Glucose metabolism has been well studied in CCA, similar to other cancers, and it has a high impact on anti-cancer drug development[24]. In addition to glucose metabolism, the metabolic pathways involving the catabolism of glutamine and anabolism of lipids also showed their critical roles in CCA progression. As mentioned, the Warburg effect is more likely a choice of cancer metabolism, which benefits cancer cell growth and proliferation. Glycolytic intermediates and their products can serve as precursors for other biomolecule production. For example, pyruvate, a final product of glycolysis, is a precursor for acetyl coenzyme A synthesis, which is a substrate for fatty acid and cholesterol synthesis. Fatty acid and cholesterol are essential molecules for cell proliferation, and thus, the inhibition of the synthetic pathways of both molecules significantly suppresses CCA cell growth[49-51]. Moreover, as cancer cells preferably utilize glucose in glycolysis to provide a carbon skeleton for biosynthesis, glutamine is a choice for anaplerosis of the tricarboxylic acid (TCA) cycle to replenish energy production. A higher requirement for glutamine is reported in CCA, together with the upregulation of genes involved in glutaminolysis[15]. Inhibition of glutaminolysis is then another promising strategy for CCA treatment, and co-targeting glutaminase and GLUTs showed synergistic effects on CCA inhibition[14]. These suggest that glucose metabolism might be the primary pathway that is reprogrammed in cancers, including CCA, and then it is networked with the other metabolic pathways to orchestrate the driving of CCA progression. The following topics in this review are mainly focused on this particular aspect and extend to the recent discovery that reported non-metabolic roles of glucose in CCA, which is another emerging aspect and has the potential for therapeutic development.
WARBURG EFFECT AND THE PROGRESSION OF CCA
Normal proliferating cells in multicellular organisms display a unique energy metabolism with strict regulation to ensure proper cell functioning and a balance between cell cycle arrest and cell proliferation[52,53]. This balance is essential to maintain proper cellular homeostatic responses and requires the process of generating energy in the form of ATP obtained from rather complex molecules. Glucose, a major source of carbohydrates, is a molecule that is primarily utilized for ATP production to meet high metabolic demands. In normal healthy cells, glucose is converted to pyruvate to yield a small amount of ATP via the process of glycolysis. The pyruvate derived from glycolysis is then transported from the cytosol to the mitochondria to be oxidized into acetyl coenzyme A, which is further incorporated into the TCA cycle in the form of citrate and generates abundant ATPs in a flowing mitochondrial OXPHOS.
Cancer cells, including CCA, on the contrary, have the ability to alter this normal metabolic process. This change in cancer cell bioenergetics is also referred to as metabolic reprogramming[54], one of the hallmarks of cancer, which is shown to influence multiple factors to support tumor development and malignant transformation[44,45]. Consequently, this aberrant reprogramming facilitates cancer progression, including activation of oncogenes, alteration of receptor-initiated signaling pathways, and deregulation of cellular energetics. Like other cancers, CCA cells require a lot of energy to maintain their continuous growth[12]. Some oncogenic mutations lead to metabolic reprogramming of CCA, such as the mutation of KRAS. KRAS activation can enhance glucose uptake by upregulation of GLUTs and several glycolytic enzymes[55]. The overexpression of GLUTs has been reported in KRAS mutant CCA cell lines, and the upregulated GLUT1 was associated with progressive carcinogenesis and poor survival of CCA patients[30,56]. In addition, an increase in glucose uptake and, thereby, glycolysis, parallel with large amounts of lactate secretion, provides an acidic tumor microenvironment for facilitating CCA invasion, is also observed[57-59]. Moreover, Colyn
Although aerobic glycolysis is a less efficient metabolic means of generating ATP compared to OXPHOS in the mitochondria, cancer cells still benefit substantially from high glycolytic rates, enabling them to survive and proliferate accordingly[43]. The rationale behind this occurrence is explained by the higher rate of ATP production by glycolysis, which is considered to be much more rapid compared to normal OXPHOS and, thus, can be more efficiently employed to meet the increase in ATP demand, gaining a selective advantage under tumor microenvironment where nutrients have limited availability. Secondly, increased glycolysis results in an increase in the synthesis of glycolytic metabolites that are necessary biosynthetic precursors for many cellular pathways, such as the production of lipids, amino acids, and nucleotides due to the overexpression of pyruvate kinase M2 (PKM2) which causes a reduction in conversion of phosphoenolpyruvate to pyruvate in the final, irreversible step of glycolysis[62,63]. The upregulated PKM2 is also evident in CCA, and it is associated with a poor prognosis in CCA patients who have DM[18]. High expression levels of PKM2, which causes the retention of glycolytic intermediates, can benefit cancer cells in many ways. For instance, the rise in levels of glucose-6-phosphate (G6P), one of the glycolytic intermediates for shunts in PPP responsible for generating nucleotide synthetic precursors, is indispensable for DNA synthesis and cancer cell progression, while rises in glyceraldehyde-3-phosphate (G3P) account for an increase in triglyceride and serine biosynthesis[64]. Hence, this transformed glucose metabolism is highly useful for cancer cells to unlimitedly supply themselves with biosynthetic precursors to satisfy their highly proliferative characteristics. Moreover, subsequent nicotinamide adenine dinucleotide phosphate (NADPH) derived from the PPP provides adequate reduced glutathione for resistance to chemotherapeutic agents, providing additional rationale for how cancer cells may benefit from the Warburg effect.
Apart from altered glucose metabolism, the Warburg effect also influences the expression of signaling molecules and transcriptional regulatory factors, which promotes cancer occurrence. Oncogenic mutations cause upregulation of various cancer-promoting factors such as HIF1, SIRTs, and MYC[65]. These genes have been reported in CCA cells exhibiting the Warburg effect, while the downregulation of tumor suppressor genes was also manifested. Reprogramming also affects signal transduction pathways, consequently leading to increased activation of downstream signaling that triggers tumor initiation and progression[10,12]. The PI3K/Akt/mTOR regulatory pathway is one of the pathways being explored in CCA cells[66,67]. Stimulation of the PI3K/Akt/mTOR pathway indirectly enhances the Warburg effect by activating the transcription factor cMyc, HIF-1, and SIRTs[9]. Together, this promotes the expression of glycolytic proteins and enzymes such as GLUT1, PKM2, phosphofructokinase 1 (PFK1), hexokinase II (HK2), and lactate dehydrogenase A (LDHA), hence explaining the high glycolytic rates seen in the cancer cells including CCA. Glycolytic inhibitors are therefore considered therapeutic interventions that have an anti-Warburg effect by inhibiting cancer cell growth and progression[48,68,69].
Hypoxia-inducible factor 1 (HIF-1) is another master regulatory protein for glycolysis by upregulating genes of GLUTs and glycolytic enzymes[70,71]. Under physiological oxygen level, HIF-1 is modified by prolyl hydroxylation by the enzyme prolyl hydroxylase (PHD) and interacts with tumor-suppressor protein von Hippel-Lindau (vHL) to be targeted for proteasomal degradation[72]. However, PHD is inhibited during hypoxic conditions, and thus, HIF-1 is stabilized. As seen in cancer cells, mutations in oncogenes and tumor suppressor genes such as vHL ultimately lead to the stabilization of HIF-1, suppressing mitochondrial activity. HIF-1 not only promotes cancer progression and malignant transformation by potentiating transcriptions of GLUT1, HK2, PKM2, PFK1, and LDHA, but also increases the expression of pyruvate dehydrogenase kinase (PDK), which inactivates the pyruvate dehydrogenase complex. Additionally, HIF-1 upregulates vascular endothelial growth factor (VEGF), thereby resulting in increased angiogenesis. The upregulation of HIF-1α, a subunit of HIF-1, in a normoxic and hypoxic condition of CCA cells was also reported[63-76]. The current findings of these effects in CCA are summarized in Figure 1.
Figure 1. Regulatory roles of HIF1 in the expression of genes associated with glycolysis in CCA. HIF1, especially a subunit HIF1-α, is a master regulatory protein that upregulates many genes encoding the enzymes and transporters that play roles in glucose metabolism, such as GLUT1, HK2, PFK1, PKM2, and GFAT. Glc: Glucose; G-6-P: glucose-6-phosphate; F-6-P: fructose-6-phosphate; F1,6BP: fructose-1,6,-bisphosphate; PEP: phosphoenolpyruvate; G6PD: glucose-6-phosphate dehydrogenase; PPP: pentose phosphate pathway; HBP: hexosamine biosynthetic pathway; HIF1: hypoxia-inducible factor 1; CCA: cholangiocarcinoma; GLUT1: glucose transporter 1; HK2: hexokinase II; PFK1: phosphofructokinase 1; PKM2: pyruvate kinase isoform M2; LDHA: lactate dehydrogenase A; GFAT: glutamine fructose-6-phosphate amidotransferase.
Emphasizing the Warburg effect, studies suggest that mitochondrial dysfunction is essential in promoting tumor progression[77], as several underlying mechanisms regarding deregulated cellular energetics are associated with this phenomenon. Oncogenes such as HIF1 and tumor suppressor gene TP53 impose a direct link toward mitochondrial activity. Thus, impairments of these genes due to metabolic reprogramming consequently alter normal cellular metabolism[78]. Firstly, increased expression of pyruvate dehydrogenase kinase 1 (PDHK1) inhibits the conversion of pyruvate to acetyl coenzyme A by phosphorylating the pyruvate dehydrogenase, resulting in less substrate entering the TCA cycle and thus repressing mitochondrial oxidative mechanism. Secondly, HK2, a prevalent isoform with the most enzymatic activity that catalyzes the conversion of glucose to G6P, can interact with voltage-dependent anion channels (VDACs) on the outer membrane of the mitochondria; it is this VDAC-HK2 interaction that gives cancer cells an anti-apoptotic property by blocking cytochrome c release into the cytoplasm, leading to no caspase activation. Thirdly, the upregulation of GLUTs, especially GLUT1 in CCA cells, which divert its metabolic flux from OXPHOS to glycolysis as a response to hypoxia and inhibition of mitochondrial respiration, may be explained by the increased activation of AMP-activated protein kinase (AMPK) upon decreased ATP production as a subsequent result of mitochondrial dysfunction. Moreover, the supplementation of glycolysis in CCA was also supported by the uptake of fructose, as reported in the study that showed the upregulation of GLUT5[79].
In addition, SIRT3, a nuclear-encoded mitochondrial protein deacetylase, is shown to regulate several mitochondrial protein activities, including OXPHOS, and is thought to play an antitumor role. Loss of SIRT3 function results in mitochondrial dysfunction, increased intracellular reactive oxygen species (ROS) levels, and tumorigenesis. Interestingly, one study shows that decreased expression of SIRT3 corresponds to an increase in the glycolytic rate in CCA, highlighting an inverse correlation with HIF-1, therefore suggesting SIRT3 as a possible molecule exhibiting an anti-Warburg effect[80]. Another study also showed that high glucose levels can increase the levels of ROS, which promotes the aggressiveness of CCA[81]. Taken together, Warburg effects might be a linkage among these pro-tumorigenic pathways that enhance the aggressiveness of CCA cells and promote the disease progression.
Another pathway that is demonstrated to inhibit apoptosis in CCA, thereby promoting cancer aggressiveness, is the SIRT2/cMYC pathway[82]. SIRT2 is classified as a class III histone deacetylase (HDAC), responsible for post-translational modification, an important step in controlling a variety of metabolic regulatory processes. There has been growing evidence that SIRT2 can promote malignant tumor transformation by upregulating its downstream target transcription factor-cMYC. Pyruvate is a known inhibitor of HDAC3, such as SIRT2. So, with a low sustained level of pyruvate due to overexpression of lactate dehydrogenase (LDH) and PKM2, SIRT2 is stabilized. This, in turn, allows SIRT2 to deacetylate cMYC, thereby inhibiting its ubiquitin degradation. Conversely, the mechanisms by which the SIRT2/cMYC pathway has been shown to contribute to metabolic reprogramming in CCA have been explored. A study using CCA cell lines revealed that these molecules, in coordination, target PDHA1 activity in response to site-specific phosphorylation, thus inhibiting the TCA and OXPHOS. The increased lactate secretion levels in the CCA cell line with overexpressed SIRT2 further reinforce the effect of this pathway on the Warburg effect. Alternatively, in a similar study, the SIRT2/cMYC pathway is also revealed to promote serine anabolic metabolism by increasing the conversion of glucose to serine via activation of the serine synthesis pathway (SSP). As aforementioned, the transformed glucose oxidative mechanism led to an increase in serine biosynthesis[82,83]. However, the specific role of SSP and its interplay with cancer has not been largely explored. Nevertheless, a recent study indicates that downstream activation of SSP plays a crucial role in antioxidant production (T-GSH/GSSG/GSH) and anti-apoptotic effect in CCA cell lines. Serine is a non-essential amino acid, and its function in cancer progression has been assessed. It is shown that serine can act as the ROS scavenger, contributing to redox homeostasis. Consequently, this provides CCA with an anti-oxidative tumor microenvironment enhanced for growth and proliferation and protects CCA cells from oxidative stress-induced apoptosis. The inhibition of SSP is then a promising strategy to inhibit CCA cell growth, particularly by inhibiting a gain of benefit from metabolic reprogramming[84]. Collectively from these findings, the metabolic reprogramming functions of the SIRT2/cMYC pathway may attract potential attention for further anti-Warburg therapies.
DIABETOGENIC GLUCOSE AND ITS NON-WARBURG’S ROLES IN CCA
In addition to being an energy source, glucose at a supraphysiological level may have other functions rather than a simple nutrient[37]. Some straightforward reasons are that glucose can be shunted into collateral pathways such as the PPP and the HBP, which are important for other biomolecule anabolisms[85]. The HBP is responsible for synthesizing an amino sugar called N-acetylglucosamine (GlcNAc), a precursor of an O-link GlcNAc glycosylation or O-GlcNAcylation[86]. The glycosylations of GlcNAc to proteins have several crucial biological functions, such as activation of the proteins, increasing protein stability, or even activating the O-GlcNAcylated proteins for cellular degradations. The changes in protein properties become significant for CCA development and progression when dysregulated O-GlcNAcylation occurs at tumor suppressors or oncoproteins[33,34,87]. High glucose level was shown to promote global O-GlcNAcylation in CCA cells by upregulating the expression of glutamine fructose-6-phosphate amidotransferase (GFAT)[33], the rate-limiting enzyme in HBP, thus resulting in an increase in glucose flux for GlcNAc production in CCA cells. The increased GlcNAcylation of oncoproteins, namely nuclear factor-κB (NF-κB)[34], vimentin[33], (Forkhead Box O3) FOXO3[88], mannosidase alpha class 1A member 1[88], and heterogeneous nuclear ribonucleoprotein-K (hnRNP-K)[89], have been reported for their involvement with aggressive phenotypes of CCA, e.g., proliferation, migration, and invasion [Figure 2]. Thus, glycosylations are another underlying mechanism of high glucose promoting the progression of CCA, which is non-negligible.
Figure 2. Non-energetic roles of glucose in the progression of CCA. Apart from being metabolized in glycolysis to yield ATP, glucose can be shunted to the hexosamine biosynthetic pathway to be a precursor for GlcNAc synthesis. GlcNAc is utilized in an O-GlcNAcylation, which alters the stability of several oncoproteins and hence promotes the aggressive phenotypes of CCA cells. On the other hand, glucose can activate several intracellular signaling pathways such as GSK3β/β-Cat, JAK2/STAT3, and NF-κB. Moreover, high glucose also upregulates IL-1β and IL-6, which are the upstream activators of those signaling pathways. Altogether, these mechanisms explain in part how diabetogenic glucose promotes CCA progression. However, the in-depth molecular mechanisms remain elucidated. VIM: Vimentin; FOXO3: Forkhead Box O3; MAN1A1: Mannosidase Alpha Class 1A Member 1; hnRNPK: Heterogeneous Nuclear Ribonucleoprotein K; CCND1: cyclin D1; CCNA: cyclin A; MMP2: matrix metalloproteinase-2; MMP7: matrix metalloproteinase-7; CCA: cholangiocarcinoma; GlcNAc: N-acetylglucosamine; O-GlcNAcylation: O-link GlcNAc glycosylation; GSK3β/β-Cat: glycogen synthase kinase-3β/β-catenin; JAK2/STAT3: Janus kinase 2/signal transducer and activator of transcription 3; NF-κB: nuclear factor-κB; IL-1β: interleukin-1β; IL-6: interleukin-6.
With unclear mechanisms, high glucose also affects the intracellular signaling pathways of CCA cells. High glucose levels activate the signal transducer and activator of transcription 3 (STAT3) pathways by increasing STAT3 phosphorylation and promoting nuclear translocation in CCA cell lines cultured in high glucose and tumor tissues from patients with CCA who had DM[37,38]. The activation of STAT3 in high glucose was also found to crosstalk with NF-κB by the upregulation of communicating cytokines, interleukin-6 (IL-6) and interleukin-1β (IL-1β), which reciprocally activate STAT3 and NF-κB pathways[39,90]. The activation of STAT3 has been shown to have several aggressive consequences in CCA cells, such as increased proliferation and metastatic potential. Moreover, upregulated IL-1β in CCA cells under hyperglycemia was shown as one of the factors that promote CCA growth in vitro and in vivo[90]. High glucose also regulates other transcription factors, such as β-catenin[40]. By controlling several transcription factors, high glucose can upregulate many proteins that enhance CCA’s aggressive phenotypes, i.e., cell cycle regulatory proteins[19]. Altogether, glucose in the hyperglycemic ranges is one factor that promotes CCA progression. Rather than being utilized by glycolysis, multiple pathways respond to the increased glucose levels, which leads to the higher aggression of CCA. Concerning these points, CCA patients with DM who have chronic hyperglycemia might then be prone to have a poorer prognosis than those with euglycemia[18,19], as evidenced by the association between high-glucose-induced molecules and poorer survival of CCA patients[18,19]. The development of novel therapeutic agents should thus take the underlying DM and hyperglycemia into consideration as factors that might compromise the effectiveness of treatment. In addition, the development of any therapeutic agents that target high-glucose-induced molecules would help reverse the aggressive phenotypes of CCA cells in hyperglycemic conditions as reported by using static (STAT3 inhibitor)[37], dehydroxymethylepoxyquinomicin (NF-κB inhibitor)[39], and anakinra (IL-1 receptor antagonist), to inhibit CCA cell proliferation under diabetic condition in the in vitro and in vivo model[90].
OPPORTUNITIES FOR THERAPEUTIC DEVELOPMENT TARGETING GLUCOSE METABOLISM IN CCA
The current treatments of CCA in clinical practice are facing several limitations. The only highly effective and curative treatment for CCA is radical surgical resection. However, this curative treatment can be expected only when the tumor is localized, which is limited to the very early stage of CCA. Standard chemotherapeutic drugs can prolong the survival of patients in a few months, but most patients experience undesired adverse effects[2]. The approved targeted therapies against fibroblast growth factor receptor 2 (FGFR2) are effective only in patients with FGFR2 mutation, which is found to be approximately 15% worldwide and less than 1% in liver fluke-associated CCA[91]. Therefore, the research and development of therapeutic agents for CCA remains needed, and targeting metabolic reprogramming is one of the hopes to improve the therapeutic outcomes of the patients.
High glucose uptakes in CCA cells are correlated with the high expression of glucose transporter families such as GLUT1[30,31], GLUT2[31], and GLUT5[79], which provide opportunities to develop therapeutic agents targeting these molecules. The gradual upregulation of GLUT1 was demonstrated along with cholangiocarcinogenesis in the liver-fluke-associated CCA in a hamster model[30]. High expressions of GLUT1 in tumor tissues from patients with CCA were also associated with shorter survival of patients[29,30], and thus, silencing GLUT1 in CCA cell lines showed a significantly decreased CCA cell proliferation[30]. Apart from GLUT1, which is mainly responsible for glucose uptake, immunohistochemistry staining of CCA tissue microarrays and the study of CCA cell lines discovered significantly higher SLC2A5 (GLUT5) expression in CCA than in normal biliary epithelial cells[79]. Upon knocking down the expression of GLUT5 using siRNA, suppression of CCA cell proliferation was observed in a time-dependent manner, along with the inhibition of tumor migration and invasion. Since GLUT5 has a higher affinity to fructose than the other sugars, these results suggested the dependency on the fructose of CCA cells. Fructose is another monosaccharide that replenishes glycolysis by being metabolized to glycolytic intermediates. Targeting these GLUTs and glycolysis might be promising for developing CCA treatment.
HK2, the first rate-limiting step of glucose metabolism, is another potential target for drug development. Overexpression of HK2 in CCA tumor tissues was significantly associated with poor overall survival[68]. Using siRNA to silence the expression of HK2 in CCA cell lines resulted in a significant reduction in clonogenicity along with their migration capacity. The requirement of the Warburg effect in CCA was shown in CCA cells with high expression of PKM2, the isoform of pyruvate kinase that promotes glycolysis and the retention of glycolytic intermediate[18,69]. PKM2 depletion sensitized CCA cells, HCC9810 and RBE, to gemcitabine, a chemotherapeutic drug[92-94]. Using PKM2 inhibitors also exhibits significant antitumor effects on CCA cells[69]. On the contrary, another study by Tang et al. also shows that metformin can inhibit the Warburg effect in CCA cells[95]. Still, it exerts a minimal impact on inducing CCA cells to apoptosis. However, metformin, in combination with BG-45, a HDAC 3 inhibitor, significantly increased CCA cell apoptosis and decreased the expression of LDHA along with metabolite fluctuations. Some potential molecular targets that have been reported in CCA are summarized in Figure 3.
Figure 3. Potential CCA therapeutic targets based on glucose metabolism. Upregulation of genes involving glycolysis in CCA provides opportunities to develop targeted therapies. Previous reports showed that inhibiting the expression of GLUT1, GLUT5, and HK2 by siRNA, and LDHA by metformin combined with BG-45, significantly reduced CCA cell proliferation and induced cell apoptosis. Additionally, inhibition of the activities of GLUT1, HK2, and PKM2 by their inhibitor also retarded CCA cell growth. Glc: Glucose; G-6-P: glucose-6-phosphate, F-6-P: fructose-6-phosphate; PEP: phosphoenolpyruvate; CCA: cholangiocarcinoma; GLUT1: glucose transporters 1; GLUT5: glucose transporter 5; HK2: hexokinase II; LDHA: lactate dehydrogenase A; PKM2: pyruvate kinase isoform M2.
Although promising results were obtained in the preclinical study, clinical trials of the agents targeting GLUT, glycolytic enzymes, and the associated molecules remained limited in CCA. This might result from a lack of a definite tumor-specificity of these enzymes, and then those agents may affect the normal, highly proliferative tissues[96]. A clinical trial phase I using metformin for IDH1/2 mutation CCA also showed unfavorable results[97,98]. Similarly, clinical trials of GLUT1 and HK2 inhibitors in other cancers also showed unsatisfactory outcomes, and some were terminated[96]. A combination of devimistat, pyruvate dehydrogenase and α-ketoglutarate dehydrogenase inhibitors, with gemcitabine/cisplatin for CCA treatment, did not meet a satisfactory overall response rate[99,100]. Comparisons of the advantages and limitations of currently available treatment strategies and the proposed metabolic targeted therapy from the aforementioned part could be summarized in Table 1. The limitation of less specific targeting by synthetic compounds may be overcome by using other strategies, such as the siRNA that could be designed to suppress the expression of specific or preferable isoforms of genes involving metabolic reprogramming, together with the development of effective delivery systems. To date, targeting glucose metabolism remains a significant challenge for developing cancer treatment, including CCA, which needs further investigations for a better outcome with minimal adverse effects. Some clinical trials are underway investigating targeting metabolisms in CCA, such as the inhibitors of IDH1 and IDH2[101], as well as sphingolipid metabolism[102].
Comparisons of advantages and limitations among currently available treatments and proposed targeted metabolic treatment
Therapeutic methods | Advantages | Limitations |
Surgical removal of tumors | - Curative in the early, non-invasive stage | - Not applicable for patients with metastatic disease - Require a skillful hepato-biliary specialist for a radical curative resection |
Chemotherapy | - Can be used for metastatic cancer - Widely available at an affordable cost | - Less specific to cancer cells, usually causes adverse effects for highly proliferative tissues - Prolong a few months survival time |
FGFR targeted therapy | - Effective and highly specific targeting at FGFR fusion or rearrangements | - Only a small proportion (approximately 15%) of CCA patients possess this mutation - Fewer than 1% of liver-fluke-associated CCA cases possess this mutation |
Targeted metabolic treatment | - Potential to develop tumor-specific isoforms - Theoretically useful for metastatic cancer - Developing small RNA targeting at the transcription level of metabolic genes could be a benefit | - Absolutely specific isoforms for tumors are rare; most metabolic genes expressed in cancer are expressed in highly proliferative tissues - Have not been extensively studied at clinical levels |
CONCLUSION
Like other cancers, metabolic reprogramming also occurs and benefits CCA’s development and progression. High glucose consumption of CCA cells, favored with high blood glucose conditions in patients with DM, is part of the driving force for Warburg effects. On the other hand, high glucose also promotes CCA progression via a collateral metabolic pathway such as PPP and HBP. Moreover, high glucose can activate several pro-tumorigenic intracellular signaling pathways, resulting in aggressive phenotypes of CCA. The attempt to target molecules involving Warburg effects is promising in preclinical experiments. However, the success rate of clinical studies remains limited. Further development of therapeutic agents targeting the Warburg effects and related molecules remains a challenge that may require more efforts to overcome this metabolic dysregulation in CCA.
DECLARATIONS
Acknowledgments
The authors would like to thank Cho-Kalaphruek Excellent Research Project for Medical Students, Faculty of Medicine, Khon Kaen University, for the general support of Singkarin A and Sriphaiboon J.
Authors’ contributions
Conceptualized and designed the review topics: Singkarin A, Saengboonmee C
Reviewed the literature and wrote the first draft of the manuscript: Singkarin A, Kusoltech C, Sriphaiboon J, Saengboonmee C
Illustrated the figures: Singkarin A, Kusoltech C
Verified the accuracy of the data and edited the manuscript: Phoomak C, Saengboonmee C
All authors contributed to editorial changes in the manuscript.
All authors read and approved the final manuscript.
All authors have participated sufficiently in the work and agreed to be accountable for all aspects of the work.
Availability of data and materials
Not applicable.
Financial support and sponsorship
This work was supported by the Mekong-Lancang Cooperation Special Fund. The funding agency has no roles in the experiment design, collection, analysis, interpretation of data, and writing of the manuscript.
Conflicts of interest
All authors declared that there are no conflicts of interest.
Ethical approval and consent to participate
Not applicable.
Consent for publication
Not applicable.
Copyright
© The Author(s) 2025.
REFERENCES
2. Banales JM, Marin JJG, Lamarca A, et al. Cholangiocarcinoma 2020: the next horizon in mechanisms and management. Nat Rev Gastroenterol Hepatol. 2020;17:557-88.
3. Treeprasertsuk S, Poovorawan K, Soonthornworasiri N, et al. A significant cancer burden and high mortality of intrahepatic cholangiocarcinoma in Thailand: a nationwide database study. BMC Gastroenterol. 2017;17:3.
4. Pupacdi B, Loffredo CA, Budhu A, et al; TIGER-LC Consortium. The landscape of etiological patterns of hepatocellular carcinoma and intrahepatic cholangiocarcinoma in Thailand. Int J Cancer. 2024;155:1387-99.
5. Chaisaingmongkol J, Budhu A, Dang H, et al. Common molecular subtypes among Asian hepatocellular carcinoma and cholangiocarcinoma. Cancer Cell. 2017;32:57-70.e3.
6. Chan-On W, Nairismägi ML, Ong CK, et al. Exome sequencing identifies distinct mutational patterns in liver fluke-related and non-infection-related bile duct cancers. Nat Genet. 2013;45:1474-8.
7. Jusakul A, Cutcutache I, Yong CH, et al. Whole-genome and epigenomic landscapes of etiologically distinct subtypes of cholangiocarcinoma. Cancer Discov. 2017;7:1116-35.
8. Hong JH, Yong CH, Heng HL, et al. Integrative multiomics enhancer activity profiling identifies therapeutic vulnerabilities in cholangiocarcinoma of different etiologies. Gut. 2024;73:966-84.
9. Raggi C, Taddei ML, Rae C, Braconi C, Marra F. Metabolic reprogramming in cholangiocarcinoma. J Hepatol. 2022;77:849-64.
10. Pastore M, Lori G, Gentilini A, et al. Multifaceted aspects of metabolic plasticity in human cholangiocarcinoma: an overview of current perspectives. Cells. 2020;9:596.
11. Yang F, Hilakivi-Clarke L, Shaha A, et al. Metabolic reprogramming and its clinical implication for liver cancer. Hepatology. 2023;78:1602-24.
12. Pant K, Richard S, Peixoto E, Gradilone SA. Role of glucose metabolism reprogramming in the pathogenesis of cholangiocarcinoma. Front Med. 2020;7:113.
13. Zhen Y, Liu K, Shi L, et al. FGFR inhibition blocks NF-ĸB-dependent glucose metabolism and confers metabolic vulnerabilities in cholangiocarcinoma. Nat Commun. 2024;15:3805.
14. Yang SM, Kim J, Lee JY, Lee JS, Lee JM. Regulation of glucose and glutamine metabolism to overcome cisplatin resistance in intrahepatic cholangiocarcinoma. BMB Rep. 2023;56:600-5.
15. Cao J, Zhang C, Jiang GQ, et al. Expression of GLS1 in intrahepatic cholangiocarcinoma and its clinical significance. Mol Med Rep. 2019;20:1915-24.
16. Xu L, Zhang Y, Lin Z, et al. FASN-mediated fatty acid biosynthesis remodels immune environment in Clonorchis sinensis infection-related intrahepatic cholangiocarcinoma. J Hepatol. 2024;81:265-77.
17. Ruiz de Gauna M, Biancaniello F, González-Romero F, et al. Cholangiocarcinoma progression depends on the uptake and metabolization of extracellular lipids. Hepatology. 2022;76:1617-33.
18. Fu K, Yang X, Wu H, Gong J, Li X. Diabetes and PKM2 affect prognosis in patients with intrahepatic cholangiocarcinoma. Oncol Lett. 2020;20:265.
19. Saengboonmee C, Detarya M, Sangkhamanon S, Sawanyawisuth K, Seubwai W, Wongkham S. High glucose induced upregulation of cyclin a associating with a short survival of patients with cholangiocarcinoma: a potential target for treatment of patients with diabetes mellitus. Nutr Cancer. 2022;74:1734-44.
20. Osataphan S, Mahankasuwan T, Saengboonmee C. Obesity and cholangiocarcinoma: a review of epidemiological and molecular associations. J Hepatobiliary Pancreat Sci. 2021;28:1047-59.
21. Lee JW, Yoo ID, Hong SP, et al. Prognostic impact of visceral adipose tissue imaging parameters in patients with cholangiocarcinoma after surgical resection. Int J Mol Sci. 2024;25:3939.
22. Saengboonmee C, Seubwai W, Lert-Itthiporn W, Sanlung T, Wongkham S. Association of diabetes mellitus and cholangiocarcinoma: update of evidence and the effects of antidiabetic medication. Can J Diabetes. 2021;45:282-90.
23. Saengboonmee C, Seubwai W, Wongkham C, Wongkham S. Diabetes mellitus: possible risk and promoting factors of cholangiocarcinoma: association of diabetes mellitus and cholangiocarcinoma. Cancer Epidemiol. 2015;39:274-8.
24. Supabphol S, Seubwai W, Wongkham S, Saengboonmee C. High glucose: an emerging association between diabetes mellitus and cancer progression. J Mol Med. 2021;99:1175-93.
25. Palmer WC, Patel T. Are common factors involved in the pathogenesis of primary liver cancers? J Hepatol. 2012;57:69-76.
26. Clements O, Eliahoo J, Kim JU, Taylor-Robinson SD, Khan SA. Risk factors for intrahepatic and extrahepatic cholangiocarcinoma: a systematic review and meta-analysis. J Hepatol. 2020;72:95-103.
27. Sun H, Qi X. The role of insulin and incretin-based drugs in biliary tract cancer: epidemiological and experimental evidence. Discov Oncol. 2022;13:70.
28. Qi X, He P, Yao H, et al. Insulin therapy and biliary tract cancer: insights from real-world data. Endocr Connect. 2022;11:e210546.
29. Kinjo Y, Naito Y, Akiba J, et al. SUOX and GLUT1 are biomarkers for the prognosis in large duct type intrahepatic cholangiocarcinoma. Hum Pathol. 2022;128:11-9.
30. Thamrongwaranggoon U, Sangkhamanon S, Seubwai W, Saranaruk P, Cha'on U, Wongkham S. Aberrant GLUT1 expression is associated with carcinogenesis and progression of liver fluke-associated cholangiocarcinoma. In Vivo. 2021;35:267-74.
31. Kubo Y, Aishima S, Tanaka Y, et al. Different expression of glucose transporters in the progression of intrahepatic cholangiocarcinoma. Hum Pathol. 2014;45:1610-7.
32. Jóźwiak P, Krześlak A, Bryś M, Lipińska A. Glucose-dependent glucose transporter 1 expression and its impact on viability of thyroid cancer cells. Oncol Rep. 2015;33:913-20.
33. Phoomak C, Vaeteewoottacharn K, Silsirivanit A, et al. High glucose levels boost the aggressiveness of highly metastatic cholangiocarcinoma cells via O-GlcNAcylation. Sci Rep. 2017;7:43842.
34. Phoomak C, Vaeteewoottacharn K, Sawanyawisuth K, et al. Mechanistic insights of O-GlcNAcylation that promote progression of cholangiocarcinoma cells via nuclear translocation of NF-κB. Sci Rep. 2016;6:27853.
35. Zheng P, Pan HH, Zhou XH, et al. Glucose 6 phosphatase dehydrogenase (G6PD): a novel diagnosis marker related to gastrointestinal cancers. Am J Transl Res. 2023;15:2304-28.
36. Qu X, Sheng J, Shen L, et al. Autophagy inhibitor chloroquine increases sensitivity to cisplatin in QBC939 cholangiocarcinoma cells by mitochondrial ROS. PLoS One. 2017;12:e0173712.
37. Saengboonmee C, Seubwai W, Pairojkul C, Wongkham S. High glucose enhances progression of cholangiocarcinoma cells via STAT3 activation. Sci Rep. 2016;6:18995.
38. Detarya M, Thaenkaew S, Seubwai W, et al. High glucose upregulates FOXM1 expression via EGFR/STAT3 dependent activation to promote progression of cholangiocarcinoma. Life Sci. 2021;271:119114.
39. Saengboonmee C, Phoomak C, Supabphol S, et al. NF-κB and STAT3 co-operation enhances high glucose induced aggressiveness of cholangiocarcinoma cells. Life Sci. 2020;262:118548.
40. Saengboonmee C, Sorin S, Sangkhamanon S, et al. γ-aminobutyric acid B2 receptor: a potential therapeutic target for cholangiocarcinoma in patients with diabetes mellitus. World J Gastroenterol. 2023;29:4416-32.
42. Thompson CB, Vousden KH, Johnson RS, et al. A century of the Warburg effect. Nat Metab. 2023;5:1840-3.
43. Heiden MG, Cantley LC, Thompson CB. Understanding the Warburg effect: the metabolic requirements of cell proliferation. Science. 2009;324:1029-33.
46. Ong CK, Subimerb C, Pairojkul C, et al. Exome sequencing of liver fluke-associated cholangiocarcinoma. Nat Genet. 2012;44:690-3.
47. Nakamura H, Arai Y, Totoki Y, et al. Genomic spectra of biliary tract cancer. Nat Genet. 2015;47:1003-10.
48. Olszewski K, Barsotti A, Feng XJ, et al. Inhibition of glucose transport synergizes with chemical or genetic disruption of mitochondrial metabolism and suppresses TCA cycle-deficient tumors. Cell Chem Biol. 2022;29:423-35.e10.
49. Saisomboon S, Kariya R, Boonnate P, et al. Diminishing acetyl-CoA carboxylase 1 attenuates CCA migration via AMPK-NF-κB-snail axis. Biochim Biophys Acta Mol Basis Dis. 2023;1869:166694.
50. Tomacha J, Dokduang H, Padthaisong S, et al. Targeting fatty acid synthase modulates metabolic pathways and inhibits cholangiocarcinoma cell progression. Front Pharmacol. 2021;12:696961.
51. Panawan O, Silsirivanit A, Chang CH, et al. Establishment and characterization of a novel cancer stem-like cell of cholangiocarcinoma. Cancer Sci. 2023;114:3230-46.
52. Diehl FF, Sapp KM, Vander Heiden MG. The bidirectional relationship between metabolism and cell cycle control. Trends Cell Biol. 2024;34:136-49.
53. Rong Y, Darnell AM, Sapp KM, Vander Heiden MG, Spencer SL. Cells use multiple mechanisms for cell-cycle arrest upon withdrawal of individual amino acids. Cell Rep. 2023;42:113539.
54. Allen CNS, Arjona SP, Santerre M, Sawaya BE. Hallmarks of metabolic reprogramming and their role in viral pathogenesis. Viruses. 2022;14:602.
55. Shen X, Niu N, Xue J. Oncogenic KRAS triggers metabolic reprogramming in pancreatic ductal adenocarcinoma. J Transl Int Med. 2023;11:322-9.
56. Tiemin P, Peng X, Qingfu L, et al. Dysregulation of the miR-148a-GLUT1 axis promotes the progression and chemoresistance of human intrahepatic cholangiocarcinoma. Oncogenesis. 2020;9:19.
57. Thamrongwaranggoon U, Kuribayashi K, Araki H, et al. Lactic acidosis induces metabolic and phenotypic reprogramming in cholangiocarcinoma cells via the upregulation of thrombospondin-1. Cancer Sci. 2023;114:1541-55.
58. Thamrongwaranggoon U, Detarya M, Seubwai W, et al. Lactic acidosis promotes aggressive features of cholangiocarcinoma cells via upregulating ALDH1A3 expression through EGFR axis. Life Sci. 2022;302:120648.
59. Thonsri U, Seubwai W, Waraasawapati S, et al. Overexpression of lactate dehydrogenase A in cholangiocarcinoma is correlated with poor prognosis. Histol Histopathol. 2017;32:503-10.
60. Colyn L, Alvarez-Sola G, Latasa MU, et al. New molecular mechanisms in cholangiocarcinoma: signals triggering interleukin-6 production in tumor cells and KRAS co-opted epigenetic mediators driving metabolic reprogramming. J Exp Clin Cancer Res. 2022;41:183.
61. Liu J, Zhang C, Hu W, Feng Z. Tumor suppressor p53 and metabolism. J Mol Cell Biol. 2019;11:284-92.
62. Liu VM, Howell AJ, Hosios AM, Li Z, Israelsen WJ, Vander Heiden MG. Cancer-associated mutations in human pyruvate kinase M2 impair enzyme activity. FEBS Lett. 2020;594:646-64.
63. Israelsen WJ, Vander Heiden MG. Pyruvate kinase: function, regulation and role in cancer. Semin Cell Dev Biol. 2015;43:43-51.
64. Liberti MV, Locasale JW. The Warburg effect: how does it benefit cancer cells? Trends Biochem Sci. 2016;41:211-8.
65. Liao M, Yao D, Wu L, et al. Targeting the Warburg effect: a revisited perspective from molecular mechanisms to traditional and innovative therapeutic strategies in cancer. Acta Pharm Sin B. 2024;14:953-1008.
66. Corti F, Nichetti F, Raimondi A, et al. Targeting the PI3K/AKT/mTOR pathway in biliary tract cancers: a review of current evidences and future perspectives. Cancer Treat Rev. 2019;72:45-55.
67. Tian LY, Smit DJ, Jücker M. The role of PI3K/AKT/mTOR signaling in hepatocellular carcinoma metabolism. Int J Mol Sci. 2023;24:2652.
68. Thamrongwaranggoon U, Seubwai W, Phoomak C, et al. Targeting hexokinase II as a possible therapy for cholangiocarcinoma. BBRC. 2017;484:409-15.
69. Thonsri U, Seubwai W, Waraasawapati S, et al. Antitumor effect of shikonin, a PKM2 inhibitor, in cholangiocarcinoma cell lines. Anticancer Res. 2020;40:5115-24.
70. Infantino V, Santarsiero A, Convertini P, Todisco S, Iacobazzi V. Cancer cell metabolism in hypoxia: role of HIF-1 as key regulator and therapeutic target. Int J Mol Sci. 2021;22:5703.
71. Courtnay R, Ngo DC, Malik N, Ververis K, Tortorella SM, Karagiannis TC. Cancer metabolism and the Warburg effect: the role of HIF-1 and PI3K. Mol Biol Rep. 2015;42:841-51.
72. Wicks EE, Semenza GL. Hypoxia-inducible factors: cancer progression and clinical translation. J Clin Invest. 2022;e159839:132.
73. Seubwai W, Kraiklang R, Wongkham C, Wongkham S. Hypoxia enhances aggressiveness of cholangiocarcinoma cells. Asian Pac J Cancer Prev 2012;13:53-8. Available from: https://journal.waocp.org/article_27145_9dfbf7f01173f0a9bed0e95ceb44fb22.pdf. [Last accessed on 9 Dec 2024].
74. Thongchot S, Yongvanit P, Loilome W, et al. High expression of HIF-1α, BNIP3 and PI3KC3: hypoxia-induced autophagy predicts cholangiocarcinoma survival and metastasis. Asian Pac J Cancer Prev. 2014;15:5873-8.
75. Chen Y, Xu X, Wang Y, et al. Hypoxia-induced SKA3 promoted cholangiocarcinoma progression and chemoresistance by enhancing fatty acid synthesis via the regulation of PAR-dependent HIF-1a deubiquitylation. J Exp Clin Cancer Res. 2023;42:265.
76. Tang J, Tang R, Gu P, Han J, Huang W, Xue F. Hsa_circ_0019054 up-regulates HIF1A through sequestering miR-340-5p to promote the tumorigenesis of intrahepatic cholangiocarcinoma. Hum Exp Toxicol. 2022;41:9603271221126494.
77. Hsu CC, Tseng LM, Lee HC. Role of mitochondrial dysfunction in cancer progression. Exp Biol Med. 2016;241:1281-95.
78. Senyilmaz D, Teleman AA. Chicken or the egg: Warburg effect and mitochondrial dysfunction. F1000Prime Rep. 2015;7:41.
79. Suwannakul N, Armartmuntree N, Thanan R, et al. Targeting fructose metabolism by glucose transporter 5 regulation in human cholangiocarcinoma. Genes Dis. 2022;9:1727-41.
80. Xu L, Li Y, Zhou L, et al. SIRT3 elicited an anti-Warburg effect through HIF1α/PDK1/PDHA1 to inhibit cholangiocarcinoma tumorigenesis. Cancer Med. 2019;8:2380-91.
81. Thonsri U, Wongkham S, Wongkham C, et al. High glucose-ROS conditions enhance the progression in cholangiocarcinoma via upregulation of MAN2A2 and CHD8. Cancer Sci. 2021;112:254-64.
82. Xu L, Wang L, Zhou L, et al. The SIRT2/cMYC pathway inhibits peroxidation-related apoptosis in cholangiocarcinoma through metabolic reprogramming. Neoplasia. 2019;21:429-41.
83. Sun L, Song L, Wan Q, et al. cMyc-mediated activation of serine biosynthesis pathway is critical for cancer progression under nutrient deprivation conditions. Cell Res. 2015;25:429-44.
84. Colyn L, Bárcena-Varela M, Álvarez-Sola G, et al. Dual targeting of G9a and DNA methyltransferase-1 for the treatment of experimental cholangiocarcinoma. Hepatology. 2021;73:2380-96.
85. Ferrer CM, Sodi VL, Reginato MJ. O-GlcNAcylation in cancer biology: linking metabolism and signaling. J Mol Biol. 2016;428:3282-94.
86. Minh G, Esquea EM, Young RG, Huang J, Reginato MJ. On a sugar high: role of O-GlcNAcylation in cancer. J Biol Chem. 2023;299:105344.
87. Phoomak C, Silsirivanit A, Wongkham C, et al. Overexpression of O-GlcNAc-transferase associates with aggressiveness of mass-forming cholangiocarcinoma. Asian Pac J Cancer Prev 2012;13:101-5. Available from: https://journal.waocp.org/article_27153_c1ed4aa079225da4d304049e5bfd1939.pdf. [Last accessed on 9 Dec 2024].
88. Phoomak C, Silsirivanit A, Park D, et al. O-GlcNAcylation mediates metastasis of cholangiocarcinoma through FOXO3 and MAN1A1. Oncogene. 2018;37:5648-65.
89. Phoomak C, Park D, Silsirivanit A, et al. O-GlcNAc-induced nuclear translocation of hnRNP-K is associated with progression and metastasis of cholangiocarcinoma. Mol Oncol. 2019;13:338-57.
90. Khawkhiaw K, Chomphoo S, Kunprom W, et al. Involvement of interleukin-1β in high glucose-activated proliferation of cholangiocarcinoma. Transl Gastroenterol Hepatol. 2024;9:36.
91. Kongpetch S, Jusakul A, Lim JQ, et al. Lack of targetable FGFR2 fusions in endemic fluke-associated cholangiocarcinoma. JCO Glob Oncol. 2020;6:628-38.
92. Liu C, Xuan LQ, Li K, et al. Shikonin inhibits cholangiocarcinoma cell line QBC939 by regulating apoptosis, proliferation, and invasion. Cell Transplant. 2021;30:963689720979162.
93. Qian Z, Hu W, Lv Z, et al. PKM2 upregulation promotes malignancy and indicates poor prognosis for intrahepatic cholangiocarcinoma. Clin Res Hepatol Gastroenterol. 2020;44:162-73.
94. Yu W, Zeng F, Xiao Y, et al. Targeting PKM2 improves the gemcitabine sensitivity of intrahepatic cholangiocarcinoma cells via inhibiting β-catenin signaling pathway. Chem Biol Interact. 2024;387:110816.
95. Tang D, Xu L, Zhang M, et al. Metformin facilitates BG45-induced apoptosis via an anti-Warburg effect in cholangiocarcinoma cells. Oncol Rep. 2018;39:1957-65.
96. Zhang Y, Li Q, Huang Z, et al. Targeting glucose metabolism enzymes in cancer treatment: current and emerging strategies. Cancers. 2022;14:4568.
97. Molenaar RJ, Coelen RJS, Khurshed M, et al. Study protocol of a phase IB/II clinical trial of metformin and chloroquine in patients with IDH1-mutated or IDH2-mutated solid tumours. BMJ Open. 2017;7:e014961.
98. Khurshed M, Molenaar RJ, van Linde ME, et al. A phase Ib clinical trial of metformin and chloroquine in patients with IDH1-mutated solid tumors. Cancers. 2021;13:2474.
99. Mohan A, Griffith KA, Wuchu F, et al. Devimistat in combination with gemcitabine and cisplatin in biliary tract cancer: preclinical evaluation and phase Ib multicenter clinical trial (BilT-04). Clin Cancer Res. 2023;29:2394-400.
100. Sahai V, Griffith KA, Zhen DB, et al. Phase 1b/2 results of a multicenter, randomized phase 1b/2 study of gemcitabine and cisplatin +/- devimistat as first-line therapy for patients with advanced biliary tract cancer (BilT-04). JCO. 2024;42:4116.
101. Tsang ES, O'kane GM, Knox JJ, Chen EX. A phase II study of olaparib and durvalumab in patients with IDH-mutated cholangiocarcinoma. JCO. 2023;41:4099.
Cite This Article
How to Cite
Download Citation
Export Citation File:
Type of Import
Tips on Downloading Citation
Citation Manager File Format
Type of Import
Direct Import: When the Direct Import option is selected (the default state), a dialogue box will give you the option to Save or Open the downloaded citation data. Choosing Open will either launch your citation manager or give you a choice of applications with which to use the metadata. The Save option saves the file locally for later use.
Indirect Import: When the Indirect Import option is selected, the metadata is displayed and may be copied and pasted as needed.
About This Article
Special Issue
Copyright
Data & Comments
Data
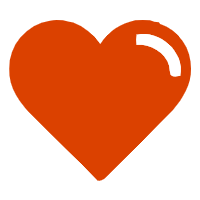
Comments
Comments must be written in English. Spam, offensive content, impersonation, and private information will not be permitted. If any comment is reported and identified as inappropriate content by OAE staff, the comment will be removed without notice. If you have any queries or need any help, please contact us at [email protected].