Modulating single-atom M-N-C electrocatalysts for the oxygen reduction: the insights beyond the first coordination shell
Abstract
The oxygen reduction reaction (ORR) is a pivotal process in electrochemical energy systems such as fuel cells and metal-air batteries. Recent advancements have highlighted the single-atom metal-nitrogen-carbon (M-N-C) catalysts for their exceptional ORR electrocatalytic performance. However, further exploration is needed for the optimization methods of single atomic active sites. Significantly, the modulation of coordination environment emerges as a pivotal technique for the enhancement of M-N-C catalysts, while extending this modulation beyond the first coordination shell has ignited substantial investigation. This review delves into the frontier of M-N-C optimization by transcending the first coordination shell, presenting a comprehensive analysis of innovative strategies that modulate the electronic structure and reactivity of MN4 sites. The primary focus lies in three regulation approaches: regulating atomic entities, introducing metallic species and tailoring non-metallic modulators. These strategies are scrutinized for their ability to fine-tune the ORR activity and stability at the atomic level. By providing a clearer trajectory for future research, this review should be able to inspire novel designs of high-performance M-N-C ORR catalysts.
Keywords
INTRODUCTION
The global energy landscape is undergoing a transformative shift towards sustainable sources, necessitating advancements in energy conversion technologies. The electrochemical reduction of oxygen (ORR) plays a crucial role in the advancement of future renewable energy storage and conversion technologies, including fuel cells and metal-air batteries[1-3]. However, the inherent challenge with the ORR process lies in its sluggish kinetics, which originates from the intricate multi-step electron transfer process and inefficient mass transfer process. Currently, ORR is primarily categorized into two main processes based on electron transfer numbers: the 2e- process and the 4e- process[4]. When oxygen accepts two electrons and is incompletely reduced to hydrogen peroxide, it not only results in a decrease in energy conversion efficiency but also causes deactivation of catalysts and deterioration of devices through the attack of free radicals. In other words, we ought to develop ORR electrocatalysts with superior 4e- selectivity in order to achieve better performance in energy conversion systems.
Single-atom metal-nitrogen-carbon (M-N-C) catalysts, with atomically precisely designed active sites and conductive carbon frameworks, are promising candidate materials for usage in renewable energy devices[5,6]. The unique microstructure of M-N-C catalysts, facilitated by the coordination of transition metals with nitrogen in a carbon matrix, is central to their ORR activity. Further research revealed that the MN4 structure contributes most significantly to the reactivity of the 4e- process[7-9]. Nevertheless, this extensively studied scaffold lacks the optimal adsorption behavior for oxygen and oxygen-containing intermediates and is susceptible to deactivation during the operation of electrochemical devices. Therefore, optimizing the activity and stability of M-N-C catalysts via structural design and modification is of crucial significance in advancing the ORR performance.
The core of regulating the catalytic performance of M-N-C lies in the modification of the electronic structure, which is closely related to the metal center and its coordination environment[10]. In transition metal elements, Fe and Co exhibit superior intrinsic ORR activity over the other non-noble metals[11]. However, isolated MN4 sites struggle to match the performance of Pt/C, and it is difficult to achieve a balance between activity and stability. Consequently, numerous studies endeavored to modify the electronic structure by altering the coordination environment, aiming to optimize the interaction between the metal centers and oxygen-containing intermediates, and furthermore, address the trade-off issue. With intensive research focusing on the modification of the first coordination shell, such as the substitution of N in MN4 to other heteroatoms[12-14], the addition of axially coordinated atoms[15,16], etc., we highlight the significance of modification in the outer coordination shell which possesses a more delicate regulation effect.
Expanding the scope of modification beyond the first coordination sphere opens up a new dimension of possibilities. By adjusting coordination environment in the second or higher shells, it is plausible to exert a more subtle yet profound influence on the MN4 sites. In this review, we categorize the approaches for regulating MN4 in the high coordination layer as follows [Figure 1]: (1) Adjusting atomic entities, encompassing the impact of atoms in the second shell or dispersed in the carbon framework, as well as the intrinsic order on the activity-stability trade-off of MN4 sites; (2) Incorporating metallic species such as nanoclusters or nanoparticles to effectively modulate the electronic structure of MN4 and function as radical scavengers; and (3) Introducing non-metallic modulators, including atomic defects and functional groups, to enhance ORR activity at MN4 sites. We focus on exploring these outer coordination sphere modifications, aiming to unveil the full potential of M-N-C catalysts for ORR applications.
REGULATING ATOMIC ENTITIES BEYOND THE FIRST COORDINATION SHELL
In the local microstructure of heterogenous single-atom catalytic sites, it is crucial to define the configuration of coordination environment. For the outer shells of MN4, only the introduced atoms in the second shell can be accurately detected within the limits of current characterization methods[17]. However, atoms in the whole microenvironment surrounding MN4 exhibit potential to regulate active centers and thus it is momentous to explore the modulation method for meticulously tailoring the reactivity. In this section, we first discuss the introduced atomic entities altering original carbon atoms in the outer coordination shell, including second and higher shells’ atoms. On the other hand, the intrinsic order of local construction is deliberated, highlighting the inherent activity and stability exchange of catalytic sites.
Introducing the doped atoms
Designing atoms in the second shell
Atoms in the second coordination shell significantly affect the active center, and the introduction of other atomic entities disrupts the original electronic and geometric symmetry, thereby altering and optimizing the adsorption of ORR intermediates. Substituting light heteroatoms for the carbon atoms surrounding MN4 is a straightforward and efficient approach to modulate the electronic structure of the monometal center[18-20]. For instance, Qin et al. introduced S anions in the second coordination shell [Figure 2A], effectively manipulating the electronic structure of the central metal Ru[21]. X-ray absorption near-edge structure (XANES) fitting based on optimized density functional theory (DFT) models indicated unambiguously that S atoms were confirmed to coordinate with N atoms [Figure 2B and C]. The asymmetrical expansion from RuN4 to RuN4S weakened the adsorption of ORR intermediates at Ru sites, enhancing ORR performance with a half-wave potential (E1/2) of 0.861 V in alkaline media, surpassing that of Pt/C (0.853 V). Many studies have also demonstrated the positive impact of doping heteroatoms such as P[22], S[23] and O[24] into the second coordination layer of the central metal. Chen et al. elucidated the effect of S doping in the second coordination layer on the spin state of iron in FeN4 sites[23]. 57Fe Mössbauer spectroscopy demonstrated that neighboring S prominently increased the content of low-spin Fe3+ sites (D1) [Figure 2D], which possesses favorable adsorption strength for ORR intermediates owing to lowered eg filling[25]. This was also confirmed in the operando Mössbauer spectrum [Figure 2E]. Upon applying different voltages, D1 sites underwent the transition in the spin polarization, indicating its activation of O2 molecules as active sites. Further DFT calculations certified that the appropriate amount of S doping effectively facilitated the desorption process of OH* species, leading to an enhancement for the ORR catalytic activity. Zhang et al. distinguished structural differences between Co/Ni-centered and Fe-centered single atomic active sites[26]. The lower coordination tendency of Fe to S led to the preferential formation of the FeN4S2 structure during pyrolysis, whereas Co/Ni directly coordinated with single S atoms in the post-pyrolysis active sites [Figure 2F]. FeN4S2 exhibited superior ORR catalytic performance compared to Co/NiN3S1, which can be attributed to the higher charge density in the Fe center generated by the increment of S doping. However, this prompts inquiries regarding the dosage of dopants. Theoretically, the number and positioning of the doped atoms in the second shell matter as well, as the structural asymmetry affects charge depletion and accumulation to varying degrees, improving electron transport process[27]. In the research of Chang et al., double P doping generated excessive electron transfer from Fe/P to N species than single P doping [Figure 2G and H], resulting in stronger adsorption of OH*, which was unfavorable for the ORR process[28]. As for structural distortions and local strain induced by mismatching of atomic radius[29], however, the lack of accurate synthesis and characterization methods has limited research into the mechanism of strain effect as a result of different numbers and positions of dopants, necessitating further exploration.
Figure 2. (A) Schematic atomic model of asymmetrical expansion from RuN4 to RuN4S. Reproduced with permission[21]. Copyright 2022, American Chemical Society. Comparison of Ru K-edge XANES spectrum (B) and the E-space derivation (C) between the experimental and theoretical fitting. Reproduced with permission[21]. Copyright 2022, American Chemical Society. (D) Content of Fe moieties with varied spin polarization deriving from 57Fe Mössbauer spectroscopy. Reproduced with permission[23]. Copyright 2021, John Wiley and Sons. (E) The correlation between ORR overpotential (ηORR) and OH* adsorption free energy (ΔGOH*) on Fe moieties with distinct local configurations. Reproduced with permission[23]. Copyright 2021, John Wiley and Sons. (F) Schematic illustration of the formation of Fe single-atom sites with different numbers and locations of S doping. Reproduced with permission[26]. Copyright 2019, American Chemical Society. Charge density difference of Fe1N4P1 (G) and Fe1N4P2 (H). The brown, blue, and golden spheres represent carbon, nitrogen, and iron atoms, respectively. Reproduced with permission[28]. Copyright 2023, Elsevier.
Designing atoms in the higher shell
As the distance between introduced atomic entities and the active metal center increases, the level of interaction gradually diminishes. However, the so-called long-range interaction from the distant shells still exerts a significant impact on the reactivity of MN4 sites. From this perspective, one can design not only dispersed heteroatoms to facilitate electron transfer through the electronic “pull effect”[30], but also additional atomic sites to function as an electroactivity modulator or generate synergistic effect[31]. The former, apart from emphasizing the disruption of charge symmetry at single atomic sites[32], necessitates acknowledging the coordination field effects induced by light heteroatoms from higher shells. Yin et al. revealed heteroatom-driven cerium single-atom stabilization[33]. The long-range interaction of P and S sites enhances the electroactivity and site-to-site electron transfer while N stabilizes the Ce single atoms to form CeN4 sites. And the electronic modulation through the coordination field can be reflected in projected density of states (PDOS). It can be seen in Figure 3A and B that the incorporation of P and S guarantees the highest electroactivity and alleviates the electron transfer barrier with a proper ratio. Furthermore, the microenvironment of outer coordination shells was engineered by Li et al. through ternary heteroatomic doping[34]. At low iron loading (≈0.89 wt.%), N, F, S triple-doped hollow carbon nanofibers endow individual FeN4 site with high intrinsic activity, resulting in a remarkable ORR half-wave potential (0.90 V) in alkaline media. Therefore, coupling single-atom metal sites with a complex coordination environment is a promising approach to improve electronic mobility. Besides, single atomization of rare earth elements induced by heteroatomic coordination field broadens the boundaries of the ORR catalytic system.
Figure 3. PDOS of Ce-4f (A) and N-2p (B) with different S:P ratios in the coordination environment. Reproduced with permission[33]. Copyright 2023, John Wiley and Sons. (C) ηORR on CuN4 and Cu-Se pairs with varied distances. Reproduced with permission[35]. Copyright 2023, John Wiley and Sons. (D) The calculated ORR reaction paths for medium-distance Cu-Se pair. Reproduced with permission[35]. Copyright 2023, John Wiley and Sons. (E) Schematic of Fe-Co pair with bifunctional catalytic capability. Reproduced with permission[39]. Copyright 2022, John Wiley and Sons. Volcano plots of ORR (F) and OER (G) for varied configurations of Fe-Co pairs. Reproduced with permission[39]. Copyright 2022, John Wiley and Sons.
In terms of newly introduced atomic sites, the effect extends from the improvement of electron transfer to site interaction, and it prompts the exploration of appropriate spatial distance between interactional sites. Sun et al. built CuN4 surrounding with Se1C3 atomic sites and an optimal distance between Cu and Se was deemed to be 3.64 Å, which presented the least ORR overpotential than other distances [Figure 3C][35]. In this case, the synergistic catalytic path from OOH* in Cu1N4 to O* in Se1C2 could be regulated to the best [Figure 3D], corresponding to its outstanding performance. Similar trend in the existence of optimal distance between modulating atomic entities and the original active center was discovered in other research[36]. This puts forward higher requirements for accurate chemical synthesis methods. Furthermore, the synergy of site interaction might induce the bifunctional catalytic capability, which is highly desired for energy storage and conversion systems[37,38]. For instance, CoN4 and NiN4 sites were anchored adjacent to FeN4 for bifunctional oxygen electrocatalysis. Liu et al. discovered that CoN4 could optimize the ORR performance at FeN4 sites while exhibiting oxygen evolution reaction (OER) activity [Figure 3E][39]. By manipulating the configuration of bimetallic sites, the optimal adsorption of *O could be achieved, which attained both minimum overpotential for both ORR at Fe sites and OER at Co sites [Figure 3F and G]. The bifunctional oxygen electrocatalysis displayed a small potential gap (∆E) of 0.79 V, indicating its superior catalytic bifunctionality and electrode reversibility. Chen et al. constructed atomically dispersed Fe and Ni sides on the different sides of the hollow carbon nanosphere[40]. Similarly, FeN4 on the outer surface contributed to ORR activity and NiN4 on the inner surface is responsible for the OER process, respectively. These observations demonstrate the generation of multifunctional catalytic ability originates from the synergistic effect between the introduced single metal sites and ORR active centers. By fine-tuning the local structure or spatial orientation of bimetallic sites, both optimized ORR and OER activity can be achieved.
Adjusting the intrinsic order
Apart from engineering microenvironment of MN4 through introducing atomic entities in the outer shells, intrinsic order of atoms also emerges as a pivotal factor to regulate the inherent nature of active sites[41,42]. In the scope of M-N-C catalysts, intrinsic order is constructed by N and C atoms on the support with various heterocyclic structures, typically, i.e., the pyrrolic-, pyridinic-, and graphitic-N [Figure 4A]. Exploring the contribution of different N types to active sites is crucial for unraveling structure-performance relationships in M-N-C catalysts. And the current discourse primarily revolves around the discrepancy of MN4C12 (pyrrole-type) and MN4C10 (pyridine-type) as the origin of ORR activity[7,43-47]. Ni et al. attributed active sites in Fe-N-C catalysts to the iron center with pyrrolic N-coordination[48]. Employing operando Mössbauer spectroscopy, ORR intermediates attached to FeN4C12 were detected rigorously by distinct isomer shift δiso and quadrupole splitting ΔEQ [Figure 4B]. It was thereafter validated computationally by Hu et al. through constant-potential implicit solvent models that pyrrolic N contributes to a higher activity[49]. Besides, pH-dependence of active sites was interpreted as the variation in the affinity of the adsorbed species. As shown in [Figure 4C], pyridinic FeN4 adsorbed OH* too strongly at the high pH and low potential location while the alkaline environment further hindered the desorption process of OH*, indicating its inferior performance in alkali conditions. On the other hand, the rate-determining step for pyrrolic FeN4 underwent a transit from OH* desorption to O* protonation as pH increases, and pyrrolic FeN4 exhibited a more optimized adsorption strength for ORR intermediates across a wide potential range [Figure 4D]. Therefore, design for activity improvement for M-N-C should be aimed at increasing the content of pyrrolic N with promoted O* protonation in the alkaline and facilitated OH* desorption in the acid based on the above observations.
Figure 4. (A) Schematic illustration of pyrrolic-, pyridinic- and graphitic-N on the carbon support. (B) Identification of ORR intermediates adsorbed on pyrrole-type active sites derived from Mössbauer spectroscopy. Reproduced with permission[48]. Copyright 2022, American Chemical Society. (C) Contour plot showing adsorption energy of OH* on pyridinic FeN4 sites as a function of pH and potential. Reproduced with permission[49]. Copyright 2022, American Chemical Society. (D) Comparison of adsorption energy of ORR intermediates between pyrrole-type and pyridine-type active sites. Reproduced with permission[49]. (E) Coordination or structural changes of pyrrole-type and pyridine-type active sites under operando conditions. Reproduced with permission[53]. Copyright 2020, Springer Nature. (F) Comparison of ΔG of demetallation between pyrrolic FeN4 and monosymmetric FeN2 + N’2. Reproduced with permission[55]. (G) ORR activity of Fe-N-C catalysts prepared at different temperatures (600-1,100 °C). Reproduced with permission[57]. Copyright 2017, American Chemical Society. (H) Correlation between ORR activity and content of varied types of nitrogen altered with temperature. Reproduced with permission[57]. Copyright 2017, American Chemical Society.
For the construction of intrinsic order, it is imperative to not only consider the electroactivity but also prioritize long-term durability, since the severe activity loss of M-N-C catalysts (particularly in the acidic environment) limits their large-scale application in electrochemical devices[50]. Meanwhile, the trade-off between activity and stability remains a conundrum for M-N-C active site design[51,52]. The more intrinsically active pyrrolic FeN4 sites, however, are prone to deactivate during fuel cell operation, as identified by
Graphitic-N dispersed in the outer coordination shells, on the other hand, affects the single-atom active center akin to doped heteroatoms. Owing to a larger electronegativity than C, graphitic-N in the carbon sheet could induce electron deficiency in neighboring carbon atoms and reduce the energy gap between the Fermi level and conduction band, thereby enhancing the charge mobility of the graphene lattice[56]. Typically, the content of graphitic-N increases as the pyrolysis temperature elevates, and
INTRODUCING METALLIC SPECIES BEYOND THE FIRST COORDINATION SHELL
Introducing metallic species in proximity to MN4 sites is another emerging regulation method, which can effectively ameliorate the ORR performance of M-N-C. It should be noted that metallic species encompass metal nanoparticles and metal nanoclusters, ranging from a few atoms to hundreds or thousands of atoms. The scale effect leads to significant disparities in the geometry and electronic structure of nanoclusters and nanoparticles, resulting in a distinct interaction with single atomic sites[59]. In this section, we emphasize the modification of the electronic structure of MN4 by nanoclusters and the function of the nanoparticles as site stabilizers for alleviating deactivation of catalytic sites.
Incorporating nanoclusters as electronic modulator
The electronic structure of atomically dispersed metal atoms can be regulated through coupling with nanoclusters. Recently, the ORR performance of M-N-C has been enhanced by utilizing various types of clusters, including pure metal clusters[60-62], metallic carbides[63,64], nanoalloys[65,66], etc. For example,
Figure 5. (A) Schematic depiction of the catalytic function of PtN4, PtN4@Pt nanoclusters and Pt nanoclusters in the ORR process. Reproduced with permission[67]. Copyright 2024, American Chemical Society. (B) ORR free energy diagrams for PtN4 (redline),
In addition to pure metal clusters, the electronic structure of M-N-C can also be influenced by metallic carbides[71-73] and nanoalloys[66] in a similar manner. The incorporation of Fe/Fe3C nanocrystals was observed to significantly enhance the ORR performance of FeN4[72]. Afterward, many studies have further investigated the synergistic effects of Fe3C nanoclusters on the electronic structure of FeN4 sites[74,75], and have been extended to different systems, such as FeN4@Ti3C2[76], FeN4@Fe4C[71], MnN4@Fe3C[77], etc. Chang et al. utilized the seed-mediated strategy to fabricate Fe3C species that are closely surrounded by Fe-N4-C active sites[64]. By slightly adjusting the proportion of components, the optimal electrical interaction between Fe3C and FeN4 was achieved. Xu et al. synthesized a doped carbon skeleton with a unique structure via g-C3N4-assisted growing strategy, consisting of 1D nanotubes and 2D nanosheets[78]. FeN4 active sites coupled with Fe3C encapsulated in this hierarchical structure, which promoted both mass and electron transfer, demonstrated a strong synergistic effect and accelerated the oxygen adsorption kinetics. In addition, the utilization of nanoalloys is also a potent strategy for the modification of M-N-C. Wu et al. uniformly deposited PtFe nanoalloys onto the surface of FeN4 sites, where the combination of both acted as an electronic structure modulator[66]. As shown in Figure 5F, the differential charge density calculations reveal a notable accumulation of charges between FeN4 sites and PtFe nanoalloys, suggesting the presence of reciprocal electron transfer and electron modulation between them. The study conducted by
Incorporating nanoparticles as site stabilizer
As electronic structure of MN4 can be effectively regulated with assistance of nanoclusters, the stability and durability of M-N-C towards ORR, despite significant advancements in their activity, remains insufficient, particularly in the acidic environment[79,80]. Currently, the degradation mechanism is commonly believed to vary under different pH conditions[81]. In an acidic environment with relatively poor stability, MN4 site is susceptible to trigger Fenton or Fenton-like reaction with hydrogen peroxide (H2O2) during the 2-electron process[80,82,83]. The carbon matrix of catalysts could be irreversibly damaged by the aggressive reactive oxygen species (ROS), particularly hydroxyl radicals (·OH)[84]. On the other hand, in alkaline environments, the metal leaching from active sites during the ORR process becomes an inevitable factor contributing to activity decay and serves as the primary degradation mechanism. Therefore, it is imperative to eliminate ROS generated by side reaction and decelerate the leaching of metal ions to pursue superior ORR performance. As aforesaid, designing the intrinsic order enhances inherent resistance against degradation, while the incorporation of nanoparticles can function as site stabilizers from the outer coordination environment.
Introduction of radical scavengers into the carbon support is an effective strategy for enhancing the stability of ORR catalysts by rapidly eliminating ROS generated during the ORR process, and thus inhibiting Fenton or Fenton-like reactions. The functionality of radical scavenging has been well-recognized in nanoparticles, especially metal oxide nanoparticles[85,86]. Xie et al. employed the high-temperature pulse technique to synthesize Ta-TiOx modulating Fe-N-C catalysts[87]. Qualifying with a highly efficient capacity to scavenge oxygen radicals, dispersing Ta-TiOx nanoparticles throughout the carbon framework can effectively decelerate the attack of free radicals on the active site during the ORR process [Figure 6A]. Further DFT calculations proved that TaO2-OH had a higher affinity towards H2O2 and related radicals [Figure 6B], thereby preventing the destruction of active sites in Fe-N-C through competitive adsorption. The protected catalyst exhibited exceptional durability, which showed a current density decay of only 3% at 0.9 V after an accelerated durability test, whereas Fe-N-C alone decayed by 33% [Figure 6C]. In addition, cerium dioxide (CeO2) has also been found to be a proficient scavenger of free radicals[88,89]. ROS formed at MN4 sites, such as ·OH and HO2·, can be rapidly captured and eliminated by CeO2 nanoparticles. Increasing the dispersion and reducing the size of the nanoparticles is important because scavengers adjacent to the catalytic active sites can simultaneously eliminate the generated ROS, thereby reducing the survival time of radicals and limiting the spatial extent of their damaging effects [Figure 6D]. To sum up, the stability of ORR in M-N-C can be significantly improved with the modification of radical scavengers. This strategy extends the protection of M-N-C from passive shielding to active defense.
Figure 6. (A) Schematic illustration of carbon substrate well-dispersing with nanoparticle scavengers. Reproduced with permission[87]. Copyright 2022, Springer Nature. (B) The size distribution of the Ta-TiOx nanoparticles. Reproduced with permission[87]. Copyright 2022, Springer Nature. (C) Adsorption affinity of H2O2 and derived free radical on the Fe-N-C and TaO2-OH surfaces. Copyright 2022, Springer Nature. Reproduced with permission[87]. (D) Schematic depiction of CeO2 nanoparticles scavenging radicals and their elimination zone. Reproduced with permission[89]. Copyright 2023, John Wiley and Sons. (E) Long-term stability test of FeN4 with Pt nanoparticles at a constant current density of 0.1 A cm-2. Reproduced with permission[90]. Copyright 2023, American Chemical Society. (F) HAADF-STEM image of atomically dispersed Pt, Fe sites and Pt-Fe alloy nanoparticles. Reproduced with permission[93]. Copyright 2022, Springer Nature. (G) H2/O2 fuel cell polarization and power density plots of the Pt-Fe-N-C with Pt-Fe nanoalloys before and after 100,000 potential cycles between 0.6 and 0.95 V. Reproduced with permission[93]. Copyright 2022, Springer Nature.
Instead of acting as radical scavengers, nanoparticles can also strengthen M-N bonds through electronic interaction to prohibit demetallation. Gao et al. investigated the impact of different types, forms, and contents of metal nanoparticles on M-N bond strength[90]. The underlying mechanism goes that nanoparticles, acting as electron donors, can augment the electron density of the MN4 site, thereby enhancing the M-N bond and impeding metal dissolution. In their work, Fe-N-C@Pt nanoparticles were found to be the most stable among all synthesized M-N-C materials and in the direct methanol fuel cell stability test, the cell voltage experienced a rapid decrease in the first 10 h and then remained stable in subsequent 430 h, which excess most of the ORR catalysts nowadays [Figure 6E]. Pt species are consequently deemed to be an excellent stabilizer for single-atom metal sites[91,92]. Furthermore, in Xiao’s research, the stability of Fe-N-C was improved greatly after hybridization with Pt single atoms and Pt-Fe alloy nanoparticles[93]. The simultaneous presence of dispersed single atoms and nanoparticles was distinctly observed in high-angle annular dark-field scanning transmission electron microscopy (HAADF-STEM) [Figure 6F]. Synergistic effects among active sites in hybrid electrocatalysts effectively hindered the dissolution of iron, thus mitigating the degradation of membranes and ionomers in fuel cells. As a result, 97% activity retention was achieved after 100,000 potential cycles [Figure 6G].
TAILORING NON-METALLIC MODULATORS BEYOND THE FIRST COORDINATION SHELL
Defects and functional groups are inevitable formation components during the synthesis process of heterogenous catalysts, and the development of precise design strategies to augment the active sites merits comprehensive investigation. Atomic defects facilitate the electron mobility and the accessibility between MN4 sites and reactants, which is advantageous for accelerative mass transfer and reduced internal ohmic resistance[94,95]. Additionally, functional groups can be constructed purposely and systematically to explore the favored active sites associated with specific local states[96]. Through meticulous design of these non-metallic modulators, it is plausible to optimize the electronic structure of the single-atom active sites.
Atomic defect modification
The significance of defects in improving catalytic efficiency is well-recognized. Vacancies are a common kind of defect in carbon-based materials[97]. These coordination vacancies can modulate the electronic structure from the higher coordination shell, thereby optimizing the behavior of reactive intermediates. Significant focus has been placed on enhancing the efficiency of M-N-C catalysts for ORR through optimized atomic vacancies, including Fe-N-C with carbon vacancies[54], Zn-N-C with nitrogen vacancies[98], Co-N-C with carbon vacancies[99], and so on. Specifically, M-N-C with atomic-level carbon vacancies (M=Fe, Co, Ni, Cu, and Mn) was synthesized by Tian et al., aiming to precisely control the chemical environment and electronic configuration of the metal center[100]. Fe-N-C with well-defined electronic structure exhibited superior ORR activity and stability compared with commercial Pt/C and other reference samples. To further explain the reason, the authors considered six possible single carbon vacancies, as shown in Figure 7A. By calculating the Gibbs free energy change on the defective FeN4 along the 4e ORR process, site E with a carbon vacancy in the fourth coordination shell was found to be most conducive to the process of the potential-determining step, that is, the reduction of OH* [Figure 7B].
Figure 7. (A) The atomic structures of FeN4 with six single carbon vacancies. Reproduced with permission[100]. Copyright 2023, John Wiley and Sons. (B) The free energy diagrams of ORR on FeN4 with six single carbon vacancies. Reproduced with permission[100]. Copyright 2023, John Wiley and Sons. (C) Variants of FeN4 with different divacancy defect models. Reproduced with permission[101]. Copyright 2022, Springer Nature. (D) Adsorption energy of O2, O-O, and Fe-O bond length on FeN4 with different divacancy structures. Reproduced with permission[101]. Copyright 2022, Springer Nature. HOMO and LUMO distributions of the center-N-modified divacancies trapped Fe sites (E) and edge-N-modified divacancies trapped Fe sites (F) models. Reproduced with permission[102]. Copyright 2020, John Wiley and Sons.
The edge-site engineering of MN4 site has also aroused intensive research[102-104]. Reconfigured by the mesoporous structure, the MN4 moiety located at the edge exhibited a favorable energy profile thermodynamically compared to the intact atomic configuration, leading to enhanced catalytic performance[105]. Besides, the design based on the edge increases the accessibility and the loading of MN4 sites[102]. Cui et al. constructed edge-rich single-atomic Cu-N4 sites by NH4Cl-assisted etching strategy[106]. The introduced NH4Cl promoted the formation of abundant carbon edge, which was preferential for the anchoring of Cu sites. Impressively, the as-synthesized Cu-N-C exhibited a high E1/2 of 0.91 V [vs. reversible hydrogen electrode (RHE)] and onset potential of 1.02 V (vs. RHE), respectively, surpassing the Pt/C benchmark and ranking among the top-performing electrocatalysts. Wang et al. further demonstrated the electron redistribution of edge sites in defective carbon[102]. According to DFT calculations, FeN4 anchored at the edge exhibited a higher electron density, indicating greater electron donor capacity than those anchored on the intact carbon basal plane. And a narrower highest-occupied molecular orbital (HOMO)-lowest-unoccupied molecular orbital (LUMO) gap could generate rapid electron transfer in the catalytic reactions
Functional group modification
The modification of single atomic sites through the functional groups in the high coordination sphere is also a crucial approach for regulating the performance of ORR. The electronic structure of the catalytic sites can be systematically modulated by the electron-withdrawing or electron-donating effect imparted by functional groups, thereby effectively regulating the adsorption/desorption behavior of reaction intermediates[107]. The efficacy of the functional group modification has been well demonstrated in M-N-C catalysts, such as epoxy modifying Sb-N-C[108], Cu-N-C with carboxyl groups[109], hydroxyl-rich Co-N-C[110], etc. Recently, Wei et al. explored the influence of the functional group and local structure on the electronic properties of Co centers[111]. Planar conjugated structures with different functional groups [amino (-NH2), hydroxyl (-OH), and sulfhydryl (-SH)] were used as the skeleton. And it is noteworthy that a higher magnetic moment (1.12 μB) was observed when Co site bridged triphenylene with hydroxyl functional group, indicating a transition from low-spin to high-spin states for electron filling of eg orbitals
Figure 8. (A) Bader charge transfer and magnetic moments of Co sites coupled with different local structures. Reproduced with permission[111]. Copyright 2023, American Chemical Society. (B) Reconfiguration of spin state in the Co center with a high magnetic moment. Reproduced with permission[111]. Copyright 2023, American Chemical Society. (C) 2D volcano plot of ηORRvs. ICOHP of Co-O bonds between Co sites and OH* species. Reproduced with permission[111]. Copyright 2023, American Chemical Society. (D) Schematic diagram of FeN4 with P-O bond in different outer shells. Reproduced with permission[112]. Copyright 2023, American Chemical Society. (E) Top: Contour plots showing the charge density difference after *OOH adsorption; bottom: differential charge densities upon *OOH adsorption. Reproduced with permission[112]. Copyright 2023, American Chemical Society. (F) Schematic illustration of selectivity shift and active site shift after the decoration of C-O-C groups. Reproduced with permission[113]. Copyright 2021, American Chemical Society. (G) LSV curves for Co-N-C with C-O-C groups before and after the addition of 10 mM KSCN. Reproduced with permission[113]. Copyright 2021, American Chemical Society. (H)In situ ATR-SEIRAS spectra of Co-N-C with C-O-C groups and the comparison of the band around 1220 cm-1 for Co-N-C with C-O-C groups and Co-N-C. Reproduced with permission[113]. Copyright 2021, American Chemical Society.
CONCLUSION AND OUTLOOK
This review summarizes the methods of modulating single atomic sites beyond the first coordination shell, focusing on the effects of adjusting atomic entities, metallic and non-metallic modulators in the vicinity of MN4. Compared to direct coordination with single atoms, regulation beyond the first coordination shell has a more moderate control scale and can produce a more optimized electronic structure through synergistic interactions. Adjusting atomic entities could give rise to unsymmetric electronic distribution of MN4 sites and generate synergistic effects through site interaction while manipulating the intrinsic order of single atoms which can selectively control the balance between activity and stability of active sites. The incorporation of metallic species such as nanoclusters or nanoparticles significantly adjusts the electronic structure of single-atom centers, optimizing the hybridization of metal 3d orbitals with oxygen 2p orbitals, thereby reducing the reaction barrier for ORR and producing superior performance. On the other hand, the specific configuration of the MN4 sites with the metallic species may also change the oxygen adsorption pattern (i.e., from end-on to side-on) to promote O-O bond cleavage. In addition to serving as electronic modulators, functional nanoparticles can also act as stabilizers to enhance the stability of MN4 sites, which is reflected in rapid removal of free radicals generated during ORR process to inhibit Fenton reactions; moreover, interaction between nanoparticles and MN4 sites can strengthen M-N bonds to avoid demetallation. Introducing non-metallic modulators into high coordination layers is also an important way to regulate MN4 sites. Defects can increase site exposure and adjust adsorption energy for oxygen intermediates while functional groups mainly interact with MN4 sites through electron-donating/electron-withdrawing effects. The performance of ORR electrocatalysts modified by the above methods is summarized in Table 1.
Summary of M-N-C ORR performance with different modifications
Regulation method | Active site | Electrolyte | Eonset (V vs. RHE) | E1/2 (V vs. RHE) | Kinetic current (mA cm-2) | Ref. |
Atomic entities | CoN4-S2 2nda | 0.1 M KOH | 1.00 | 0.87 | 35.9@0.8 V | [26] |
RuN4-S1 2nd | 0.1 M KOH | 0.998 | 0.861 | 101.7@0.8 V | [21] | |
FeN4S1-P1 2nd | 0.1 M HClO4 | NA | 0.814 | 46.7@0.7 V | [22] | |
0.1 M KOH | NA | 0.912 | 60@0.85 V | |||
FeN4-P2 2nd | 0.1 M KOH | 1.01 | 0.90 | 25.4@0.85 V | [27] | |
FeN4-S1hs b | 0.1 M HClO4 | 0.92 | 0.821 | 6.7@0.5 V | [114] | |
0.1 M KOH | 1.05 | 0.887 | 7.1@0.5 V | |||
FeN4-Cl1hs | 0.1 M KOH | 1.02 | 0.917 | 23.8@0.85 V | [32] | |
CeN4-Phs-Shs | 0.1 M KOH | NA | 0.90 | 81.1@0.85 V | [33] | |
FeN4@ZrN4 | 0.1 M HClO4 | NA | 0.872 | 43.48@0.8 V | [115] | |
FeN4@MoN4 | 0.1 M HClO4 | 0.98 | 0.84 | 23.5@0.8 V | [116] | |
CuN4@Se1C3 | 0.1 M KOH | 1.12 | 0.905 | 25.6@0.85 V | [35] | |
SbN2C2@Se1C2 | 0.1 M KOH | NA | 0.865 | 7.424@0.85 V | [36] | |
FeN4@NiN4 | 0.1 M KOH | 0.93 | 0.83 | NA | [40] | |
Metallic species | FeN4@Fe7 ACc | 0.1 M KOH | 1.02 | 0.894 | 15.91@0.85 V | [61] |
PtN4@Ptx AC | 0.1 M HClO4 | NA | 0.87 | 9@0.85 V | [67] | |
CoN4@Cox AC | 0.1 M KOH | NA | 0.89 | 21.64@0.85 V | [117] | |
FeN4@Co4 AC | 0.5 M H2SO4 | 0.980 | 0.835 | NA | [69] | |
MnN4@Mnx AC | 0.1 M KOH | 0.97 | 0.90 | 56.44@0.85 V | [70] | |
MnN4@Fe-Mn AC | 0.5 M H2SO4 | 0.89 | 0.79 | 7.27@0.7 V | [118] | |
0.1 M KOH | 1.00 | 0.90 | 7.71@0.7 V | |||
FeN4@Cux AC | 0.1 M HClO4 | 0.96 | 0.83 | 11.9@0.8 V | [62] | |
0.1 M PBS | 1.02 | 0.84 | 68@0.7 V | |||
0.1 M KOH | 1.06 | 0.94 | 145.8@0.85 V | |||
FeN4@Fe4C AC | 0.5 M H2SO4 | NA | 0.836 | 14.79@0.8 V | [71] | |
FeN4@Ti3N2 AC | 0.1 M KOH | 1.00 | 0.92 | NA | [76] | |
MnN4@Pt NPd | 0.1 M HClO4 | 1.01 | 0.93 | NA | [119] | |
FeN4@Fe-CeO2 NP | 0.1 M KOH | NA | 0.948 | 35.15@0.9 V | [120] | |
Pt/FeN4@Pt-Fe NP | 0.1 M HClO4 | NA | 0.909 | NA | [93] | |
Non-metallic species | FeN4-CVe | 0.5 M H2SO4 | NA | 0.915 | 10.3@0.9 V | [54] |
SbN4-CV | 0.1 M KOH | NA | 0.905 | 6.74@0.9 V | [121] | |
CoN4-CV | 0.1 M KOH | 0.99 | 0.878 | 12.39@0.85 V | [99] | |
FeN4-NVf | 0.1 M KOH | 0.990 | 0.902 | 51.7@0.85 V | [122] | |
FeN4@P-O | 0.1 M KOH | 1.01 | 0.912 | 7.54@0.9 V | [112] | |
FeN4@C-O-C | 0.1 M KOH | 1.08 | 0.92 | 51.75@0.85 V | [24] | |
FeN4@oxidized S | 0.5 M H2SO4 | 0.88 | 0.76 | 6.6@0.85 V | [123] | |
0.1 M KOH | 1.01 | 0.91 | NA | |||
SbN4@C-O-C | 0.1 M KOH | 1.03 | 0.86 | 24@0.82 V | [108] |
Although extensive research has been conducted on the regulation methods of M-N-C catalysts, they still encounter obstacles in achieving widespread commercial application. Therefore, we propose the following points to illustrate the future development trend of M-N-C materials.
In situ accurate design
In the realm of ORR catalysts, the advent of M-N-C represents a paradigm shift, offering a new dimension of catalytic efficiency and specificity. This review summarizes the design of modification of M-N-C beyond the first coordination shell, but achieving accurate in situ control remains a challenge. The meticulous approach necessitates not only innovative design, but also a profound comprehension of the microscopic atomic scale, thereby imposing exceedingly high demands on in situ characterization methods. Through further development of advanced characterization techniques such as in situ Mössbauer spectroscopy and in situ XAS, combined with DFT calculations, researchers can establish precise atomic-scale structure-activity relationships. This is advantageous for the in situ design of micro-local configurations. By manipulating the electronic and geometric structure at the atomic scale, researchers can create catalysts that are finely tuned for specific reaction steps, minimizing unwanted byproducts and maximizing energy transformation. This level of control is pivotal for the development of next-generation catalysts that can drive chemical processes with unparalleled precision.
Large-scale production
The transition from laboratory-scale production to large-scale preparation of M-N-C is a significant leap toward their commercialization and practical application. This scale-up is crucial for meeting the demands of industrial processes, which require robust and consistent performance over extended periods. However, the large-scale preparation of atomically dispersed M-N-C ORR electrocatalysts remains a challenge, closely related to unavoidable thermal annealing, atom aggregation and many other issues. Recent advancements have led to the development of innovative synthesis techniques that maintain the integrity and dispersion of single atoms on various substrates[124]. By integrating these scalable approaches, the gap between the potential and practical use of M-N-C is narrowing, setting the stage for their widespread adoption in catalysis-driven industries.
Computational high-throughput screening
High-throughput screening for electrocatalysts has emerged as a powerful tool in the discovery and optimization of M-N-C catalysts. By leveraging advanced algorithms and machine learning techniques, researchers can rapidly evaluate a vast array of potential catalyst structures and compositions. This approach also significantly accelerates the identification of promising candidates by predicting their performance and stability with various catalytic reactivity descriptors[125]. High-throughput screening not only reduces the time and cost associated with experimental trials but also provides valuable insights into the fundamental principles governing catalytic activity. By integrating computational methods with experimental validation, researchers can systematically explore the vast design space of M-N-C catalysts, leading to the development of highly efficient and durable materials. This synergy between computation and experimentation is essential for the rational design of next-generation ORR catalysts, paving the way for their practical application in energy conversion and storage technologies.
DECLARATIONS
Authors’ contributions
Proposed the topic of this review: Kou Z
Wrote the manuscript: Zhang D, Zhang X
Designed and drew the figures: Zhang D, Li X, Zhang X
Collectively discussed and revised the manuscript: Zhang D, Li X, Zhang X, Feng C, Kou Z, Chu Y, Chen C
Availability of data and materials
Not applicable.
Financial support and sponsorship
The work is financially supported by the Natural Science Foundation of Hubei Province (Grant No.2022CFB388), Natural Science Foundation of Hainan Province of China (Grant No.623MS068), National College Student Innovation and Entrepreneurship Training Program (S202310497121).
Conflicts of interest
All authors declared that there are no conflicts of interest.
Ethical approval and consent to participate
Not applicable.
Consent for publication
Not applicable.
Copyright
© The Author(s) 2025.
REFERENCES
1. Wang, X. X.; Swihart, M. T.; Wu, G. Achievements, challenges and perspectives on cathode catalysts in proton exchange membrane fuel cells for transportation. Nat. Catal. 2019, 2, 578-89.
2. Xia, Q.; Zhai, Y.; Zhao, L.; et al. Carbon-supported single-atom catalysts for advanced rechargeable metal-air batteries. Energy. Mater. 2022, 2, 200015.
3. Staffell, I.; Scamman, D.; Velazquez, A. A.; et al. The role of hydrogen and fuel cells in the global energy system. Energy. Environ. Sci. 2019, 12, 463-91.
5. Gong, X.; Song, P.; Han, C.; Xiao, Y.; Mei, X.; Xu, W. Heterogeneous single-atom catalysts for energy process: recent progress, applications and challenges. Energy. Mater. 2023, 3, 300016.
6. Li, Z.; Yang, C.; Xu, B.; Yao, L.; Zhu, W.; Cui, Y. Electrochemically nitrate remediation by single-atom catalysts: advances, mechanisms, and prospects. Energy. Mater. 2024, 4, 400046.
7. Zitolo, A.; Goellner, V.; Armel, V.; et al. Identification of catalytic sites for oxygen reduction in iron- and nitrogen-doped graphene materials. Nat. Mater. 2015, 14, 937-42.
8. Jia, Q.; Ramaswamy, N.; Hafiz, H.; et al. Experimental observation of redox-induced Fe-N switching behavior as a determinant role for oxygen reduction activity. ACS. Nano. 2015, 9, 12496-505.
9. Wu, F.; Pan, C.; He, C. T.; et al. Single-atom Co-N4 electrocatalyst enabling four-electron oxygen reduction with enhanced hydrogen peroxide tolerance for selective sensing. J. Am. Chem. Soc. 2020, 142, 16861-7.
10. Li, Z.; Ji, S.; Xu, C.; et al. Engineering the electronic structure of single-atom iron sites with boosted oxygen bifunctional activity for zinc-air batteries. Adv. Mater. 2023, 35, e2209644.
11. He, Y.; Wu, G. PGM-free oxygen-reduction catalyst development for proton-exchange membrane fuel cells: challenges, solutions, and promises. ACC. Mater. Res. 2022, 3, 224-36.
12. Ji, S.; Jiang, B.; Hao, H.; et al. Matching the kinetics of natural enzymes with a single-atom iron nanozyme. Nat. Catal. 2021, 4, 407-17.
13. Li, X.; Yang, X.; Liu, L.; et al. Chemical vapor deposition for N/S-doped single Fe site catalysts for the oxygen reduction in direct methanol fuel cells. ACS. Catal. 2021, 11, 7450-9.
14. Zhou, S.; Chen, C.; Xia, J.; et al. FeN3S1-OH single-atom sites anchored on hollow porous carbon for highly efficient pH-universal oxygen reduction reaction. Small 2024, 20, e2310224.
15. Peng, L.; Yang, J.; Yang, Y.; et al. Mesopore-rich Fe-N-C catalyst with FeN4-O-NC single-atom sites delivers remarkable oxygen reduction reaction performance in alkaline media. Adv. Mater. 2022, 34, e2202544.
16. Zhao, K.; Liu, S.; Li, Y.; et al. Insight into the mechanism of axial ligands regulating the catalytic activity of Fe-N4 sites for oxygen reduction reaction. Adv. Energy. Mater. 2022, 12, 2103588.
17. Sarma, B. B.; Maurer, F.; Doronkin, D. E.; Grunwaldt, J. D. Design of single-atom catalysts and tracking their fate using operando and advanced X-ray spectroscopic tools. Chem. Rev. 2023, 123, 379-444.
18. Chen, Y.; Ji, S.; Zhao, S.; et al. Enhanced oxygen reduction with single-atomic-site iron catalysts for a zinc-air battery and hydrogen-air fuel cell. Nat. Commun. 2018, 9, 5422.
19. Yin, H.; Yuan, P.; Lu, B.; et al. Phosphorus-driven electron delocalization on edge-type FeN4 active sites for oxygen reduction in acid medium. ACS. Catal. 2021, 11, 12754-62.
20. Wang, L.; Tian, W. W.; Zhang, W.; Yu, F.; Yuan, Z. Y. Boosting oxygen electrocatalytic performance of Cu atom by engineering the d-band center via secondary heteroatomic phosphorus modulation. Appl. Catal. B. Environ. 2023, 338, 123043.
21. Qin, J.; Liu, H.; Zou, P.; Zhang, R.; Wang, C.; Xin, H. L. Altering ligand fields in single-atom sites through second-shell anion modulation boosts the oxygen reduction reaction. J. Am. Chem. Soc. 2022, 144, 2197-207.
22. Liu, J.; Chen, W.; Yuan, S.; Liu, T.; Wang, Q. High-coordination Fe-N4SP single-atom catalysts via the multi-shell synergistic effect for the enhanced oxygen reduction reaction of rechargeable Zn-air battery cathodes. Energy. Environ. Sci. 2024, 17, 249-59.
23. Chen, Z.; Niu, H.; Ding, J.; et al. Unraveling the origin of sulfur-doped Fe-N-C single-atom catalyst for enhanced oxygen reduction activity: effect of iron spin-state tuning. Angew. Chem. Int. Ed. 2021, 60, 25404-10.
24. Zhao, Y.; Shen, Z.; Huo, J.; et al. Epoxy-rich Fe single atom sites boost oxygen reduction electrocatalysis. Angew. Chem. Int. Ed. 2023, 62, e202308349.
25. Shen, G.; Zhang, R.; Pan, L.; et al. Regulating the spin state of FeIII by atomically anchoring on ultrathin titanium dioxide for efficient oxygen evolution electrocatalysis. Angew. Chem. Int. Ed. 2020, 59, 2313-7.
26. Zhang, J.; Zhao, Y.; Chen, C.; et al. Tuning the coordination environment in single-atom catalysts to achieve highly efficient oxygen reduction reactions. J. Am. Chem. Soc. 2019, 141, 20118-26.
27. Zhou, Y.; Lu, R.; Tao, X.; et al. Boosting oxygen electrocatalytic activity of Fe-N-C catalysts by phosphorus incorporation. J. Am. Chem. Soc. 2023, 145, 3647-55.
28. Chang, H.; Guo, Y. F.; Liu, X.; Wang, P. F.; Xie, Y.; Yi, T. F. Dual MOF-derived Fe/N/P-tridoped carbon nanotube as high-performance oxygen reduction catalysts for zinc-air batteries. Appl. Catal. B. Environ. 2023, 327, 122469.
29. Huang, Z. F.; Song, J.; Dou, S.; Li, X.; Wang, J.; Wang, X. Strategies to break the scaling relation toward enhanced oxygen electrocatalysis. Matter 2019, 1, 1494-518.
30. Han, J.; Tan, H.; Guo, K.; et al. The “pull effect” of a hanging ZnII on improving the four-electron oxygen reduction selectivity with Co porphyrin. Angew. Chem. Int. Ed. 2024, 63, e202409793.
31. Wang, Z.; Cheng, M.; Liu, Y.; et al. Dual-atomic-site catalysts for molecular oxygen activation in heterogeneous thermo-/electro-catalysis. Angew. Chem. Int. Ed. 2023, 62, e202301483.
32. Wei, S.; Yang, R.; Wang, Z.; Zhang, J.; Bu, X. H. Planar chlorination engineering: a strategy of completely breaking the geometric symmetry of Fe-N4 site for boosting oxygen electroreduction. Adv. Mater. 2024, 36, e2404692.
33. Yin, L.; Zhang, S.; Sun, M.; Wang, S.; Huang, B.; Du, Y. Heteroatom-driven coordination fields altering single cerium atom sites for efficient oxygen reduction reaction. Adv. Mater. 2023, 35, 2302485.
34. Li, H.; Zhao, H.; Yan, G.; et al. Ternary heteroatomic doping induced microenvironment engineering of low Fe-N4-loaded carbon nanofibers for bifunctional oxygen electrocatalysis. Small 2024, 20, e2304844.
35. Sun, Z.; Zhang, H.; Cao, L.; et al. Understanding synergistic catalysis on Cu-Se dual atom sites via operando X-ray absorption spectroscopy in oxygen reduction reaction. Angew. Chem. Int. Ed. 2023, 62, e202217719.
36. Niu, Z.; Lu, Z.; Qiao, Z.; et al. Long-range regulation of Se doping for oxygen reduction of atomically dispersed Sb catalysts for ultralow-temperature solid-state Zn-air batteries. ACS. Catal. 2023, 13, 7122-31.
37. Wang, Q.; Kaushik, S.; Xiao, X.; Xu, Q. Sustainable zinc-air battery chemistry: advances, challenges and prospects. Chem. Soc. Rev. 2023, 52, 6139-90.
38. Liu, S.; Wang, Z.; Zhou, S.; et al. Metal-organic-framework-derived hybrid carbon nanocages as a bifunctional electrocatalyst for oxygen reduction and evolution. Adv. Mater. 2017, 29, 1700874.
39. Liu, M.; Li, N.; Cao, S.; et al. A “pre-constrained metal twins” strategy to prepare efficient dual-metal-atom catalysts for cooperative oxygen electrocatalysis. Adv. Mater. 2022, 34, 2107421.
40. Chen, J.; Li, H.; Fan, C.; et al. Dual single-atomic Ni-N4 and Fe-N4 sites constructing janus hollow graphene for selective oxygen electrocatalysis. Adv. Mater. 2020, 32, 2003134.
41. Guo, D.; Shibuya, R.; Akiba, C.; Saji, S.; Kondo, T.; Nakamura, J. Active sites of nitrogen-doped carbon materials for oxygen reduction reaction clarified using model catalysts. Science 2016, 351, 361-5.
42. Yu, W.; Huang, H.; Qin, Y.; et al. The synergistic effect of pyrrolic-N and pyridinic-N with Pt under strong metal-support interaction to achieve high-performance alkaline hydrogen evolution. Adv. Energy. Mater. 2022, 12, 2200110.
43. Zitolo, A.; Ranjbar-Sahraie, N.; Mineva, T.; et al. Identification of catalytic sites in cobalt-nitrogen-carbon materials for the oxygen reduction reaction. Nat. Commun. 2017, 8, 957.
44. Zhang, N.; Zhou, T.; Chen, M.; et al. High-purity pyrrole-type FeN4 sites as a superior oxygen reduction electrocatalyst. Energy. Environ. Sci. 2020, 13, 111-8.
45. Yang, H.; Gao, S.; Rao, D.; Yan, X. Designing superior bifunctional electrocatalyst with high-purity pyrrole-type CoN4 and adjacent metallic cobalt sites for rechargeable Zn-air batteries. Energy. Storage. Mater. 2022, 46, 553-62.
46. Ha, Y.; Fei, B.; Yan, X.; et al. Atomically dispersed Co-pyridinic N-C for superior oxygen reduction reaction. Adv. Energy. Mater. 2020, 10, 2002592.
47. Li, L.; Wen, Y.; Han, G.; et al. Tailoring the stability of Fe-N-C via pyridinic nitrogen for acid oxygen reduction reaction. Chem. Eng. J. 2022, 437, 135320.
48. Ni, L.; Gallenkamp, C.; Wagner, S.; Bill, E.; Krewald, V.; Kramm, U. I. Identification of the catalytically dominant iron environment in iron- and nitrogen-doped carbon catalysts for the oxygen reduction reaction. J. Am. Chem. Soc. 2022, 144, 16827-40.
49. Hu, X.; Chen, S.; Chen, L.; et al. What is the real origin of the activity of Fe-N-C electrocatalysts in the O2 reduction reaction? Critical roles of coordinating pyrrolic N and axially adsorbing species. J. Am. Chem. Soc. 2022, 144, 18144-52.
50. Gu, Y.; Nie, N.; Liu, J.; et al. Enriching H2O through boron nitride as a support to promote hydrogen evolution from non-filtered seawater. EcoEnergy 2023, 1, 405-13.
51. Liu, S.; Shi, Q.; Wu, G. Solving the activity-stability trade-off riddle. Nat. Catal. 2021, 4, 6-7.
52. Cui, L.; Zhao, X.; Xie, H.; Zhang, Z. Overcoming the activity-stability trade-off in heterogeneous electro-Fenton catalysis: encapsulating carbon cloth-supported iron oxychloride within graphitic layers. ACS. Catal. 2022, 12, 13334-48.
53. Li, J.; Sougrati, M. T.; Zitolo, A.; et al. Identification of durable and non-durable FeNx sites in Fe-N-C materials for proton exchange membrane fuel cells. Nat. Catal. 2021, 4, 10-9.
54. Liu, S.; Li, C.; Zachman, M. J.; et al. Atomically dispersed iron sites with a nitrogen-carbon coating as highly active and durable oxygen reduction catalysts for fuel cells. Nat. Energy. 2022, 7, 652-63.
55. Bai, J.; Zhao, T.; Xu, M.; et al. Monosymmetric Fe-N4 sites enabling durable proton exchange membrane fuel cell cathode by chemical vapor modification. Nat. Commun. 2024, 15, 4219.
56. Wang, W.; Jia, Q.; Mukerjee, S.; Chen, S. Recent insights into the oxygen-reduction electrocatalysis of Fe/N/C materials. ACS. Catal. 2019, 9, 10126-41.
57. Zhang, H.; Hwang, S.; Wang, M.; et al. Single atomic iron catalysts for oxygen reduction in acidic media: particle size control and thermal activation. J. Am. Chem. Soc. 2017, 139, 14143-9.
58. Ni, B.; Shen, P.; Zhang, G.; et al. Second-shell N dopants regulate acidic O2 reduction pathways on isolated Pt sites. J. Am. Chem. Soc. 2024, 146, 11181-92.
59. Liu, L.; Corma, A. Metal catalysts for heterogeneous catalysis: from single atoms to nanoclusters and nanoparticles. Chem. Rev. 2018, 118, 4981-5079.
60. Lu, J.; Lu, Q.; Guo, Y.; et al. Cobalt atom-cluster interactions synergistically enhance the activity of oxygen reduction reaction in seawater. Energy. Storage. Mater. 2024, 65, 103093.
61. Wang, Z.; Lu, Z.; Ye, Q.; et al. Construction of Fe nanoclusters/nanoparticles to engineer FeN4 sites on multichannel porous carbon fibers for boosting oxygen reduction reaction. Adv. Funct. Mater. 2024, 34, 2315150.
62. Liang, C.; Han, X.; Zhang, T.; et al. Cu nanoclusters accelerate the rate-determining step of oxygen reduction on Fe-N-C in all pH range. Adv. Energy. Mater. 2024, 14, 2303935.
63. Chen, Y.; Kong, X.; Wang, Y.; et al. A binary single atom Fe3C|Fe-N-C catalyst by an atomic fence evaporation strategy for high performance ORR/OER and flexible zinc-air battery. Chem. Eng. J. 2023, 454, 140512.
64. Chang, J.; Zhang, Q.; Yu, J.; et al. A Fe single atom seed-mediated strategy toward Fe3C/Fe-N-C catalysts with outstanding bifunctional ORR/OER activities. Adv. Sci. 2023, 10, e2301656.
65. Zhu, W.; Pei, Y.; Douglin, J. C.; et al. Multi-scale study on bifunctional Co/Fe-N-C cathode catalyst layers with high active site density for the oxygen reduction reaction. Appl. Catal. B. Environ. 2021, 299, 120656.
66. Wu, Y.; Chen, L.; Geng, S.; et al. PtFe nanoalloys supported on Fe-based cubic framework as efficient oxygen reduction electrocatalysts for proton exchange membrane fuel cells. Adv. Funct. Mater. 2024, 34, 2307297.
67. Wang, F.; Yang, J.; Li, J.; et al. Which is best for ORR: single atoms, nanoclusters, or coexistence? ACS. Energy. Lett. 2024, 9, 93-101.
68. Liu, M.; Lee, J.; Yang, T. C.; et al. Synergies of Fe single atoms and clusters on N-doped carbon electrocatalyst for pH-universal oxygen reduction. Small. Methods. 2021, 5, e2001165.
69. Han, A.; Sun, W.; Wan, X.; et al. Construction of Co4 atomic clusters to enable Fe-N4 motifs with highly active and durable oxygen reduction performance. Angew. Chem. Int. Ed. 2023, 62, e202303185.
70. Li, Y.; Li, Z.; Shi, K.; et al. Single-atom Mn catalysts via integration with Mn sub nano-clusters synergistically enhance oxygen reduction reaction. Small 2024, 20, e2309727.
71. Yuan, L. J.; Liu, B.; Shen, L. X.; et al. d-orbital electron delocalization realized by axial Fe4C atomic clusters delivers high-performance Fe-N-C catalysts for oxygen reduction reaction. Adv. Mater. 2023, 35, e2305945.
72. Jiang, W. J.; Gu, L.; Li, L.; et al. Understanding the high activity of Fe-N-C electrocatalysts in oxygen reduction: Fe/Fe3C nanoparticles boost the activity of Fe-Nx. J. Am. Chem. Soc. 2016, 138, 3570-8.
73. Chen, G.; Liu, Y.; Xue, S.; et al. Exceptionally bifunctional ORR/OER performance via synergistic atom-cluster interaction. Small 2024, 20, e2308192.
74. Cui, X.; Gao, L.; Lei, S.; et al. Simultaneously crafting single-atomic Fe sites and graphitic layer-wrapped Fe3C nanoparticles encapsulated within mesoporous carbon tubes for oxygen reduction. Adv. Funct. Mater. 2021, 31, 2009197.
75. Wei, X.; Song, S.; Wu, N.; et al. Synergistically enhanced single-atomic site Fe by Fe3C@C for boosted oxygen reduction in neutral electrolyte. Nano. Energy. 2021, 84, 105840.
76. Lee, Y.; Ahn, J. H.; Jang, H.; et al. Very strong interaction between FeN4 and titanium carbide for durable 4-electron oxygen reduction reaction suppressing catalyst deactivation by peroxide. J. Mater. Chem. A. 2022, 10, 24041-50.
77. Pan, Y.; Li, M.; Mi, W.; et al. Single-atomic Mn sites coupled with Fe3C nanoparticles encapsulated in carbon matrixes derived from bimetallic Mn/Fe polyphthalocyanine conjugated polymer networks for accelerating electrocatalytic oxygen reduction. Nano. Res. 2022, 15, 7976-85.
78. Xu, C.; Guo, C.; Liu, J.; et al. Accelerating the oxygen adsorption kinetics to regulate the oxygen reduction catalysis via Fe3C nanoparticles coupled with single Fe-N4 sites. Energy. Storage. Mater. 2022, 51, 149-58.
79. Bae, G.; Han, S.; Oh, H. S.; Choi, C. H. Operando stability of single-atom electrocatalysts. Angew. Chem. Int. Ed. 2023, 62, e202219227.
80. Choi, C. H.; Lim, H. K.; Chung, M. W.; et al. The Achilles’ heel of iron-based catalysts during oxygen reduction in an acidic medium. Energy. Environ. Sci. 2018, 11, 3176-82.
81. Bae, G.; Chung, M. W.; Ji, S. G.; Jaouen, F.; Choi, C. H. pH effect on the H2O2-induced deactivation of Fe-N-C catalysts. ACS. Catal. 2020, 10, 8485-95.
82. Li, Y.; Chen, M. Y.; Lu, B. A.; Wu, H. R.; Zhang, J. N. Unravelling the role of hydrogen peroxide in pH-dependent ORR performance of Mn-N-C catalysts. Appl. Catal. B. Environ. 2024, 342, 123458.
83. Luo, E.; Zhang, H.; Wang, X.; et al. Single-atom Cr-N4 sites designed for durable oxygen reduction catalysis in acid media. Angew. Chem. Int. Ed. 2019, 58, 12469-75.
84. Guo, Y.; Wang, C.; Xiao, Y.; et al. Stabilizing Fe single atom catalysts by implanting Cr atomic clusters to boost oxygen reduction reaction. Appl. Catal. B. Environ. Energy. 2024, 344, 123679.
85. Lawler, R.; Cho, J.; Ham, H. C.; et al. CeO2 (111) surface with oxygen vacancy for radical scavenging: a density functional theory approach. J. Phys. Chem. C. 2020, 124, 20950-9.
86. Karakoti, A.; Singh, S.; Dowding, J. M.; Seal, S.; Self, W. T. Redox-active radical scavenging nanomaterials. Chem. Soc. Rev. 2010, 39, 4422-32.
87. Xie, H.; Xie, X.; Hu, G.; et al. Ta-TiOx nanoparticles as radical scavengers to improve the durability of Fe-N-C oxygen reduction catalysts. Nat. Energy. 2022, 7, 281-9.
88. Li, J.; Maurya, S.; Kim, Y. S.; et al. Stabilizing single-atom iron electrocatalysts for oxygen reduction via ceria confining and trapping. ACS. Catal. 2020, 10, 2452-8.
89. Cheng, X.; Jiang, X.; Yin, S.; et al. Instantaneous free radical scavenging by CeO2 nanoparticles adjacent to the Fe-N4 active sites for durable fuel cells. Angew. Chem. Int. Ed. 2023, 62, e202306166.
90. Gao, X. B.; Wang, Y.; Xu, W.; et al. Mechanism of particle-mediated inhibition of demetalation for single-atom catalytic sites in acidic electrochemical environments. J. Am. Chem. Soc. 2023, 145, 15528-37.
91. Bae, G.; Kim, M. M.; Han, M. H.; et al. Unravelling the complex causality behind Fe-N-C degradation in fuel cells. Nat. Catal. 2023, 6, 1140-50.
92. Mechler, A. K.; Sahraie, N. R.; Armel, V.; et al. Stabilization of iron-based fuel cell catalysts by non-catalytic platinum. J. Electrochem. Soc. 2018, 165, F1084-91.
93. Xiao, F.; Wang, Q.; Xu, G. L.; et al. Atomically dispersed Pt and Fe sites and Pt-Fe nanoparticles for durable proton exchange membrane fuel cells. Nat. Catal. 2022, 5, 503-12.
94. Zhou, H.; Yang, T.; Kou, Z.; et al. Negative pressure pyrolysis induced highly accessible single sites dispersed on 3D graphene frameworks for enhanced oxygen reduction. Angew. Chem. Int. Ed. 2020, 59, 20465-9.
95. Yu, Y.; Lv, Z.; Liu, Z.; et al. Activation of Ga liquid catalyst with continuously exposed active sites for electrocatalytic C-N coupling. Angew. Chem. Int. Ed. 2024, 63, e202402236.
96. Yuan, S.; Peng, J.; Zhang, Y.; et al. Tuning the catalytic activity of Fe-phthalocyanine-based catalysts for the oxygen reduction reaction by ligand functionalization. ACS. Catal. 2022, 12, 7278-87.
97. Fei, H.; Dong, J.; Chen, D.; et al. Single atom electrocatalysts supported on graphene or graphene-like carbons. Chem. Soc. Rev. 2019, 48, 5207-41.
98. Zhao, Z.; Xiong, Y.; Yu, S.; et al. Single-atom Zn with nitrogen defects on biomimetic 3D carbon nanotubes for bifunctional oxygen electrocatalysis. J. Colloid. Interface. Sci. 2023, 650, 934-42.
99. Li, L.; Liu, X.; Wang, J.; et al. Atomically dispersed Co in a cross-channel hierarchical carbon-based electrocatalyst for high-performance oxygen reduction in Zn-air batteries. J. Mater. Chem. A. 2022, 10, 18723-9.
100. Tian, H.; Song, A.; Zhang, P.; et al. High durability of Fe-N-C single atom catalysts with carbon vacancies towards oxygen reduction reaction in alkaline media. Adv. Mater. 2023, 35, 2210714.
101. Liu, K.; Fu, J.; Lin, Y.; et al. Insights into the activity of single-atom Fe-N-C catalysts for oxygen reduction reaction. Nat. Commun. 2022, 13, 2075.
102. Wang, X.; Jia, Y.; Mao, X.; et al. Edge-rich Fe-N4 active sites in defective carbon for oxygen reduction catalysis. Adv. Mater. 2020, 32, e2000966.
103. Fu, X.; Li, N.; Ren, B.; et al. Tailoring FeN4 sites with edge enrichment for boosted oxygen reduction performance in proton exchange membrane fuel cell. Adv. Energy. Mater. 2019, 9, 1803737.
104. Kong, F.; Huang, Y.; Chen, M.; et al. Creation of densely exposed and cavity-edged single Fe active sites for enhanced oxygen electroreduction. Appl. Catal. B. Environ. 2022, 317, 121768.
105. Zhang, R.; Xue, B.; Tao, Y.; et al. Edge-site engineering of defective Fe-N4 nanozymes with boosted catalase-like performance for retinal vasculopathies. Adv. Mater. 2022, 34, e2205324.
106. Cui, L.; Zhao, J.; Liu, G.; Wang, Z.; Li, B.; Zong, L. Rich edge-hosted single-atomic Cu-N4 sites for highly efficient oxygen reduction reaction performance. J. Colloid. Interface. Sci. 2022, 622, 209-17.
107. Mun, Y.; Lee, S.; Kim, K.; et al. Versatile strategy for tuning ORR activity of a single Fe-N4 site by controlling electron-withdrawing/donating properties of a carbon plane. J. Am. Chem. Soc. 2019, 141, 6254-62.
108. Gu, Y.; Xi, B. J.; Zhang, H.; Ma, Y. C.; Xiong, S. L. Activation of main-group antimony atomic sites for oxygen reduction catalysis. Angew. Chem. Int. Ed. 2022, 61, e202202200.
109. Chang, X.; Xu, S.; Wang, D.; Zhang, Z.; Guo, Y.; Kang, S. Flash dual-engineering of surface carboxyl defects and single Cu atoms of
110. Lv, M.; Cui, C. X.; Huang, N.; et al. Precisely engineering asymmetric atomic CoN4 by electron donating and extracting for oxygen reduction reaction. Angew. Chem. Int. Ed. 2024, 63, e202315802.
111. Wei, X.; Jiang, C.; Xu, H.; et al. Synergistic effect of organic ligands on metal site spin states in 2D metal-organic frameworks for enhanced ORR performance. ACS. Catal. 2023, 13, 15663-72.
112. Liu, M.; Sun, T.; Peng, T.; et al. Fe-NC single-atom catalyst with hierarchical porous structure and P-O bond coordination for oxygen reduction. ACS. Energy. Lett. 2023, 8, 4531-9.
113. Tang, C.; Chen, L.; Li, H.; et al. Tailoring acidic oxygen reduction selectivity on single-atom catalysts via modification of first and second coordination spheres. J. Am. Chem. Soc. 2021, 143, 7819-27.
114. Qiao, Y.; Yuan, P.; Hu, Y.; et al. Sulfuration of an Fe-N-C catalyst containing FexC/Fe species to enhance the catalysis of oxygen reduction in acidic media and for use in flexible Zn-air batteries. Adv. Mater. 2018, 30, e1804504.
115. Chi, B.; Zhang, L.; Yang, X.; et al. Promoting ZIF-8-derived Fe-N-C oxygen reduction catalysts via Zr doping in proton exchange membrane fuel cells: durability and activity enhancements. ACS. Catal. 2023, 13, 4221-30.
116. Zhu, P.; Xiong, X.; Wang, X.; et al. Regulating the FeN4 moiety by constructing Fe-Mo dual-metal atom sites for efficient electrochemical oxygen reduction. Nano. Lett. 2022, 22, 9507-15.
117. Zhang, M.; Li, H.; Chen, J.; et al. High-loading Co single atoms and clusters active sites toward enhanced electrocatalysis of oxygen reduction reaction for high-performance Zn-air battery. Adv. Funct. Mater. 2023, 33, 2209726.
118. Liu, H.; Jiang, L.; Khan, J.; et al. Decorating single-atomic Mn sites with FeMn clusters to boost oxygen reduction reaction. Angew. Chem. Int. Ed. 2023, 62, e202214988.
119. Wei, X.; Song, S.; Cai, W.; et al. Pt nanoparticle-Mn single-atom pairs for enhanced oxygen reduction. ACS. Nano. 2024, 18, 4308-19.
120. Xu, X.; Li, X.; Lu, W.; et al. Collective effect in a multicomponent ensemble combining single atoms and nanoparticles for efficient and durable oxygen reduction. Angew. Chem. Int. Ed. Engl. 2024, 63, e202400765.
121. Zhang, Y.; Chen, Z. W.; Liu, X.; et al. Vacancy-enhanced Sb-N4 sites for the oxygen reduction reaction and Zn-air battery. Nano. Lett. 2024, 24, 4291-9.
122. Lyu, L.; Hu, X.; Lee, S.; et al. Oxygen reduction kinetics of Fe-N-C single atom catalysts boosted by pyridinic N vacancy for temperature-adaptive Zn-air batteries. J. Am. Chem. Soc. 2024, 146, 4803-13.
123. Liu, H.; Jiang, L.; Sun, Y.; et al. Revisiting the role of sulfur functionality in regulating the electron distribution of single-atomic Fe sites toward enhanced oxygen reduction. Adv. Funct. Mater. 2023, 33, 2304074.
124. Qu, Y.; Li, Z.; Chen, W.; et al. Direct transformation of bulk copper into copper single sites via emitting and trapping of atoms. Nat. Catal. 2018, 1, 781-6.
Cite This Article
How to Cite
Zhang, D.; Zhang, X.; Li, X.; Feng, C.; Chu, Y.; Chen, C.; Kou, Z. Modulating single-atom M-N-C electrocatalysts for the oxygen reduction: the insights beyond the first coordination shell. Energy Mater. 2025, 5, 500009. http://dx.doi.org/10.20517/energymater.2024.42
Download Citation
Export Citation File:
Type of Import
Tips on Downloading Citation
Citation Manager File Format
Type of Import
Direct Import: When the Direct Import option is selected (the default state), a dialogue box will give you the option to Save or Open the downloaded citation data. Choosing Open will either launch your citation manager or give you a choice of applications with which to use the metadata. The Save option saves the file locally for later use.
Indirect Import: When the Indirect Import option is selected, the metadata is displayed and may be copied and pasted as needed.
About This Article
Special Issue
Copyright
Data & Comments
Data
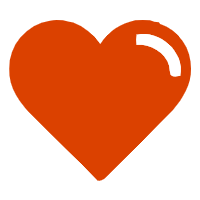
Comments
Comments must be written in English. Spam, offensive content, impersonation, and private information will not be permitted. If any comment is reported and identified as inappropriate content by OAE staff, the comment will be removed without notice. If you have any queries or need any help, please contact us at support@oaepublish.com.