Construction and catalytic applications of advanced ceramic-supported single atoms
Abstract
In the domain of novel catalyst design and application, single-atom catalysts (SACs) have attracted widespread interest due to their ability to provide high catalytic activity and maximize the utilization of active sites. Various support materials capable of effectively anchoring single metal atoms have been reported, among which ceramic materials have received notable attention due to their distinctive versatility. This work primarily aims to elucidate the unique role of typical ceramic carriers in anchoring, isolating metal atoms, and participating in catalytic reactions. Here, we will clarify the interaction between metal atoms and ceramic carriers to explain the stabilization of atomic metal sites and the rational adjustment of individual atomic geometry and electronic structures. Furthermore, a comprehensive summary of recent research progress in SACs, with particular emphasis on advancements in preventing the migration or aggregation of isolated metal atoms, has also been stated. Regarding applications, we review the utilization of ceramic-supported SACs in electrocatalysis, photocatalysis, and other catalytic reactions. Finally, we discuss the challenges and prospects of ceramic-supported SACs in this field.
Keywords
INTRODUCTION
The world is currently facing critical issues of depleting non-renewable energy sources and escalating environmental pollution, which have become urgent problems requiring immediate attention from human society[1-3]. Transforming the pollutants generated by fossil energy into valuable commodities, such as carbon (C) dioxide reduction and nitrogen (N) reduction, offers an ideal solution pathway[4,5]. Additionally, the pursuit of clean renewable energy sources, such as electrocatalytic or photocatalytic water splitting for hydrogen production, is of paramount importance[6]. These chemical reactions have become focal points of research. Generally, appropriate catalysts are required in these conversion processes to enhance reaction rates. However, improving the catalytic performance of catalysts still poses significant challenges.
Critical to the catalytic performance of a catalyst is the consideration of factors such as size, corner positioning, and edge placement, all of which collectively determine its high specific surface area[7-10]. The size of the catalyst stands out as one of the pivotal factors influencing its overall performance. Catalytic reactions typically occur predominantly at the catalyst surface, with internal atoms playing a negligible role[11-13]. From this perspective, reducing nanoparticles to singular atoms proves to be a highly effective strategy, amplifying surface area and triggering quantum size effects[14]. As the size of nanoparticles diminishes, distinct physicochemical properties emerge, characterized by a notable increase in the count of exposed surface atoms, alongside alterations in surface atomic and electronic structures, as well as surface defects. Moreover, due to advantageous geometric and electronic effects, this increase significantly enhances the density of active sites, correspondingly enhancing intrinsic activity, an essential aspect in achieving unconventional catalytic performance[15-18]. In this regard, Qiao et al. pioneered the fabrication of the first successful Pt single-atom catalyst and introduced the concept of single-atom catalysts” (SACs), defined as supported metal catalysts housing exclusively isolated single metal active sites on the surface[19]. Consequently, owing to their maximal atomic utilization efficiency, unsaturated active sites, and clear reaction mechanisms, SACs have emerged as one of the leading catalyst systems to date[20]. However, despite the dispersion method of single atoms (SAs) theoretically enabling 100% atomic utilization as nanoparticle size diminishes, surface energy escalates significantly, leading to metal atom aggregation, as shown in Figure 1A and B[21,22]. Therefore, the judicious selection of suitable support materials to anchor singly dispersed metal atoms has become indispensable in stabilizing these metal atoms and advancing single-atom catalysis[23].
Currently, various support materials required for SACs have garnered widespread research interest[24]. Due to the high surface-to-volume ratio of single metal atoms, they can strongly interact with oxides[25-27], carbon-based materials[28-30], ceramics[31], and metal-organic frameworks (MOFs)[32], facilitating effective dispersion of metal atoms and preventing agglomeration phenomena. Such intense interactions may lead to dynamic charge transfer at the interface, thereby influencing the catalytic performance of SACs. Although porous MOFs with high surface areas are considered promising support materials for anchoring SACs, the structural instability of the pristine MOFs limits their application in electrochemical applications[33]. Compared with other materials, ceramic materials are more attractive in catalytic applications due to their higher stability at high temperatures[34]. Ceramic materials also possess specific and variable properties. For example, the abundant defect sites (steps, vacancies) and -OH groups on the surface can serve as anchor sites for single metal atoms[25]. Furthermore, through chemical modification, metal-support interactions can be established, and this synergistic effect greatly affects catalytic performance. The robustness of oxides at high temperatures is also an important factor in enhancing the mechanical and thermal stability of SACs. Therefore, among various supported SACs, ceramic-supported SACs are particularly interesting. Ceramic-supported SACs are particularly intriguing among the numerous supported SACs due to their diverse properties in various ceramic materials, including high-temperature stability and the presence of abundant coordinatively unsaturated sites on the surface[35,36].
This paper presents a comprehensive overview of research on SACs supported by ceramics in the field of energy conversion. The performance of SACs is intricately linked to their geometric and electronic structures. Therefore, we have selected representative and recent cases, including oxides, perovskites, spinels, MXenes, and other ceramic-based SACs, and delved deeply into the structure-property relationships among them. Additionally, we emphasize the analysis of interactions between single metal atoms and ceramic supports. Furthermore, we discuss several pivotal catalytic reactions (oxygen evolution (OER), hydrogen evolution (HER), oxygen reduction (ORR), carbon dioxide reduction (CO2RR), nitrogen reduction (NRR), and carbon dioxide hydrogenation reactions (CO2HR)), alongside pertinent theoretical investigations and reaction mechanisms, as detailed in Figure 2. We aim to provide valuable insights for exploring potential ceramics for SACs. The final section provides a concise summary and outlook on the development of ceramic-supported SACs, elucidating potential challenges and outlining future research directions.
DIFFERENT TYPES OF CERAMIC-SUPPORTED SACs
In the field of energy conversion catalysts, various types of SACs exhibit unique characteristics. Through careful selection of supports, we can manipulate the spatial configuration and coordination environment of single metal atoms in different carriers, thereby enhancing catalytic performance. This section focuses on the catalytic activity of single metal atoms on various ceramic supports. Firstly, we focus on oxides such as Al2O3, ZrO2, and CeO2, as they are among the most widely used supports. Subsequently, we investigate spinel, characterized by exceptional chemical and thermal stability, along with tunable pore structures and surface active sites, rendering them ideal catalytic carriers capable of facilitating efficient reaction environments across diverse conditions. Following that, we present perovskites as structurally stable materials proficient in securely anchoring SACs loaded on their surfaces, thus averting aggregation and deactivation, consequently augmenting catalyst stability and recyclability. Finally, we discuss the application prospects of MXene as a novel class of two-dimensional materials. MXene has abundant surface functional groups and active sites, and by loading SACs on its surface, active metal atoms can be effectively dispersed and anchored on the carrier, thereby improving the stability and activity of the catalyst. SACs demonstrate enormous potential in the field of catalysis, being widely applied not only in electrocatalysis[37] and photocatalysis[38], but also garnering extensive attention in the battery domain[39,40].
Typical oxide carriers
Oxide supports emerge as an ideal choice for metal catalysts due to their high surface area and diverse defect types, such as oxygen vacancies (Ov), metal vacancies (Mv), edges, and steps. These defects are considered ideal anchoring sites for single-atom metals since they can be tailored through the crystal structure and synthesis conditions of oxides, thereby influencing the adsorption and stability of single-atom metals[41-44]. In the absence of reducing conditions, according to the Toppolin electron rule, positively charged single-atom metals can stably adsorb onto surface bonds M-O(H) with a slight negative charge[45]. Oxides such as CeO2, TiO2, Al2O3, SiO2, and ZrO2 exhibit advantages in immobilizing individual metal atoms, not only stabilizing them but also participating in reactions, where surface cations of oxide supports can be replaced by metal atoms, interacting with adjacent oxygen anions to further enhance stability. These characteristics render oxide supports highly valuable in supporting SACs, enhancing catalyst stability and activity, thus playing a pivotal role in the catalysis domain.
CeO2-supported SACs
CeO2 stands as a pivotal 4f oxide; thanks to continuous optimization of its manufacturing process, it increasingly meets the requirements for large-scale production. It has garnered extensive attention and research across a range of applications including electrocatalysis, biosciences, and electronic ceramics[46-48]. Due to its unique properties, it is widely used as a support material for SACs. Known for its high density of vacancies and reversible oxygen storage process, CeO2 enables the modulation of local coordination environments and electronic states of metal atomic sites in SACs through redox thermal activation. During oxidative calcination, the metal nanocrystalline structure on the CeO2 support can form covalent M-O bonds, facilitating the dispersion of metal atoms at the atomic scale[49,50]. Notably, CeO2 has also garnered attention for its strong metal-support interaction (SMSI) with noble metals, enabling the stable existence of noble metals in highly dispersed forms[51,52]. Strategies such as low metal loading, high surface area nanoscale carriers, or a combination of both are commonly employed to prevent the aggregation of active phases in noble metal SACs. Additionally, nanoscale structures can significantly alter the chemical properties of
TiO2-supported SACs
Within natural environments, TiO2 manifests in three principal crystalline forms: rutile, anatase, and brookite. Rutile stands as the most stable phase, while anatase and brookite may transition to rutile under thermal treatments. Due to its abundant raw materials and cost-effective production, TiO2 has become a prominent material in various emerging research domains, including energy conversion and storage, environmental remediation, and optoelectronics[59]. Notably, TiO2 in rutile and anatase phases finds extensive application as catalyst support due to its indispensable roles in catalytic reactions[60]. Throughout catalysis, the migration of metal atoms facilitates the emergence of Ov and Ti3+ sites on TiO2 surfaces, thereby fostering the development of active metal-TiO2 interfaces[61-63]. Wan et al. proposed a methodology to stabilize single-atom sites through the introduction of defects on the support surface, resulting in the fabrication of nanosheets with exceptional catalytic performance by supporting single-atom Au on defective TiO2, as shown in Figure 4A and B[64]. These defects serve to diminish energy barriers and mitigate competitive adsorption among isolated Au atomic sites, thus amplifying catalytic efficiency. And the elements are evenly distributed [Figure 4C]. Yang et al. introduced a novel strategy to stabilize isolated gold atoms via ultraviolet (UV) irradiation of titanium dioxide supports, achieving stabilization in ethanol[65]. This method facilitates the facile dissociation of water molecules on gold-oxygen-titanium dioxide sites, ensuring that even nanoscale particles formed at elevated gold loadings do not augment the activity of gold-bound atoms on titanium dioxide. The integrity of the catalytic activity is upheld by eliminating excess gold through sodium cyanide leaching, thereby ensuring the steadfast binding of atomically dispersed gold to titanium dioxide. These investigations underscore the potential of defect engineering in optimizing catalyst performance, thereby furnishing novel pathways for the design of efficacious catalysts.
Figure 4. (A) Schematic illustration of synthesis procedure and the results of Au-SA/Def-TiO2. (B) HAADF-STEM image, single atomic Au sites highlighted by red circles. (C) Elemental mappings show the distribution of Ti (blue) and Au (red)[64].
Al2O3-supported SACs
Al2O3 plays a pivotal role in industrial catalysis. Thanks to advanced production technology and abundant raw materials, it is cost-effective and widely used in refractory and electronic components[66-69], particularly as a carrier for metal catalysts. Despite the lack of covalent metal-support bonds, Al2O3 can stabilize individual metal atoms on its surface through metal-oxygen/hydroxyl interactions[70,71]. This stability allows the metal species to connect with the porous nanostructure supported by Al2O3, maintaining the dispersion of metals[72]. This strategy not only aids in enhancing the activity and stability of catalysts but may also influence surface properties such as surface charge transfer, changes in metal structure, and regulation of molecular adsorption, thereby affecting catalyst performance[73-75]. However, due to the relatively weak metal-support interaction of Al2O3 for stabilizing isolated active metal atoms, further modification of the support cations may be necessary to achieve better performance. Mesoporous alumina presents a feasible option due to its increased defect sites, which can enhance the interaction between metal and support, further improving catalytic performance[76,77]. Shang et al. successfully prepared atomically dispersed platinum nanosheets (Pt/dp-Al2O3) on mesoporous alumina support using a combination of wetness and thermal decomposition methods[78], as shown in Figure 5A. These nanosheets exhibited high-density active sites, strong anchoring effects and interfacial synergy between the support and platinum atoms. Zhang et al. also described a highly stable, atomically dispersed Pt catalyst supported on mesoporous Al2O3[79]. The catalyst was synthesized using an enhanced sol-gel solvent evaporation self-assembly approach, followed by calcination in air and then reduction with H2, as shown in Figure 5B. Owing to the stability of the coordinatively unsaturated tetrahedral Al3+ centers, Pt atoms were securely immobilized on the inner surface of mesoporous Al2O3. This catalyst retained its catalytic activity and structural integrity throughout a series of prolonged, rigorous reactions.
SiO2-supported SACs
SiO2 has abundant reserves on Earth and has become an indispensable material in various fields such as catalysis and biotechnology[80-83]. Similar to Al2O3, SiO2 is also an irreducible oxide. It has been reported that various metals such as Pt[84] can be atomically dispersed on SiO2 and utilized in catalytic reactions such as catalytic cracking, epoxidation, methanol carbonylation, and C-H bond functionalization[85-88]. However, under reaction conditions, metals initially deposited or grafted onto the SiO2 surface often tend to aggregate. To address this issue, several strategies have been developed, such as incorporating alkali ions (Na+, K+) or ligands containing nitrogen during synthesis[89,90]. Zhai et al. found that adding metals to silica surfaces promoted by alkali ions has a similar effect to adding metals to ceria[91]; that is, the surface oxygen associated with metals and alkali ions becomes reducible at low temperatures, and the presence of alkalis during heat treatment inhibits the growth of metal particles. This indicates that Na-modified silica-supported Pt atoms remain stable after heat treatment. Nevertheless, a more effective approach is to confine metal species within the supporting lattice or even immobilize them within porous solid materials. For example, hydrated metal aluminosilicate compounds, namely zeolites, can form three-dimensional channel structures. Zeolites greatly enhance the thermal stability of isolated metal atoms, as the framework cavities within zeolites act as traps preventing further aggregation via the Brownian motion of metal atoms. Transition metals (TMs) can be easily loaded into zeolites via ion exchange methods, with framework oxygens in the ring pockets stabilizing the metal atoms. Additionally, depending on the pore size and shape, molecular sieves are commonly employed for molecular separations. Thus, using zeolites as catalyst supports can be expected to enhance the activity and/or selectivity of the catalyst[92]. Li et al. proposed a novel strategy involving the grafting of separated and defective CeOx nanoclusters onto high-surface-area SiO2, wherein each nanocluster hosts an average of one platinum atom[93]. This involved the grafting of separated and defective CeOx nanoclusters onto high-surface-area SiO2, wherein each nanocluster hosts an average of one platinum atom. They observed that Pt atoms remained dispersed even under high-temperature oxidation and reduction conditions, resulting in a significant enhancement in the CO oxidation activity of the catalyst. This approach ensures the mobility of Pt atoms while constraining them within their respective nanoclusters. The utilization of functional nanoclusters to confine metal atom dispersion and concurrently enhance reactivity represents a prevalent strategy, thereby bridging the gap between SACs and practical applications.
ZrO2-supported SACs
ZrO2 commonly exists in multiple crystal forms, each exhibiting distinct physical properties[94]. ZrO2 ceramics are renowned for their excellent thermal and chemical stability and have been widely used in various fields, such as solid oxide fuel cells, biomedical sectors, and high-temperature structural ceramics[95-97]. Notably, in the monoclinic crystal system of ZrO2, there is a higher concentration of hydroxyl groups, facilitating the binding of individual metal atoms and thus presenting significant advantages in the field of single-atom catalysis[98,99]. The surface of zirconia possesses abundant active sites capable of securely anchoring individual metal atoms, thereby providing reliable active centers for catalytic reactions[100,101]. Additionally, the electronic structure of zirconia can be modulated through the control of surface oxidation states and lattice defects, optimizing the catalytic performance and selectivity of SACs. The zirconia surface exhibits excellent resistance to poisoning, reducing the risk of catalyst impairment by external impurities and toxic substances, thus enhancing its long-term stability and catalytic activity[102,103]. Zirconia SACs have demonstrated broad application prospects in fields such as redox reactions and hydrocarbon conversion[104]. Du et al. successfully immobilized Nb SAs onto a ZrO2 substrate (Nb1-ZrO2)[105]. Their study revealed that the formation of Nb1-Zr dual sites on Nb1-ZrO2 enhanced the adsorption and activation of NO2, optimized the adsorption of key ammonia (NH3) intermediates, and decreased the reaction energy barrier, leading to an improvement in the activity of NO2RR. Choudhary et al. utilized an easy co-precipitation method to produce Co/ZrO2 SACs[106]. Their study revealed that cobalt-doped ZrO2 acts as a SAC, with each Co2+ ion facilitating CO2 fixation. Characterization of the Co/ZrO2 catalyst through EXAFS and STEM affirmed the existence of isolated Co2+ species on the ZrO2 support.
Spinel-based carriers
Spinel is a category of materials possessing a unique crystal structure, with a general chemical formula of AB2O4, where A and B represent two different metal ions, and X denotes oxygen ions. Spinel exhibits remarkable activity and high stability owing to its typical crystal structure[107], and holds important promise for applications in cutting-edge domains, especially in battery technology and electromagnetic wave absorption materials[108-110]. In AB2O4, TM cations occupy octahedral sites (TMoct) consisting of six oxygen anions and tetrahedral sites (TMtd) composed of four oxygen anions, with TMoct typically serving as the primary active sites for OER, exposed near the surface of AB2O4[111,112]. In a typical inverse spinel structure, all A2+ cations and half of the B3+ cations occupy TMoct, facilitating electron transitions between A2+ and B3+, achieving higher conductivity, and providing more surface redox-active centers from A2+ and B3+ with different oxidation states, thereby accelerating reaction kinetics[113,114]. Due to the typical lattice structure of AB2O4, oxygen anions are shared by one TMtd and three TMoct, reducing electron polarization from oxygen anions to TM cations between neighboring cations, decreasing overlap between metal-d and oxygen-p orbitals, thereby suppressing the covalency of TM-O bonds[115,116]. Therefore, spinel, as a crystal material with unique structure and properties, holds broad application prospects in energy conversion and storage applications[117]. Its low cost, accessibility, high activity, and stable catalytic properties provide important support for the design and development of efficient and stable SACs. Shan et al. designed a catalyst by placing iridium SAs on the cationic sites of cobalt oxide spinel, synthesizing Ir0.06Co2.94O4, as shown in Figure 6A[118]. This catalyst exhibited twice the oxygen evolution performance of cobalt oxide under acidic conditions. Due to the strong interaction between iridium and the cobalt oxide support, the corrosion resistance and oxidation potential of Ir0.06Co2.94O4 catalyst significantly improved under acidic conditions. This work eliminates the “close-packing” limitation of noble metals and provides promising opportunities for creating analogs with diverse catalytic applications requiring specific topological structures. Wang et al. introduced an innovative approach involving single-atom bismuth (Bi) doping, resulting in the synthesis of single-atom dual-doped Co3O4 (Bi-Co3O4) through a combination of electrodeposition and calcination techniques, as shown in Figure 6B[119]. Experimental characterization alongside theoretical computations unveiled that single Bi atoms replaced cobalt ions at the octahedral positions within the Co3O4 structure, thereby facilitating the generation of active hydroxyl groups at nearby tetrahedral Co sites. This study highlights the promising avenue of employing single-atom doping strategies to enhance the electrocatalytic performance of spinel oxides.
Perovskite-based carriers
Perovskite oxides have garnered significant attention due to their outstanding high-temperature stability and pristine ABO3 crystal structure[120-122]. Perovskite materials have attracted great attention due to their excellent photoelectric properties and impressive conversion rates, particularly in the field of optoelectronic sector[123,124]. Firstly, their exceptional lattice and chemical stability ensure reliable surface support, aiding in the preservation of individual metal atom stability and the formation of stable surface active sites crucial for catalytic reactions. These sites play a pivotal role in catalysis, effectively facilitating catalytic activity[125]. Secondly, interactions between perovskite and single metal atoms can modulate the mechanisms and activity of catalytic reactions, influencing reaction selectivity and efficiency by adjusting electronic structures, and thereby optimizing catalytic performance[126]. Furthermore, perovskite possesses a diverse electronic structure capable of charge transfer and interaction, enabling controlled modulation of catalytic activity to meet the varied demands of different catalytic reactions[127]. Its high specific surface area and abundant active sites provide ample reaction sites for catalysis, while defects in the lattice and surface Ov further enhance catalytic performance[128]. Lastly, perovskite not only serves as a carrier for SACs but also, through structural and compositional adjustments, achieves multifunctional regulation of various catalytic reactions, exhibiting extensive application prospects[129-131]. Tian et al. first reported the loading of single metal atoms onto perovskite oxides, utilizing heterostructure perovskite as a unique nanostructure for SAC immobilization[132]. The authors provided a generalizable method for manufacturing highly stable Au monoatomic catalysts with tunable catalytic properties. The resulting Au SAC not only exhibits resistance to sintering at 700 °C but also demonstrates high catalytic activity under reaction conditions, displaying significant self-activation activity. This discovery has significantly spurred the exploration of other perovskite-loaded metal SACs, thereby advancing their catalytic applications. Shin et al. introduced a versatile method for fabricating SACs on metal, metal oxide, and perovskite nanosheet scaffolds[133]. They achieved an impressive metal loading of up to 3.94 wt% by utilizing nitrogen-doped graphene as a sacrificial template to confine SAs spatially. Initially, the research team anchored the precursor of the target support material onto the stable sacrificial template hosting SAs. Subsequent thermal treatment facilitated the transfer of SAs onto the support material while eliminating the graphene layer, as shown in Figure 7. Remarkably, Pt SAs on the oxide carrier displayed minimal aggregation during annealing at temperatures exceeding 275 °C for over 10,000 min, highlighting their outstanding thermal stability. Therefore, perovskites exhibit enormous potential in stabilizing SAs at high temperatures.
Figure 7. Schematic illustration for the synthesis of Pt single-atoms stabilized on various inorganic (metal oxide, perovskite, and metallic) nanosheets via the N-doped graphene sacrificial templating route[133].
MXene-based carriers
In recent years, researchers have drawn attention to the exceptional capability of two-dimensional materials to effectively stabilize individual metal atoms[134]. The inherent thin-layered structure of these materials provides a larger surface area and numerous anchoring points, facilitating the synthesis of SACs with high loadings. Among these materials, MXenes have emerged as a novel and innovative class of two-dimensional materials characterized by their surface terminal groups[135], offering the potential to serve as support for anchoring atomic metal species. They have garnered widespread interest across various disciplines due to their unique surface chemistry, graphene-like morphology, metallic conductivity, high hydrophilicity, excellent mechanical properties, and redox capabilities, leading to rapid advancements and applications in fields such as electrochemistry, catalysis, and photothermal conversion[136-139]. Notably, MXene sheets typically exhibit spontaneously formed Mv during synthesis, offering advantages for the introduction and modification of heteroatoms[140]. What sets MXenes apart from other two-dimensional materials is their exceptional tunability in terms of electronic bandgaps, conductivity, and charge transfer rates. The monolayer structure of MXenes, characterized by hexagonal symmetry akin to graphene, adopts an interlayer structure with X as the central layer and M as the edge layer. It is coordinated by six N or C atoms directly with M and surrounded by a series of surface terminal functional groups and metal coordination. Some MXenes are more prone to decompose into monolayer structures, which can be achieved by adjusting the elemental ratios of M and X to tailor the material's structure and properties, or by modifying terminal functional groups to enhance material characteristics such as hydrophilicity/hydrophobicity, conductivity, and stability. The controlled formation of defect sites during synthesis renders MXenes an ideal support material for the fabrication of SACs[141-144]. Furthermore, through the modulation of the central metal type and substitution of terminal groups with other functional groups, the electronic bandgap width of MXenes can be readily adjusted, a feat challenging to achieve with other two-dimensional materials such as graphene[145,146]. Ramalingam et al. demonstrated the synthesis of isolated ruthenium SAs (RuSA) and Ti3C2Tx MXene supported by N and sulfur (S) heteroatom-doped carriers, as shown in Figure 8A[147]. The stabilization of RuSA on the MXene carrier was facilitated through Ru-N and Ru-S bonds formed with N and S atoms, respectively. The coordinated species of N and S in RuSA-N-S-Ti3C2Tx significantly enhanced the catalytic activity of HER in acidic solutions. Additionally, Zhang et al. introduced Pt SAs (PtSA) onto monolayer Ti3C2Tx matrices using a rapid thermal shock strategy under H2 atmosphere, exploiting unconventional methods due to the formation of Ov in hydroxylated MXenes during conventional annealing in H2, as shown in Figure 8B[148]. Post-H2 annealing of monolayer Ti3C2Tx was shown to induce Ov formation under reducing atmospheres. High-angle annular dark-field scanning transmission electron microscopy (HAADF-STEM) and density functional theory (DFT) calculations confirmed that Ov formation on monolayer Ti3C2Tx could be captured through Pt-Ti bonding to trap PtSA.
CATALYTIC APPLICATION OF CERAMIC-SUPPORTED SINGLE ATOM
SACs have gained widespread application in catalytic fields such as thermal catalysis, electrocatalysis, and photocatalysis due to their unique structure, which exhibits distinct properties from nanoparticle catalysts. In this section, we will focus on the applications of SACs in various catalytic reactions, including OER, HER, ORR, CO2RR, NRR, and CO2HR, as shown in Table 1. We will conduct detailed investigations into the mechanisms and structure-performance relationships of these reactions from both experimental and theoretical perspectives.
Catalytic application of ceramic-based SACs
Catalyst | Atomic species | Carrier type | Electrolytes | Application | Refs. |
PtSA-Mn3O4 | Pt | Spinel | 1 M KOH | HER | [154] |
Ru-ZrO2-x/C | Ru | Oxide | 0.5 M H2SO4 | HER | [155] |
Ir@Sr-p-TiO2 NWs | Sr | Oxide | 0.5 M H2SO4 | OER | [162] |
Ir1/Ni1.6Mn1.4O4 | Ir | Spinel | 1 M KOH | OER | [163] |
Fe@ZrO2/NC | Fe | Oxide | 0.1 M KOH | ORR | [168] |
Fe-N-C/Nb4C3Tx | Fe | MXene | 0.1 M KOH | ORR | [169] |
FeSAs+NPsCeSAs+Fe-ONPs/NC | Fe | Oxide | 0.1 M KOH | ORR | [170] |
Ru-NS-Ti3C2O2 | Ru | MXene | - | CO2RR | [171] |
CuSiOx | Cu | Oxide | 0.1 M KHCO3 | CO2RR | [172] |
GaSA/a-TiO2 | Ga | Oxide | 0.1 M Na2SO4 | NRR | [175] |
LaFeO-Ru | Ru | Perovskite | 0.1 M K2SO4 | NRR | [176] |
PtSA/TiO2 | Pt | Oxide | 0.1 M Na2SO4 | HER | [186] |
Cu/TiO2 | Cu | Oxide | 25% methanol | HER | [187] |
Co-Ti3C2Tx | Co | Oxide | H2O/acetonitrile | CO2RR | [188] |
Co-N-C@BiFeO3 | Co | Perovskite | - | CO2RR | [189] |
Au/Al2O3 | Au | Oxide | H2O | CO2RR | [192] |
Cu/ZrO2 | Cu | Oxide | - | CO2HR | [196] |
ZrO2/Cu | Cu | Oxide | - | CO2HR | [197] |
Cu/Mo2CTx | Cu | MXene | - | CO2HR | [198] |
Electrocatalytic application
With the rapid increase in the production of sustainable and renewable energy sources, there is a growing emphasis on electrochemical performance[149,150]. However, limitations still exist in the catalytic performance required for various electrocatalytic systems. Therefore, efforts are being made to find more rational approaches, such as controlling the size, shape, composition, and structure of electrocatalysts, to enhance catalytic efficiency[151,152]. In particular, research on SACs has sparked emerging interest in electrocatalysis due to their high catalytic activity, stability, selectivity, and 100% atom utilization. Ceramic-supported SACs exhibit great potential in electrocatalytic HER, OER, ORR, CO2RR, and NRR, as shown in Table 1.
HER
Hydrogen is considered an ideal clean energy source, and electrocatalytic water splitting is deemed a sustainable means of production. Presently, Pt-based catalysts are recognized for their superior performance in the HER. However, the high cost associated with Pt, stemming from its limited natural abundance, poses a significant barrier to its widespread adoption. Hence, there is a pressing need to minimize Pt usage or explore alternative materials. SACs have emerged as a promising avenue, offering maximal atomic efficiency, and have, therefore, garnered significant research attention[153].
In a pioneering study, Wei et al. reported, for the first time, the synthesis of spinel-type Mn3O4-supported
Figure 9. (A) HER polarization curves of NF, Mn3O4, PtSA-Mn3O4, and Pt/C. (B) The mass activity of Pt SA-Mn3O4 and Pt/C. (C and D) Calculated Gibbs free energy for the Volmer step on the surfaces, and GH* values of hydrogen evolution at the equilibrium potential of Mn3O4, Pt (111) and PtSA-Mn3O4[154]. (E) HER polarisation curves of Pt/C, Ru/C, and Ru-ZrO2-x/C catalysts. (F) Comparisons of the overpotentials at 10 mA cm-2. (G) Mass activities of Ru/C, Pt/C, and Ru-ZrO2-x/C catalysts and the hydrogen adsorption energies on the Ru(0001), Pt(111), and Ru-ZrO2-x surfaces obtained from DFT calculations[155].
Kim et al. proposed a novel approach to SACs by tightly integrating stable metal oxide nanoparticles, resulting in a significant enhancement in the electrochemical activity and stability of catalysts for HER[155]. In their study, Ru-ZrO2-x/C exhibited a remarkable improvement in HER activity, particularly at high current densities, with the smallest overpotential observed when the current density exceeded
OER
The process of water electrolysis encompasses both the HER and OER. The sluggish, multi-step proton-coupled electron transfer process of OER markedly diminishes the overall electrolysis rate[157-159]. Hence, there is a concerted effort among researchers to explore efficient OER catalysts. IrO2/RuO2 stands as a relatively advanced catalyst; however, its high cost is a barrier to further advancement[160,161]. Consequently, the quest for low-cost, high-efficiency, and stable OER catalysts has emerged as a focal point of research.
Zhu et al. have demonstrated the remarkable catalytic performance of plasma-treated TiO2 nanowires (Ir@Sr-p-TiO2 NWs) in the OER, achieving a substantial reduction in overpotential to 250 mV at a current density of 10 mA cm-2 [Figure 10A][162]. The inset in Figure 10A shows that the mass activity of Ir@Sr-p-TiO2 NWs at a potential of 1.53 V is 1364.7 A gIr-1, which is much higher than the mass activity of c-IrO2
ORR
The ORR occurring at the air cathode plays a critical role in energy conversion and storage technologies, such as rechargeable zinc-air batteries (ZABs). As a fundamental component of ZABs, the cathodic ORR exhibits slow reaction kinetics due to the high-energy barriers associated with O2 adsorption, O-O bond cleavage, and the intricate reaction mechanisms involved[164-166]. Hence, the development of highly efficient catalysts is imperative. SACs have garnered extensive research interest owing to their ability to significantly enhance the number of active sites. Notably, their well-defined active centers elucidate the interactions between individual metal atoms and the supporting materials more clearly[167].
Zhang et al. proposed a ligand-assisted strategy to synthesize single-atom Fe-N-C derived from
Figure 11. (A) LSV curves of Pt/C. (B and C) Differential charge density analysis of SA Fe/NC and SA Fe@ZrO2/NC models. Light blue area represents charge density decrease, and yellow area denotes charge density increase[168]. (D) LSV curves of the catalysts for the ORR. (E) ORR curves of the Fe-N-C/Nb4C3Tx (2:1) before and after sweeping for 2,000 cycles. (F) Chronoamperometric curves of the catalyst based on the Fe-N-C/Nb4C3Tx (2:1)[169]. (G) ORR polarization curves. (H) jk and TOF of catalysts to present intrinsic activity. (I) Durability results of the catalyst after 50,000 potential cycles[170].
CO2RR
To address the issue of global warming caused by CO2 emissions from fossil fuel combustion, converting CO2 into valuable chemical products such as carbon monoxide (CO), CH4, and ethanol presents a promising strategy. The selection of catalysts plays a crucial role in the selectivity of the CO2RR; thus, SACs with high catalytic activity have attracted significant attention.
Cao et al. have devised and screened 50 candidate SACs comprising Ti3C2O2 coordinated with 25 TMs in N, S, or N, N configurations (NS/NN-Ti3C2O2) for the CO2RR to produce CO[171]. Through analysis of the limiting potential for CO production and competition with the formic acid generation, CO reduction, and HER, it was found that Ru-NS-Ti3C2O2 and Co-NN-Ti3C2O2 serve as outstanding catalysts for CO2RR to generate CO. In actual electrocatalysis, Ru-NS-Ti3C2O2 exhibited a remarkable electrochemical response;
Figure 12. (A) Free energy diagram vs. SHE at different potentials on Ru-NS-Ti3C2O2. (B) The binding energy of CO2, COOH, and CO as a function of surface charge density (σ) for Ru-NS-Ti3C2O2[171]. (C) FEs of CO2RR products at different potentials. (D) Difference in limiting potentials for CO2R and HER on CuSiOx and Cu (111), and reaction free energy difference between *CO protonation and C-C coupling steps on CuSiOx and Cu (111) surfaces. (E) CuSiOx reacts at a potential of -1.6 V vs. RHE for 12 h[172].
NRR
Ammonia serves as the fundamental component for the production of the majority of fertilizers; thus, its production volume is crucial for today's food security[173]. However, the current production of NH3 relies on fossil fuel consumption, prompting the search for sustainable production methods. Electrochemical NRR boasts higher energy efficiency and is poised to enable decentralized NH3 production, ultimately potentially supporting distributed fertilizer manufacturing[174].
Zhang et al. have successfully synthesized lotus root-like amorphous TiO2 nanofibers[175]. In contrast to previously reported Vacco-engineered TiO2 nanocrystals, these fibers exhibit abundant intrinsic Ov and dangling bonds, resulting in significantly enhanced N2 activation and electron transport capabilities. To address selectivity challenges, a confinement effect was achieved through Vo, leading to the successful synthesis of relatively isolated SAs and maximizing the availability of Ga-Vo reaction sites. DFT calculations indicated favorable N2 adsorption on the catalyst surface by Ga SAs, while Vo facilitated N2 activation and reduction. This synergistic activity/selectivity design resulted in high NH3 yield (24.47 g·h-1·mg-1) and FE (48.64%) at remarkably low overpotentials [Figure 13A]. The catalyst was subjected to a long-term durability test for 24 h, and the time-series current measurement curve was stable, as shown in Figure 13B. Han et al. utilized a plasma-enhanced chemical vapor deposition technique in this investigation to immobilize TM elements onto two-dimensional conductive materials[176]. Among numerous trials, it was discovered that Ru SAs and Ru clusters supported on calcium titanate oxide demonstrated remarkable electrocatalytic capabilities (achieving NH3 production rates of up to 137.5 ± 5.8 µg h-1 mgcat-1, as depicted in Figure 13C, surpassing the previous records for Ru-based catalysts. After five consecutive experiments, the yield of LaFeO-Ru decreased by approximately 9%, from 137.3 µg h-1 mgcat-1 to 124.7 µg h-1, accompanied by a reduction in its efficiency of about 6.7%, dropping from 59.7% to 55.7% [Figure 13D]. These durability test results indicate a slight decline in the catalytic performance of LaFeO-Ru over prolonged usage, although it maintains relatively high catalytic activity and stability. This marginal performance degradation may be attributed to partial deactivation of the material surface or subtle structural alterations, warranting further investigation to comprehend its specific mechanisms.
Figure 13. (A) NH3 yields and FEs of Ga SA/a-TiO2 nanofibers at different potentials for 2 h. (B) Chronoamperometry curve of
Photocatalytic application
Single-atom photocatalysts have shown tremendous potential in the production of value-added chemicals and/or fuels, sparking considerable interest among researchers[177-179]. SAC photocatalysts refer to semiconductor substrates decorated with atomic-level metal particles. These metal atoms play the role of auxiliary catalysts on the semiconductor surface, increasing the number of active sites while receiving electrons from the process of light harvesting. Additionally, SAC photocatalysts can effectively reduce the usage of precious metals. This design offers higher catalytic efficiency and selectivity while lowering costs. As a result, SAC photocatalysts have become one of the focal points of current research, with broad prospects for applications in fields such as energy conversion and environmental remediation[180-182]. In the field of photocatalysis research, photocatalytic hydrogen production is a focal point of investigation[183,184]. As one of the most promising photocatalysts, TiO2 has the advantages of innocuity, abundant availability and good stability[185]. Kerketta et al. have confirmed that Grätzel-type mesoporous TiO2 layers serve as ideal carriers for Pt SAs, facilitating efficient photocatalytic H2 generation[186]. By optimizing the geometric structure and Pt SA loading layer, the research team achieved a high photocatalytic H2 production rate of approximately 2,900 mL h-1 (under conditions of wavelength λ = 365 nm and light intensity
Figure 14. (A) The annealing temperature (using 14 μm layer thickness and 0.05 mM H2PtCl6 concentration in each case). (B) TiO2 layer thickness and H2PtCl6 concentration (using annealing temperature of 550 °C in each case)[186]. (C) Rate of photocatalytic H2 generation of M/TiO2 (0.75 wt%) for each type of co-catalyst. (D) Cyclic measurements of photocatalytic H2 generation of Cu/TiO2 (0.75 wt%), showing the stable activity[187].
Converting carbon dioxide into valuable products via photocatalysis offers an eco-friendly solution to mitigate the greenhouse effect. Chen et al. reported an efficient photocatalytic system using two-dimensional Ti3C2Tx-MXene nanosheets as carriers, where individual cobalt atoms are anchored as active sites for CO2 reduction under visible light[188]. In this system, Ti3C2Tx nanosheets serve as bridges connecting the visible light absorber with cobalt active sites. Cobalt atoms form strong metal-oxygen/carbon bonds with Ti3C2Tx, resulting in the formation of the Co-Ti3C2Tx integrated structure. The results show a CO production rate of up to 6.06 mmol h-1 g-1, indicating significantly enhanced CO2 photocatalytic performance compared to previous MXene-based catalysts. Theoretical calculations suggest that the synergistic interaction between isolated cobalt atoms and Ti3C2Tx effectively promotes CO generation.
Other catalytic applications
Presently, the conversion of carbon dioxide into liquid fuels stands as a viable strategy to combat contemporary energy and environmental issues. Recent years have witnessed significant advancements in carbon dioxide hydrogenation catalysts, particularly in the realm of SACs supported by oxides. Among these catalysts, the Fischer-Tropsch synthesis serves as a pivotal reaction process, directly impacting the coupling degree between carbon and the desired products[100,195].
Zhao et al. have presented an intriguing study unveiling a novel copper-based catalyst characterized by distinctive isolated active copper sites, exhibiting exceptional performance in CO hydrogenation[196]. With the CAZ-1 catalyst, only methanol was discerned, devoid of any accompanying byproducts. Notably, this catalyst showcased remarkable selectivity, prioritizing methanol production with an impressive TOFCu value reaching 1.37 h-1. Their investigation reveals that the single-atom Cu-Zr catalyst predominantly facilitates methanol synthesis at 180 °C, whereas small copper clusters or nanoparticles with Cu-Cu structural motifs induce CO byproduct formation. Moreover, during the catalytic process, copper undergoes a transition from Cu-Cu structures to Cu1-O3 units, forming a catalyst surface with a quasi-planar structure, thereby expediting the hydrogenation of carbon dioxide. This seminal discovery not only underscores the pivotal roles of high activity, independent copper sites, and discernible structural motifs in the thermal catalytic hydrogenation of CO2 but also presents novel conceptual frameworks for the application of SACs in energy conversion. Wu et al. synthesized adjustable ratio ZrO2/Cu inverse catalysts using the oxalic acid
CONCLUSIONS
This work provides a comprehensive review of the latest advancements in ceramic-supported SACs for catalysis. By thoroughly examining representative cases spanning oxides, perovskites, spinel, MXene, and other ceramic-based SACs, it reveals the intricate relationship between geometric and electronic structures of SACs and their corresponding performance. Oxides provide robust support for SACs due to their high thermal stability, chemical inertness, and high specific surface area, effectively stabilizing SA active centers. However, their poor conductivity and lack of redox activity limit their application. Spinel has various combinations and structures and excellent thermal stability, but its complex synthesis and high cost pose challenges to its widespread application. Perovskite exhibits structural flexibility, good oxygen migration, and conductivity. However, it is often prone to aggregation at high temperatures, and its high cost further limits its actual development. In contrast, MXene, as a novel 2D material, has high conductivity and abundant surface functional groups, although its complex synthesis process and long-term stability still require further investigation. Overall, ceramic supports play a crucial role in anchoring and isolating metal atoms while actively participating in catalytic reactions. By elucidating the complex interactions between metal atoms and ceramic carriers, insights are gained into the stability of atomic metal sites and the rational manipulation of individual atomic geometries and electronic structures. Additionally, this work systematically summarizes recent research progress in SACs, with a particular focus on advancements aimed at mitigating the migration or aggregation of isolated metal atoms. The review explores the applications of SACs in several pivotal catalytic reactions. Through detailed analysis of relevant theoretical research and reaction mechanisms, robust theoretical support is provided for the practical deployment of SACs in catalysis. Furthermore, this work highlights the advantages of SACs in the field of catalysis, including their exceptional catalytic performance, robust stability, and tunable electronic structure.
The research on SACs in the realm of catalysis continues to hold significant promise. As our understanding deepens, we anticipate the emergence of more innovative combinations of ceramic supports and metal atoms, thereby augmenting the catalytic performance and stability of SACs. The following points should be considered in future research.
(1) Research on the stability of metal atoms represents a critical avenue of investigation, with a central focus on preventing the migration or aggregation of isolated metal atoms. While several methods exist to achieve this objective, challenges persist. Consequently, future research will concentrate on developing more effective and stable anchoring strategies. These strategies will emphasize investigating the adsorption energy of metal atoms on various ceramic carriers, controlling the environment, and chemical states. Additionally, new synthesis strategies such as atomic layer deposition and self-assembly techniques will be developed to achieve uniform dispersion and stable anchoring of metal atoms. Through these efforts, we can gain a better understanding and control of the behavior of metal atoms in catalysis and other applications, laying the groundwork for designing more efficient materials and catalysts in the future.
(2) In-depth theoretical and experimental investigation of the catalytic mechanisms of SACs is crucial for driving advancements in this field. With the progress of computational simulation and theoretical studies, the development of ML-based catalyst design methods to predict optimal metal-carrier combinations has become a trend. By employing first-principles calculations to simulate the electronic structure, reaction intermediates, and energy conversion processes of SACs, and integrating experimental data such as X-ray absorption spectroscopy and infrared spectroscopy, theoretical models and reaction pathways are validated. High-throughput computational screening is utilized to rapidly identify SACs with outstanding performance. It is anticipated that a substantial body of literature will emerge in the future, elucidating the catalytic mechanisms and reaction kinetics of SACs, and providing robust guidance for designing more efficient and stable SACs.
(3) One of the key tasks for future research is to develop low-cost, simple, and cost-effective methods for preparing SACs and exploring their broad applications in energy conversion, environmental protection, and other fields. Despite some reported methods for SACs preparation, they often suffer from issues such as high cost and complexity. Therefore, there is a need to seek simpler and more economically feasible preparation techniques to facilitate the practical application of SACs. This will help transition SACs from laboratory research to industrial applications and provide new solutions for addressing challenges in the fields of energy and environment.
(4) By innovatively designing ceramic supports, it is possible to achieve features with special electronic properties and surface activity, including aspects such as doping, heterostructures, and nanostructures. These designs are crucial in investigating the physicochemical properties of supports, as they directly influence the catalytic activity and selectivity of SACs. Further research will focus on exploring the performance optimization of SACs by adjusting the electronic structure and surface properties of the supports. Additionally, advanced preparation techniques such as sol-gel synthesis and deposition-precipitation methods can be considered to precisely control the morphology and structure of the supports. This aspect holds promise for providing new ideas and approaches for developing efficient and selective SACs, while also contributing to a deeper understanding of support-metal interactions, thereby laying the foundation for designing catalysts with enhanced activity and stability.
In conclusion, this work offers a comprehensive perspective and profound analysis of ceramic-based SACs in catalysis, delineating clear directions for future research. With the relentless progress of scientific inquiry and technological innovation, we believe SACs will increasingly shape the landscape of catalysis in the coming years.
DECLARATIONS
Authors’ contributions
Made the literature review and drafted the original version: Jin C, Zhang Q
Revised the manuscript: Linda Akua Agyapomaa A, Zhang H, Zeng X
Conceived and supervised the project: Zeng X
Availability of data and materials
Not applicable.
Financial support and sponsorship
This work was supported by the National Natural Science Foundation of China (No. 22269010), the Jiangxi Provincial Natural Science Foundation (No. 20224BAB214021), and the Major Research Program of Jingdezhen Ceramic Industry (No. 2023ZDGG002).
Conflicts of interest
All authors declared that there are no conflicts of interest.
Ethical approval and consent to participate
Not applicable.
Consent for publication
Not applicable.
Copyright
© The Author(s) 2024.
REFERENCES
1. Zhang L, Zhao ZJ, Wang T, Gong J. Nano-designed semiconductors for electro- and photoelectro-catalytic conversion of carbon dioxide. Chem Soc Rev 2018;47:5423-43.
2. Li D, Li X, Gong J. Catalytic reforming of oxygenates: state of the art and future prospects. Chem Rev 2016;116:11529-653.
3. Subbaraman R, Tripkovic D, Chang KC, et al. Trends in activity for the water electrolyser reactions on 3D M(Ni,Co,Fe,Mn) hydr(oxy)oxide catalysts. Nat Mater 2012;11:550-7.
4. Zhu J, Lv L, Zaman S, et al. Advances and challenges in single-site catalysts towards electrochemical CO2 methanation. Energy Environ Sci 2023;16:4812-33.
5. Tang C, Qiao SZ. How to explore ambient electrocatalytic nitrogen reduction reliably and insightfully. Chem Soc Rev 2019;48:3166-80.
6. Zeng X, Shui J, Liu X, et al. Single-atom to single-atom grafting of Pt1 onto Fe-N4 center: Pt1@Fe-N-C multifunctional electrocatalyst with significantly enhanced properties. Adv Energy Mater 2018;8:1701345.
7. Liu J. Advanced electron microscopy of metal-support interactions in supported metal catalysts. ChemCatChem 2011;3:934-48.
8. Schlögl R, Abd Hamid SB. Nanocatalysis: mature science revisited or something really new? Angew Chem Int Ed 2004;43:1628-37.
9. Xia Y, Xiong Y, Lim B, Skrabalak SE. Shape-controlled synthesis of metal nanocrystals: simple chemistry meets complex physics? Angew Chem Int Ed 2009;48:60-103.
11. Li H, Li L, Li Y. The electronic structure and geometric structure of nanoclusters as catalytic active sites. Nanotechnol Rev 2013;2:515-28.
12. Liu J, Gong H, Ye G, Fei H. Graphene oxide-derived single-atom catalysts for electrochemical energy conversion. Rare Met 2022;41:1703-26.
13. Li R, Zhao J, Liu B, Wang D. Atomic distance engineering in metal catalysts to regulate catalytic performance. Adv Mater 2024;36:e2308653.
15. Turner M, Golovko VB, Vaughan OPH, et al. Selective oxidation with dioxygen by gold nanoparticle catalysts derived from 55-atom clusters. Nature 2008;454:981-3.
16. Vajda S, Pellin MJ, Greeley JP, et al. Subnanometre platinum clusters as highly active and selective catalysts for the oxidative dehydrogenation of propane. Nat Mater 2009;8:213-6.
17. Lei Y, Mehmood F, Lee S, et al. Increased silver activity for direct propylene epoxidation via subnanometer size effects. Science 2010;328:224-8.
18. Li J, Stephanopoulos MF, Xia Y. Introduction: heterogeneous single-atom catalysis. Chem Rev 2020;120:11699-702.
19. Qiao B, Wang A, Yang X, et al. Single-atom catalysis of CO oxidation using Pt1/FeOx. Nat Chem 2011;3:634-41.
20. Wang S, Min XT, Qiao B, Yan N, Zhang T. Single-atom catalysts: in search of the holy grails in catalysis. Chin J Catal 2023;52:1-13.
21. Yang XF, Wang A, Qiao B, Li J, Liu J, Zhang T. Single-atom catalysts: a new frontier in heterogeneous catalysis. ACC Chem Res 2013;46:1740-8.
22. Boronat M, Leyva-Pérez A, Corma A. Theoretical and experimental insights into the origin of the catalytic activity of subnanometric gold clusters: attempts to predict reactivity with clusters and nanoparticles of gold. ACC Chem Res 2014;47:834-44.
23. Liu K, Zhao X, Ren G, et al. Strong metal-support interaction promoted scalable production of thermally stable single-atom catalysts. Nat Commun 2020;11:1263.
25. Lang R, Du X, Huang Y, et al. Single-atom catalysts based on the metal-oxide interaction. Chem Rev 2020;120:11986-2043.
26. Xu Y, Zheng W, Liu X, et al. Platinum single atoms on tin oxide ultrathin films for extremely sensitive gas detection. Mater Horiz 2020;7:1519-27.
27. Lan K, Wang R, Wei Q, et al. Stable Ti3+ defects in oriented mesoporous titania frameworks for efficient photocatalysis. Angew Chem Int Ed 2020;132:17829-36.
28. Zheng T, Jiang K, Ta N, et al. Large-scale and highly selective CO2 electrocatalytic reduction on nickel single-atom catalyst. Joule 2019;3:265-78.
29. Kim MS, Lee J, Kim HS, et al. Heme cofactor-resembling Fe-N single site embedded graphene as nanozymes to selectively detect
30. Liu L, Zhu QY, Li J, et al. Atomistic engineering of Ag/Pt nanoclusters for remarkably boosted mass electrocatalytic activity. Energy Mater 2022;2:200007.
31. Ji S, Chen Y, Wang X, Zhang Z, Wang D, Li Y. Chemical synthesis of single atomic site catalysts. Chem Rev 2020;120:11900-55.
32. Chen Y, Li H, Zhao W, et al. Optimizing reaction paths for methanol synthesis from CO2 hydrogenation via metal-ligand cooperativity. Nat Commun 2019;10:1885.
33. Najam T, Ahmad Khan N, Ahmad Shah SS, et al. Metal-organic frameworks derived electrocatalysts for oxygen and carbon dioxide reduction reaction. Chem Rec 2022;22:e202100329.
34. Guillon O. Ceramic materials for energy conversion and storage: a perspective. Int J Ceram Eng Sci 2021;3:100-4.
35. Yang H, Wang CA, Dong Y. Energy ceramic design for robust battery cathodes and solid electrolytes. Adv Powder Mater 2024;3:100185.
36. Wang F, Dong B, Wang J, et al. Self-supported porous heterostructure WC/WO3-x ceramic electrode for hydrogen evolution reaction in acidic and alkaline media. J Adv Ceram 2022;11:1208-21.
37. Yang J, Li W, Wang D, Li Y. Electronic metal-support interaction of single-atom catalysts and applications in electrocatalysis. Adv Mater 2020;32:e2003300.
38. Zeng L, Xue C. Single metal atom decorated photocatalysts: progress and challenges. Nano Res 2021;14:934-44.
39. Peng L, Shang L, Zhang T, Waterhouse GIN. Waterhouse GIN. Recent advances in the development of single-atom catalysts for oxygen electrocatalysis and zinc-air batteries. Adv Energy Mater 2020;10:2003018.
40. Zhuang Z, Kang Q, Wang D, Li Y. Single-atom catalysis enables long-life, high-energy lithium-sulfur batteries. Nano Res 2020;13:1856-66.
41. Hoang S, Guo Y, Binder AJ, et al. Activating low-temperature diesel oxidation by single-atom Pt on TiO2 nanowire array. Nat Commun 2020;11:1062.
42. Kunwar D, Zhou S, Delariva A, et al. Stabilizing high metal loadings of thermally stable platinum single atoms on an industrial catalyst support. ACS Catal 2019;9:3978-90.
43. Dvořák F, Farnesi Camellone M, Tovt A, et al. Creating single-atom Pt-ceria catalysts by surface step decoration. Nat Commun 2016;7:10801.
44. Qiao B, Liu J, Wang YG, et al. Highly efficient catalysis of preferential oxidation of CO in H2-rich stream by gold single-atom catalysts. ACS Catal 2015;5:6249-54.
45. Qiao B, Liang JX, Wang A, et al. Ultrastable single-atom gold catalysts with strong covalent metal-support interaction (CMSI). Nano Res 2015;8:2913-24.
46. Liu J, Wang T, Liu X, et al. Reducible Co3+-O sites of Co-Ni-P-Ox on CeO2 nanorods boost acidic water oxidation via interfacial charge transfer-promoted surface reconstruction. ACS Catal 2023;13:5194-204.
47. Yan S, Gao Z, Ding J, et al. Nanocomposites based on nanoceria regulate the immune microenvironment for the treatment of polycystic ovary syndrome. J Nanobiotechnol 2023;21:412.
48. Yavo N, Yeheskel O, Wachtel E, Ehre D, Frenkel AI, Lubomirsky I. Relaxation and saturation of electrostriction in 10 mol% Gd-doped ceria ceramics. Acta Mater 2018;144:411-8.
49. Jones J, Xiong H, DeLaRiva AT, et al. Thermally stable single-atom platinum-on-ceria catalysts via atom trapping. Science 2016;353:150-4.
50. Nie L, Mei D, Xiong H, et al. Activation of surface lattice oxygen in single-atom Pt/CeO2 for low-temperature CO oxidation. Science 2017;358:1419-23.
51. Beniya A, Higashi S. Towards dense single-atom catalysts for future automotive applications. Nat Catal 2019;2:590-602.
52. Farmer JA, Campbell CT. Ceria maintains smaller metal catalyst particles by strong metal-support bonding. Science 2010;329:933-6.
53. Corma A, Atienzar P, García H, Chane-Ching JY. Hierarchically mesostructured doped CeO2 with potential for solar-cell use. Nat Mater 2004;3:394-7.
54. Prieur D, Bonani W, Popa K, et al. Size dependence of lattice parameter and electronic structure in CeO2 nanoparticles. Inorg Chem 2020;59:5760-7.
55. Paun C, Safonova OV, Szlachetko J, et al. Polyhedral CeO2 nanoparticles: size-dependent geometrical and electronic structure. J Phys Chem C 2012;116:7312-7.
56. Xu J, Harmer J, Li G, et al. Size dependent oxygen buffering capacity of ceria nanocrystals. Chem Commun 2010;46:1887-9.
57. Wang Y, Chen Z, Han P, et al. Single-atomic Cu with multiple oxygen vacancies on ceria for electrocatalytic CO2 reduction to CH4. ACS Catal 2018;8:7113-9.
58. Xu J, Wang Y, Wang K, et al. Single-atom Rh on high-index CeO2 facet for highly enhanced catalytic CO oxidation. Angew Chem Int Ed 2023;62:e202302877.
59. Ruan X, Li S, Huang C, Zheng W, Cui X, Ravi SK. Catalyzing artificial photosynthesis with TiO2 heterostructures and hybrids: emerging trends in a classical yet contemporary photocatalyst. Adv Mater 2024;36:e2305285.
60. Martinez-Oviedo A, Kshetri YK, Joshi B, Lee SW. Surface modification of blue TiO2 with silane coupling agent for NOx abatement. Prog Nat Sci 2021;31:230-8.
61. Kuai L, Chen Z, Liu S, et al. Titania supported synergistic palladium single atoms and nanoparticles for room temperature ketone and aldehydes hydrogenation. Nat Commun 2020;11:48.
62. Han B, Guo Y, Huang Y, et al. Strong metal-support interactions between Pt single atoms and TiO2. Angew Chem Int Ed 2020;59:11824-9.
63. Chen Y, Ji S, Sun W, et al. Engineering the atomic interface with single platinum atoms for enhanced photocatalytic hydrogen production. Angew Chem Int Ed 2020;132:1311-7.
64. Wan J, Chen W, Jia C, et al. Defect effects on TiO2 nanosheets: stabilizing single atomic site Au and promoting catalytic properties. Adv Mater 2018;30:1705369.
65. Yang M, Allard LF, Flytzani-Stephanopoulos M. Atomically dispersed Au-(OH)x species bound on titania catalyze the low-temperature water-gas shift reaction. J Am Chem Soc 2013;135:3768-71.
66. Xu H, Liu C, Guo W, et al. Sodium alginate/Al2O3 fiber nanocomposite aerogel with thermal insulation and flame retardancy properties. Chem Eng J 2024;489:151223.
67. Hu J, Gao T, Li M, Liu X. Synthesis of an (Al3BC + Al2O3)/Al composite with high stiffness and attractive high-temperature tensile properties. Mater Res Lett 2024;12:355-62.
68. Zhao L, Liang Y, Ma J, et al. Ultra-steep-slope and high-stability of CuInP2S6/WS2 ferroelectric negative capacitor transistors by passivation effect and dual-gate modulation. Adv Funct Mater 2023;33:2306708.
69. Li H, Hu J, Zhang Y, et al. Single-transistor optoelectronic spiking neuron with optogenetics-inspired spatiotemporal dynamics. Adv Funct Mater 2024;34:2314456.
70. Wang F, Ma J, Xin S, et al. Resolving the puzzle of single-atom silver dispersion on nanosized γ-Al2O3 surface for high catalytic performance. Nat Commun 2020;11:529.
71. Qin R, Zhou L, Liu P, et al. Alkali ions secure hydrides for catalytic hydrogenation. Nat Catal 2020;3:703-9.
72. Yang K, Liu Y, Deng J, et al. Three-dimensionally ordered mesoporous iron oxide-supported single-atom platinum: highly active catalysts for benzene combustion. Appl Catal B Environ Energy 2019;244:650-9.
73. Parastaev A, Muravev V, Huertas Osta E, et al. Boosting CO2 hydrogenation via size-dependent metal-support interactions in cobalt/ceria-based catalysts. Nat Catal 2020;3:526-33.
74. van Deelen TW, Hernández Mejía C, de Jong KP. Control of metal-support interactions in heterogeneous catalysts to enhance activity and selectivity. Nat Catal 2019;2:955-70.
75. Liu SR, Luo ST, Wu XD, et al. Application of silica-alumina as hydrothermally stable supports for Pt catalysts for acid-assisted soot oxidation. Rare Met 2023;42:1614-23.
76. Vaudry F, Khodabandeh S, Davis ME. Synthesis of pure alumina mesoporous materials. Chem Mater 1996;8:1451-64.
77. Li W, Kovarik L, Mei D, et al. A general mechanism for stabilizing the small sizes of precious metal nanoparticles on oxide supports. Chem Mater 2014;26:5475-81.
78. Shang H, Chen W, Jiang Z, Zhou D, Zhang J. Atomic-dispersed platinum anchored on porous alumina sheets as an efficient catalyst for diboration of alkynes. Chem Commun 2020;56:3127-30.
79. Zhang Z, Zhu Y, Asakura H, et al. Thermally stable single atom Pt/m-Al2O3 for selective hydrogenation and CO oxidation. Nat Commun 2017;8:16100.
80. Lan F, Zhang H, Zhao C, Shu Y, Guan Q, Li W. Copper clusters encapsulated in carbonaceous mesoporous silica nanospheres for the valorization of biomass-derived molecules. ACS Catal 2022;12:5711-25.
81. Zhang D, Cai H, Su Y, Sun W, Yang D, Ozin GA. Silica samurai: aristocrat of energy and environmental catalysis. Chem Catal 2022;2:1893-918.
82. Pellico J, Vass L, Carrascal-Miniño A, et al. In vivo real-time positron emission particle tracking (PEPT) and single particle PET. Nat Nanotechnol 2024;19:668-76.
83. Du J, Liu B, Zhao T, et al. Silica nanoparticles protect rice against biotic and abiotic stresses. J Nanobiotechnol 2022;20:197.
84. Rice SB, Koo JY, Disko MM, Treacy MMJ. On the imaging of Pt atoms in zeolite frameworks. Ultramicroscopy 1990;34:108-18.
85. Maschmeyer T, Rey F, Sankar G, Thomas JM. Heterogeneous catalysts obtained by grafting metallocene complexes onto mesoporous silica. Nature 1995;378:159-62.
86. Duan H, Li M, Zhang G, et al. Single-site palladium (II) catalyst for oxidative heck reaction: catalytic performance and kinetic investigations. ACS Catal 2015;5:3752-9.
87. Wu W, Cui E, Zhang Y, et al. Involving single-atom silver(0) in selective dehalogenation by AgF under visible-light irradiation. ACS Catal 2019;9:6335-41.
88. Zhang H, Zeng X, Zhang Q, Zhang Z, Jin C, Yu R. Dual template-induced construction of three-dimensional porous SiO2/NC/Co-CNTs heterostructure with highly dispersed active sites for efficient oxygen evolution reaction. Tungsten 2024;6:585-95.
89. Yang M, Li S, Wang Y, et al. Catalytically active Au-O(OH)x-species stabilized by alkali ions on zeolites and mesoporous oxides. Science 2014;346:1498-501.
90. De S, Babak MV, Hülsey MJ, Ang WH, Yan N. Designed precursor for the controlled synthesis of highly active atomic and sub-nanometric platinum catalysts on mesoporous silica. Chem Asian J 2018;13:1053-9.
91. Zhai Y, Pierre D, Si R, et al. Alkali-stabilized Pt-OHx species catalyze low-temperature water-gas shift reactions. Science 2010;329:1633-6.
92. Sun Q, Wang N, Xu Q, Yu J. Nanopore-supported metal nanocatalysts for efficient hydrogen generation from liquid-phase chemical hydrogen storage materials. Adv Mater 2020;32:e2001818.
93. Li X, Pereira-Hernández XI, Chen Y, et al. Functional CeOx nanoglues for robust atomically dispersed catalysts. Nature 2022;611:284-8.
94. Mokhtar M, Basahel SN, Ali TT. Effect of synthesis methods for mesoporous zirconia on its structural and textural properties. J Mater Sci 2013;48:2705-13.
95. Jia K, Zheng L, Liu W, et al. A new and simple way to prepare monolithic solid oxide fuel cell stack by stereolithography 3D printing technology using 8 mol% yttria stabilized zirconia photocurable slurry. J Eur Ceram Soc 2022;42:4275-85.
96. Chang CH, Lin CY, Chang CH, Liu FH, Huang YT, Liao YS. Enhanced biomedical applicability of ZrO2-SiO2 ceramic composites in 3D printed bone scaffolds. Sci Rep 2022;12:6845.
97. Wang S, Li X, Wang J, et al. Enhanced electromechanical properties in MnCO3-modified Pb(Ni, Nb)O3-PbZrO3-PbTiO3 ceramics via defect and domain engineering. J Am Ceram Soc 2023;106:1970-80.
98. Pokrovski K, Jung KT, Bell AT. Investigation of CO and CO2 adsorption on tetragonal and monoclinic zirconia. Langmuir 2001;17:4297-303.
99. Wang J, Li G, Li Z, et al. A highly selective and stable ZnO-ZrO2 solid solution catalyst for CO2 hydrogenation to methanol. Sci Adv 2017;3:e1701290.
100. Wang Y, Kattel S, Gao W, et al. Exploring the ternary interactions in Cu-ZnO-ZrO2 catalysts for efficient CO2 hydrogenation to methanol. Nat Commun 2019;10:1166.
101. Samson K, Śliwa M, Socha RP, et al. Influence of ZrO2 structure and copper electronic state on activity of Cu/ZrO2 catalysts in methanol synthesis from CO2. ACS Catal 2014;4:3730-41.
102. Arena F, Barbera K, Italiano G, Bonura G, Spadaro L, Frusteri F. Synthesis, characterization and activity pattern of Cu-ZnO/ZrO2 catalysts in the hydrogenation of carbon dioxide to methanol. J Catal 2007;249:185-94.
103. Guo X, Mao D, Lu G, Wang S, Wu G. Glycine-nitrate combustion synthesis of CuO-ZnO-ZrO2 catalysts for methanol synthesis from CO2 hydrogenation. J Catal 2010;271:178-85.
104. Kondratenko EV, Mul G, Baltrusaitis J, Larrazábal GO, Pérez-Ramírez J. Status and perspectives of CO2 conversion into fuels and chemicals by catalytic, photocatalytic and electrocatalytic processes. Energy Environ Sci 2013;6:3112-35.
105. Du W, Sun Z, Chen K, Wang F, Chu K. Nb1-Zr dual active sites constructed on ZrO2 boost nitrite-to-ammonia electroreduction. Chem Eng J 2024;481:148733.
106. Choudhary N, Jiang S, Pham H, et al. Precisely designed cobalt single atom on ZrO2 support for chemical CO2 fixation. Appl Catal B Environ Energy 2024;344:123627.
107. Huang J, Han J, Wang R, et al. Improving electrocatalysts for oxygen evolution using NixFe3-xO4/Ni hybrid nanostructures formed by solvothermal synthesis. ACS Energy Lett 2018;3:1698-707.
109. Liu X, He L, Han G, Sheng J, Yu Y, Yang W. Design of rich defects carbon coated MnFe2O4/LaMnO3/LaFeO3 heterostructure nanocomposites for broadband electromagnetic wave absorption. Chem Eng J 2023;476:146199.
110. Liu X, Duan Y, Guo Y, et al. In situ construction of complex spinel ferrimagnet in multi-elemental alloy for modulating natural resonance and highly efficient electromagnetic absorption. Chem Eng J 2023;462:142200.
111. Avcı ÖN, Sementa L, Fortunelli A. Mechanisms of the oxygen evolution reaction on NiFe2O4 and CoFe2O4 inverse-spinel oxides. ACS Catal 2022;12:9058-73.
112. Rushiti A, Hättig C, Wen B, Selloni A. Structure and reactivity of pristine and reduced spinel CoFe2O4 (001)/(100) surfaces. J Phys Chem C 2021;125:9774-81.
113. Zhu H, Zhang S, Huang YX, Wu L, Sun S. Monodisperse MxFe3-xO4 (M= Fe, Cu, Co, Mn) nanoparticles and their electrocatalysis for oxygen reduction reaction. Nano Lett 2013;13:2947-51.
114. Liu J, Zhu D, Ling T, Vasileff A, Qiao S. S-NiFe2O4 ultra-small nanoparticle built nanosheets for efficient water splitting in alkaline and neutral pH. Nano Energy 2017;40:264-73.
115. Sun S, Sun Y, Zhou Y, et al. Shifting oxygen charge towards octahedral metal: a way to promote water oxidation on cobalt spinel oxides. Angew Chem Int Ed 2019;131:6103-8.
116. Zhou Y, Sun S, Wei C, et al. Significance of engineering the octahedral units to promote the oxygen evolution reaction of spinel oxides. Adv Mater 2019;31:e1902509.
117. Geng KQ, Yang MQ, Meng JX, et al. Engineering layered/spinel heterostructure via molybdenum doping towards highly stable Li-rich cathodes. Tungsten 2022;4:323-35.
118. Shan J, Ye C, Chen S, et al. Short-range ordered iridium single atoms integrated into cobalt oxide spinel structure for highly efficient electrocatalytic water oxidation. J Am Chem Soc 2021;143:5201-11.
119. Wang Y, Zhu YQ, Xie Z, et al. Efficient electrocatalytic oxidation of glycerol via promoted OH* generation over single-atom-bismuth-doped spinel Co3O4. ACS Catal 2022;12:12432-43.
120. Yin WJ, Weng B, Ge J, Sun Q, Li Z, Yan Y. Oxide perovskites, double perovskites and derivatives for electrocatalysis, photocatalysis, and photovoltaics. Energy Environ Sci 2019;12:442-62.
121. Sun C, Alonso JA, Bian J. Recent advances in perovskite-type oxides for energy conversion and storage applications. Adv Energy Mater 2021;11:2000459.
122. Song HJ, Yoon H, Ju B, Kim D. Highly efficient perovskite-based electrocatalysts for water oxidation in acidic environments: a mini review. Adv Energy Mater 2021;11:2002428.
123. Sutherland LJ, Benitez-rodriguez J, Vak D, et al. A high-pressure isostatic lamination technique to fabricate versatile carbon electrode-based perovskite solar cells. Commun Mater 2024;5:90.
124. Hailegnaw B, Demchyshyn S, Putz C, et al. Flexible quasi-2D perovskite solar cells with high specific power and improved stability for energy-autonomous drones. Nat Energy 2024;9:677-90.
125. Xia Y, Zhu M, Qin L, et al. Organic-inorganic hybrid quasi-2D perovskites incorporated with fluorinated additives for efficient and stable four-terminal tandem solar cells. Energy Mater 2023;3:300004.
126. Zhang D, Wang Y, Peng Y, et al. Novel high-entropy perovskite-type symmetrical electrode for efficient and durable carbon dioxide reduction reaction. Adv Powder Mater 2023;2:100129.
127. Dai J, Zhu Y, Tahini HA, et al. Single-phase perovskite oxide with super-exchange induced atomic-scale synergistic active centers enables ultrafast hydrogen evolution. Nat Commun 2020;11:5657.
128. Jung JI, Jeong HY, Kim MG, Nam G, Park J, Cho J. Fabrication of Ba0.5Sr0.5Co0.8Fe0.2O(3-δ) catalysts with enhanced electrochemical performance by removing an inherent heterogeneous surface film layer. Adv Mater 2015;27:266-71.
129. Nishihata Y, Mizuki J, Akao T, et al. Self-regeneration of a Pd-perovskite catalyst for automotive emissions control. Nature 2002;418:164-7.
130. Tanaka H, Taniguchi M, Uenishi M, et al. Self-regenerating Rh- and Pt-based perovskite catalysts for automotive-emissions control. Angew Chem Int Ed 2006;45:5998-6002.
131. Onn TM, Monai M, Dai S, et al. Smart Pd catalyst with improved thermal stability supported on high-surface-area LaFeO3 prepared by atomic layer deposition. J Am Chem Soc 2018;140:4841-8.
132. Tian C, Zhang H, Zhu X, et al. A new trick for an old support: Stabilizing gold single atoms on LaFeO3 perovskite. Appl Catal B Environ Energy 2020;261:118178.
133. Shin H, Ko J, Park C, et al. Sacrificial template-assisted synthesis of inorganic nanosheets with high-loading single-atom catalysts: a general approach. Adv Funct Mater 2022;32:2110485.
134. Niu S, Yang J, Qi H, et al. Single-atom Pt promoted Mo2C for electrochemical hydrogen evolution reaction. J Energy Chem 2021;57:371-7.
135. Zeng X, Ye Y, Wang Y, Yu R, Moskovits M, Stucky GD. Honeycomb-like MXene/NiFePx-NC with "continuous" single-crystal enabling high activity and robust durability in electrocatalytic oxygen evolution reactions. J Adv Ceram 2023;12:553-64.
136. Zhang Z, Liang T, Jin C, et al. Synergistically coupling CoS/FeS2 heterojunction nanosheets on a MXene via a dual molten salt etching strategy for efficient oxygen evolution reaction. J Mater Chem A 2024;12:14517-30.
137. Zeng X, Jiang X, Ning Y, Gao Y, Che R. Constructing built-in electric fields with semiconductor junctions and Schottky junctions based on Mo-MXene/Mo-metal sulfides for electromagnetic response. Nanomicro Lett 2024;16:213.
138. Jin C, Peng H, Zeng X, Liu Z, Ding D. Hierarchical assembly of NiFe-PB-derived bimetallic phosphides on 3D Ti3C2 MXene ribbon networks for efficient oxygen evolution. ChemPhysMater 2024;3:118-24.
139. Yu LH, Tao X, Feng SR, et al. Recent development of three-dimension printed graphene oxide and MXene-based energy storage devices. Tungsten 2024;6:196-211.
140. Zeng X, Tan Y, Xia L, Zhang Q, Stucky GD. MXene-derived Ti3C2-Co-TiO2 nanoparticle arrays via cation exchange for highly efficient and stable electrocatalytic oxygen evolution. Chem Commun 2023;59:880-3.
141. Luo J, Gao S, Luo H, et al. Superhydrophobic and breathable smart MXene-based textile for multifunctional wearable sensing electronics. Chem Eng J 2021;406:126898.
142. Wu X, Han B, Zhang H, et al. Compressible, durable and conductive polydimethylsiloxane-coated MXene foams for high-performance electromagnetic interference shielding. Chem Eng J 2020;381:122622.
143. Zhong Q, Li Y, Zhang G. Two-dimensional MXene-based and MXene-derived photocatalysts: recent developments and perspectives. Chem Eng J 2021;409:128099.
144. Zeng X, Duan D, Zhang X, et al. Doping and interface engineering in a sandwich Ti3C2Tx/MoS2-xPx heterostructure for efficient hydrogen evolution. J Mater Chem C 2022;10:4140-7.
145. Zeng X, Zhao C, Jiang X, Yu R, Che R. Functional tailoring of multi-dimensional pure MXene nanostructures for significantly accelerated electromagnetic wave absorption. Small 2023;19:e2303393.
146. Zeng X, Jiang X, Ning Y, Hu F, Fan B. Construction of dual heterogeneous interface between zigzag-like Mo-MXene nanofibers and small CoNi@NC nanoparticles for electromagnetic wave absorption. J Adv Ceram 2023;12:1562-76.
147. Ramalingam V, Varadhan P, Fu HC, et al. Heteroatom-mediated interactions between ruthenium single atoms and an MXene support for efficient hydrogen evolution. Adv Mater 2019;31:e1903841.
148. Zhang J, Wang E, Cui S, Yang S, Zou X, Gong Y. Single-atom Pt anchored on oxygen vacancy of monolayer Ti3C2Tx for superior hydrogen evolution. Nano Lett 2022;22:1398-405.
149. Zeng X, Zhang H, Yu R, Stucky GD, Qiu J. A phase and interface co-engineered MoPxSy@NiFePxSy@NPS-C hierarchical heterostructure for sustainable oxygen evolution reaction. J Mater Chem A 2023;11:14272-83.
150. Luo F, Yu Y, Long X, Li C, Xiong T, Yang Z. Boosting catalytic activity toward methanol oxidation reaction for platinum via heterostructure engineering. J Colloid Interface Sci 2024;656:450-6.
151. Seh ZW, Kibsgaard J, Dickens CF, Chorkendorff I, Nørskov JK, Jaramillo TF. Combining theory and experiment in electrocatalysis: insights into materials design. Science 2017;355:eaad4998.
152. Zeng X, Zhang Q, Shen Z, Zhang H, Wang T, Liu Z. Doping and vacancy engineering in a sandwich-like g-C3N4/NiCo2O4 heterostructure for robust oxygen evolution. ChemNanoMat 2022;8:e202200191.
153. Jing H, Zhu P, Zheng X, Zhang Z, Wang D, Li Y. Theory-oriented screening and discovery of advanced energy transformation materials in electrocatalysis. Adv Powder Mater 2022;1:100013.
154. Wei J, Xiao K, Chen Y, Guo X, Huang B, Liu Z. In situ precise anchoring of Pt single atoms in spinel Mn3O4 for a highly efficient hydrogen evolution reaction. Energy Environ Sci 2022;15:4592-600.
155. Kim M, Kim S, Park J, et al. Reconstructing oxygen-deficient zirconia with ruthenium catalyst on atomic-scale interfaces toward hydrogen production. Adv Funct Mater 2023;33:2300673.
156. Navarra MA, Croce F, Scrosati B. New, high temperature superacid zirconia-doped Nafion™ composite membranes. J Mater Chem 2007;17:3210-5.
157. Fabbri E, Habereder A, Waltar K, Kötz R, Schmidt TJ. Developments and perspectives of oxide-based catalysts for the oxygen evolution reaction. Catal Sci Technol 2014;4:3800-21.
158. Zeng X, Zhang Q, Jin C, Huang H, Gao Y. Fe-induced electronic transfer and structural evolution of lotus pod-like CoNiFePx@P,
159. Long X, Xiong T, Bao H, et al. Tip and heterogeneous effects co-contribute to a boosted performance and stability in zinc air battery. J Colloid Interface Sci 2024;662:676-85.
160. Nguyen DC, Luyen Doan TL, Prabhakaran S, et al. Hierarchical Co and Nb dual-doped MoS2 nanosheets shelled micro-TiO2 hollow spheres as effective multifunctional electrocatalysts for HER, OER, and ORR. Nano Energy 2021;82:105750.
161. Liu J, Liu X, Shi H, et al. Breaking the scaling relations of oxygen evolution reaction on amorphous NiFeP nanostructures with enhanced activity for overall seawater splitting. Appl Catal B Environ Energy 2022;302:120862.
162. Zhu H, Wang Y, Jiang Z, Deng B, Xin Y, Jiang Z. Defect engineering promoted ultrafine Ir nanoparticle growth and Sr single-atom adsorption on TiO2 nanowires to achieve high-performance overall water splitting in acidic media. Adv Energy Mater 2024;14:2303987.
163. Wen N, Xia Y, Wang H, et al. Large-scale synthesis of spinel NixMn3-xO4 solid solution immobilized with iridium single atoms for efficient alkaline seawater electrolysis. Adv Sci 2022;9:e2200529.
164. Pan J, Xu YY, Yang H, Dong Z, Liu H, Xia BY. Advanced architectures and relatives of air electrodes in Zn-air batteries. Adv Sci 2018;5:1700691.
165. Ge X, Sumboja A, Wuu D, et al. Oxygen reduction in alkaline media: from mechanisms to recent advances of catalysts. ACS Catal 2015;5:4643-67.
166. Li X, Li C, Xie Y, Pan S, Luo F, Yang Z. Anion effect on oxygen reduction reaction activity of nitrogen doped carbon nanotube encapsulated cobalt nanoparticles. Appl Surf Sci 2024;648:158975.
167. Zhu Y, Peng W, Li Y, Zhang G, Zhang F, Fan X. Modulating the electronic structure of single-atom catalysts on 2D nanomaterials for enhanced electrocatalytic performance. Small Methods 2019;3:1800438.
168. Zhang J, Dong X, Xing W, et al. Engineering iron single atomic sites with adjacent ZrO2 nanoclusters via ligand-assisted strategy for effective oxygen reduction reaction and high-performance Zn-air batteries. Chem Eng J 2021;420:129938.
169. ul Haq M, Wu DH, Ajmal Z, et al. Derived-2D Nb4C3Tx sheets with interfacial self-assembled Fe-N-C single-atom catalyst for electrocatalysis in water splitting and durable zinc-air battery. Appl Catal B Environ Energy 2024;344:123632.
170. Xu X, Li X, Lu W, et al. Collective effect in a multicomponent ensemble combining single atoms and nanoparticles for efficient and durable oxygen reduction. Angew Chem Int Ed 2024;63:e202400765.
171. Cao S, Chen H, Hu Y, et al. MXene-based single atom catalysts for efficient CO2RR towards CO: a novel strategy for high-throughput catalyst design and screening. Chem Eng J 2023;461:141936.
172. Tan X, Sun K, Zhuang Z, et al. Stabilizing copper by a reconstruction-resistant atomic Cu-O-Si interface for electrochemical CO2 reduction. J Am Chem Soc 2023;145:8656-64.
173. Iqbal MS, Yao Z, Ruan Y, et al. Single-atom catalysts for electrochemical N2 reduction to NH3. Rare Met 2023;42:1075-97.
174. Suryanto BHR, Du HL, Wang D, Chen J, Simonov AN, Macfarlane DR. Challenges and prospects in the catalysis of electroreduction of nitrogen to ammonia. Nat Catal 2019;2:290-6.
175. Zhang M, Xu W, Ma CL, Yu J, Liu YT, Ding B. Highly active and selective electroreduction of N2 by the catalysis of Ga single atoms stabilized on amorphous TiO2 nanofibers. ACS Nano 2022;16:4186-96.
176. Han Z, Tranca D, Rodríguez-Hernández F, et al. Embedding Ru clusters and single atoms into perovskite oxide boosts nitrogen fixation and affords ultrahigh ammonia yield rate. Small 2023;19:e2208102.
177. Nguyen TP, Kim IT. Single-atom transition metal photocatalysts for hydrogen evolution reactions. Catalysts 2022;12:1304.
178. Fauth C, Lenzer A, Abdel-Mageed AM, Jürgen Behm R. Temporal analysis of products (TAP) reactor study of the dynamics of CO2 interaction with a Ru/γ-Al2O3 supported catalyst. Appl Catal B Environ Energy 2023;334:122817.
179. Lin Z, Wang Y, Peng Z, et al. Single-metal atoms and ultra-small clusters manipulating charge carrier migration in polymeric perylene diimide for efficient photocatalytic oxygen production. Adv Energy Mater 2022;12:2200716.
180. Ran J, Zhang J, Yu J, Jaroniec M, Qiao SZ. Earth-abundant cocatalysts for semiconductor-based photocatalytic water splitting. Chem Soc Rev 2014;43:7787-812.
181. Akhundi A, Habibi-Yangjeh A, Abitorabi M, Rahim Pouran S. Review on photocatalytic conversion of carbon dioxide to value-added compounds and renewable fuels by graphitic carbon nitride-based photocatalysts. Catal Rev 2019;61:595-628.
182. Xia B, Zhang Y, Shi B, Ran J, Davey K, Qiao S. Photocatalysts for hydrogen evolution coupled with production of value-added chemicals. Small Methods 2020;4:2000063.
183. Tentu RD, Basu S. Photocatalytic water splitting for hydrogen production. Curr Opin Electrochem 2017;5:56-62.
184. Chen X, Zhao J, Li G, Zhang D, Li H. Recent advances in photocatalytic renewable energy production. Energy Mater 2022;2:200001.
185. Wang F, Yang S, Han S, et al. Synthesis of Cu-TiO2/CuS pn heterojunction via in situ sulfidation for highly efficient photocatalytic NO removal. Prog Nat Sci 2022;32:561-9.
186. Kerketta U, Kim H, Denisov N, Schmuki P. Grätzel-type TiO2 anatase layers as host for pt single atoms: highly efficient and stable photocatalytic hydrogen production. Adv Energy Mater 2024;14:2302998.
187. Lee BH, Park S, Kim M, et al. Reversible and cooperative photoactivation of single-atom Cu/TiO2 photocatalysts. Nat Mater 2019;18:620-6.
188. Chen Y, Qi M, Li Y, et al. Activating two-dimensional Ti3C2Tx-MXene with single-atom cobalt for efficient CO2 photoreduction. Cell Rep Phys Sci 2021;2:100371.
189. Xu Q, Wang L, Sheng X, et al. Understanding the synergistic mechanism of single atom Co-modified perovskite oxide for piezo-photocatalytic CO2 reduction. Appl Catal B Environ Energy 2023;338:123058.
190. Cao Y, Guo L, Dan M, et al. Modulating electron density of vacancy site by single Au atom for effective CO2 photoreduction. Nat Commun 2021;12:1675.
191. Gao C, Low J, Long R, Kong T, Zhu J, Xiong Y. Heterogeneous single-atom photocatalysts: fundamentals and applications. Chem Rev 2020;120:12175-216.
192. Li S, Li Y, Bai H, et al. Penta-coordinated aluminum species: anchoring Au single atoms for photocatalytic CO2 reduction. Appl Catal B Environ Energy 2024;345:123703.
193. Li SQ, Liu Y, Li YL, et al. Development of γ-Al2O3 with oxygen vacancies induced by amorphous structures for photocatalytic reduction of CO2. Chem Commun 2022;58:11649-52.
194. Zhao Z, Xiao D, Chen K, et al. Nature of five-coordinated Al in γ-Al2O3 revealed by ultra-high-field solid-state NMR. ACS Cent Sci 2022;8:795-803.
195. Martin O, Martín AJ, Mondelli C, et al. Indium oxide as a superior catalyst for methanol synthesis by CO2 hydrogenation. Angew Chem Int Ed 2016;55:6261-5.
196. Zhao H, Yu R, Ma S, et al. The role of Cu1-O3 species in single-atom Cu/ZrO2 catalyst for CO2 hydrogenation. Nat Catal 2022;5:818-31.
197. Wu C, Lin L, Liu J, et al. Inverse ZrO2/Cu as a highly efficient methanol synthesis catalyst from CO2 hydrogenation. Nat Commun 2020;11:5767.
Cite This Article
How to Cite
Jin, C.; Zhang Q.; Linda Akua Agyapomaa A.; Zhang H.; Zeng X. Construction and catalytic applications of advanced ceramic-supported single atoms. Microstructures. 2024, 4, 2024054. http://dx.doi.org/10.20517/microstructures.2024.36
Download Citation
Export Citation File:
Type of Import
Tips on Downloading Citation
Citation Manager File Format
Type of Import
Direct Import: When the Direct Import option is selected (the default state), a dialogue box will give you the option to Save or Open the downloaded citation data. Choosing Open will either launch your citation manager or give you a choice of applications with which to use the metadata. The Save option saves the file locally for later use.
Indirect Import: When the Indirect Import option is selected, the metadata is displayed and may be copied and pasted as needed.
About This Article
Special Issue
Copyright
Data & Comments
Data
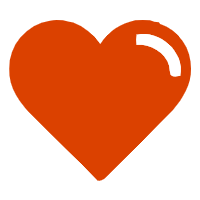
Comments
Comments must be written in English. Spam, offensive content, impersonation, and private information will not be permitted. If any comment is reported and identified as inappropriate content by OAE staff, the comment will be removed without notice. If you have any queries or need any help, please contact us at support@oaepublish.com.