Polybrominated diphenyl ether (PBDE) removal from wastewater: a perspective on advancing microbial technologies
Abstract
Contaminants of emerging concern (CEC) continue to plague wastewater treatment facilities in their battle to recover water resources and circumvent environmental contamination. Of particular challenge are legacy pollutants - pollutants that are no longer in use but continue to enter wastewater treatment facilities due to their historic uses. Among these pollutants are polybrominated diphenyl ethers (PBDEs), a flame retardant used in many consumer products. PBDEs have been detected in waterways worldwide and were documented to impact juvenile Chinook salmon in the waterways of Puget Sound in Washington State, USA. This has both economic impacts in the region where salmon is a major commercial export, and societal implications where native tribes have deep cultural heritage connections with salmon. PBDEs are primarily removed from wastewater by sorption to wastewater sludge. However, this process can result in long-term impacts when PBDEs re-emerge in landfill leachates after sorptive material disposal. In this perspective, we explore what is currently understood about engineered solutions for the removal of PBDEs from wastewater, and we present an approach for the permanent destruction of PBDEs, which combines anaerobic bioprocesses for initial debromination, followed by aerobic treatments for the final degradation of the PBDEs. Through this perspective, we hope to inspire global research efforts to develop sustainable biological treatment solutions for CEC destruction during wastewater treatment.
Keywords
INTRODUCTION
Flame retardants, such as polybrominated diphenyl ethers (PBDEs), are a contaminants of emerging concern (CEC) that pose many environmental challenges. Used for decades in a variety of consumer products such as furniture and electronics, PBDEs are detected in the environment despite production bans and regulatory efforts[1]. They are found in aquatic environments at concentrations that can adversely affect the health of exposed species[2,3]. Specifically, PBDEs disrupt endocrine systems in fish and other animals, leading to health implications[4], including impaired thyroid function[5] and changes in disease susceptibility[2,3].
The chemical and physical characteristics have previously been described[4]. Briefly, PBDEs have the base chemical formula of C12H(x)Br(10-x)O. There are 209 possible congeners with log KOW ranging from 4.3 to 9.9, all of which are classified as hydrophobic. Given the challenge presented by their large number, sorption coefficients for the PBDE congeners have been estimated using modeling methods[6], while physical measurements of these coefficients have been less frequent. Sorption potential generally increases with increased bromination, with the exception of some congeners (such as BDE-154, BDE-183, and BDE-209) where bromide-bromide interactions can interfere with sorption behavior[6].
A common pathway for PBDE movement to the environment is through treated wastewater[7], highlighting the need for effective treatment technologies. While considerable research has focused on various methods for PBDE removal from wastewater, these are limited in their effectiveness[8]. There remains a critical gap in exploring the potential of integrating biological degradation with sorptive techniques to enhance pollutant removal. In this perspective, an approach is proposed using bio-inoculated sorptive materials to support and improve the bacterial degradation of PBDEs from wastewater, ultimately reducing the entry of PBDEs and other co-occurring CECs[9] into aquatic environments.
ENVIRONMENTAL IMPACTS OF PBDES
The global production of all PBDEs between 1970 and 2020 was around 1,900,000 tons[1,10]. Considering the low rate of degradation, a significant portion of this PBDE mass remains in products or distributed in the environment. PBDEs have been detected in soil, water, sediments[4], and wastewater[10] throughout the world. Concentrations of PBDEs in effluent wastewater are much lower than in influent, with more than half of the PBDEs associated with suspended particles in both raw and treated wastewater[10]. Pollution plumes in natural waters are very low and often measured in fmol per L concentrations[11]. However, PBDEs are lipophilic and persistent[12], which contributes to their impacts on marine and terrestrial species. As an alternative to measuring marine water plumes, pollution levels can be tracked by measuring concentration in the fatty tissues of fish, sharks, and whales[13,14]. PBDEs are present across the food web. Fish and other marine animals bioaccumulate and biomagnify PBDEs from effluent wastewater through exposures in water, sediment, and their food. PBDEs were detected in penguin eggs as far as Antarctica[15] and have been shown to accumulate across trophic levels of fish species[16,17], particularly in high trophic level marine mammals, including Southern Resident Killer Whales, which can accumulate high concentrations of persistent chemicals, such as PBDEs[18].
Despite decreasing trends in environmental concentrations, PBDEs continue to be a contaminant of concern. For example, West et al. (2017) reported that concentrations of PBDEs in Pacific herring declined between approximately 2000-2015, though levels remained above health effects thresholds, particularly in stocks from the more urbanized embayments[17]. Similar spatial patterns have been documented for PBDE concentrations in tissues of Dungeness crab, English sole, and spiny dogfish, with significantly higher concentrations being reported in biota from highly urbanized areas compared to less urbanized areas[19]. Receiving waters impacted by relatively high concentrations of wastewater effluent, even those outside urbanized areas, can be highly impacted by PBDEs[20]. Monitoring juvenile Chinook salmon in the Snohomish River found high concentrations of PBDEs, at levels that could affect fish health[2,3,7,21]. The highest concentrations were found in the fish at the lower portions of the Snohomish River, with values reaching 1,500 ng total PBDE per g lipid weight[21]. Treated wastewater discharges were identified as the source of PBDE in the Snohomish River. A screening study was performed to characterize the influent of a wastewater treatment plant (WWTP) in the Snohomish River basin, as well as the effluent of several industrial discharges that were released directly into the WWTP conveyance system[22]. The study identified three facilities (an industrial laundry, an aerospace facility, and a landfill) with PBDE concentrations
PAST APPROACHES TO PBDE TREATMENT
There has been a large body of work evaluating the treatment, fate, and transport of PBDEs (reviewed in[8,23]). Three treatment approaches have shown effectiveness in lab-based studies for removing PBDE from water, including sorptive removal, biological transformation, and photocatalytic transformation [Table 1]. Each approach has limitations, and there is currently a lack of technologies that have been adopted within the wastewater treatment industry.
Methods of PBDEs removal from wastewater
Material or organism | Matrix | Congener | Removal (%) | Final concentration | Ref. |
Existing full-scale treatment plants | |||||
Secondary treatment (8 facilities) | Activated sludge (biological and sorption) | BDE-47 BDE-99 BDE-209 | 40%-99% 39%-98% 35%-99% | 0.8-11 ng/L 0.3-9.7 ng/L 1.3-36 ng/L | [25] |
Secondary treatment | Sequencing batch activated sludge (biological and sorption) | Total PBDE | 86% | 1 ng/L | [24] |
Sorption | |||||
Powdered tea and coconut leaves | Deionized water spiked with BDE | BDE-3 BDE-15 | > 90% | < 0.4 mg/L | [26] |
Activated carbon | Secondary effluent | BDE-47 BDE-99 BDE-209 | 30% 75% 90% | 1.7 ng/L 2.4 ng/L 405 ng/L | [28] |
Biological degradation | |||||
Aerobic mixed culture of Brevibacillus sp. and Achromobacter sp. | LB medium | BDE-209 | 88% | 1.2 mg/L | [29] |
Rhodococcus ruber TAW-CT127 | Manual marine culture medium | BDE-209 | 81% | 9.5 mg/L | [23] |
Microbacterium strain Y2 | LB medium Mineral salts medium | BDE-209 | [36] | ||
Dehalococcoides ethenogenes 195 | Mineral salts medium | Octa-BDE mix | 91% | 11.5 nmol | [32] |
Dehalococcoides mccartyi TZ50 | Mineral salts medium Concentrated mixed liquor from the anoxic zone in a domestic WWTP | BDE-47 | 31% | 0.35 μM | [34] |
Photocatalysis | |||||
Visible light withAgI–TiO2 | Water | BDE-209 | 70% | 2.9 mg/L | [61] |
Xenon lamp with Ag@Ag3PO4/g-C3N4/rGO | Methanol | BDE-47 | 93% | 0.32 mg/L | [62] |
rGO/TiO2 | water | BDE-47 | 25% | 3.75 mg/L | [63] |
Sorption is the primary removal mechanism in traditional wastewater treatment[24]. PBDEs are hydrophobic compounds and thus have an affinity to sorb to organic particulate matter. During wastewater treatment, PBDEs are moved from the water to the activated sludge[25]. Less-brominated BDEs (BDE-3 and BDE-15) partition to common adsorbents (black tea and coconut palm leaf powders) with short retention times[26]. Engineered modifications to secondary treatment processes can alter sorption efficiency, including chemical modifiers to improve adsorption or cation additions to decrease adsorption[27], though this approach has not been tested for PBDEs. Sorptive removal of PBDEs has additionally been demonstrated as a tertiary (i.e., polishing) step for municipal wastewater treatment[28], documenting the efficacy of absorbents across the range of PBDE congeners under environmentally realistic conditions. While modeling[6] provides a starting point for sorptive-removal designs, the experimental data on sorption coefficients for all 209 congeners with comparisons among differing sorptive materials and wastewater conditions are missing. Filling this gap will require worldwide data sharing building on current efforts[24,26,28] to ensure that information is garnered from case studies and bench studies representing broad ranges of wastewater conditions. As an additional challenge, sorption only moves the PBDEs, and the sorbent must be either regenerated or, more often, disposed of in a landfill. Since landfill leachate is among the industrial sources of PBDEs to municipal wastewater treatment facilities, sorption alone is not a sustainable solution.
Complete bacterial degradation of PBDEs can occur, but the process does not occur in a single step. Aerobic biodegradation of PBDEs has been documented using bacteria within the Bacillus[24] and Rhodococcus sp. (with genome evaluation)[23], and by aerobic mixed cultures enriched in Arcobacter sp.[29]. However, aerobic PBDE biodegradation can be congener-specific[30], and highly brominated PBDEs are resistant to oxidation. During aerobic wastewater treatment, the removal of highly brominated PBDE (i.e., BDE-209) is via absorption and not by microbial degradation[31]. Anaerobic bacteria are capable of debrominating PBDEs[32]. Several cultured anaerobic bacteria have been identified with intrinsic PBDE-debromination capabilities, such as Dehalococcoides sp.[33,34]. Other work has found PBDE debrominators present in wastewater sludge[35,36]. Shifts in microbial community structures have been documented in association with improved BDE-209 degradation during anaerobic wastewater treatment[37], and facultative anaerobes can also contribute to BDE-209 degradation, though these are often identified to be uncultured species[36]. However, the anaerobic step on its own is insufficient, because highly brominated compounds - such as BDE-209 - are converted to partially brominated compounds - such as BDE-90 and -47, which are not completely degraded and may be more toxic than the parent compound. To achieve complete destruction of the PBDEs, an oxidative step is required, during which aerobic bacteria break the carbon-carbon bonds in the diphenyl ether core of less-brominated PBDE, resulting in final destruction[38]. Genetic studies have been conducted with an aim toward identifying the genes associated with aerobic[38] and anaerobic[39] PBDE biodegradation, which allow current researchers to test hypotheses about whether PBDE-degrading genes are active in a treatment system.
ENHANCING MICROBIAL TREATMENT TECHNOLOGIES THROUGH COMBINATION WITH SORPTION
Neither sorptive removal nor biological degradation, when used alone, is an effective approach for PBDE removal in wastewater treatment systems[8]. Spent sorptive materials must be disposed of or recharged, and bacterial degradation can be too slow compared to the treatment times in wastewater systems. A solution for increasing the residence time for the bacteria in wastewater could be achieved by combining sorption with biological degradation. This combined approach has been documented in anaerobic granular sludge reactors for some trace-level organic pollutants where sorption to the biological granules was shown to be part of the removal mechanism[40], though this combination has yet to be tested for the treatment of PBDEs. As an additional example, a biofilm fluidized-bed reactor can improve biodegradation by retaining slow-growing bacteria within the reactor without the need for decreasing hydraulic retention times. Combined sorption and biological degradation has been demonstrated for recalcitrant organic pollutants such as phenol[41] and in sequencing batch mode for biodegradation of the recalcitrant aromatic pollutants 2,4-dinitrotoluene and 2,6-dinitrotoluene[42]. As an additional benefit, fluidized-bed reactors also function well for conventional wastewater contaminants while requiring less energy than other biosystems[43].
For a fluidized bed, the appropriate solid material support is important. Granular activated carbon (GAC) and zero-valent iron are both commonly used in wastewater treatment, but have an energy and material sourcing footprint that reduces the sustainability of these materials. Biochar is an eco-friendly granular support material that is documented for its ability to create an environment for microbes that contains cation exchange capacity, sorption capacity, and high porosity[44-46]. In addition, biochar can be made from a range of organic waste materials such as wood chips, algae, biosolids, crop residues, and other organic solid waste[47-50], making it a sustainable and locally sourceable resource for treatment processes. It has been reported as an excellent material for biofilm development[51] and is amenable to bioinoculation for application in wastewater treatment[52]. In addition, microbial interactions within the micropores in biochar facilitate stabilized anaerobic microbial processes[53], which is important for the breakdown of PBDE molecules into less brominated compounds.
We submit that two fluidized-bed reactors in series could solve the challenges of removal of PBDEs (and potentially other CECs) from wastewater. Using anaerobic-aerobic systems with biochar to treat municipal and industrial wastewater has been shown to achieve microbial degradation of other contaminants[54,55], and here we propose this as a potential solution for PBDE biodegradation. The first reactor would be anaerobic to allow PBDEs to debrominate. The anaerobic step requires an abundance of an electron donor[56], which would be supplied by the untreated municipal wastewater. The second reactor would be aerobic to allow biological cleavage of the carbon-carbon bonds in the diphenyl ether core of the residual PBDEs. Inoculated biochar added to each reactor would improve the treatment by encouraging rapid sorptive removal that would extend contact times for biological conversion. Pilot scale testing is needed to document the efficacy of immobilized bacterial cultures on sorptive materials using real-world wastewater to ensure an understanding of how the complex and variable matrix affects PBDE biological removal.
CONCLUSION
The scientific community has faced the challenge of treatment for trace-level organic pollutants for more than two decades[57-60]. It is time to move from documentation of the fundamentals toward demonstration of technologies that can be applied at real-world wastewater treatment facilities, while also considering the resource limitations faced by smaller communities. Inoculated biochar in anaerobic and aerobic reactors is a way forward through the innovative application of established knowledge that can create economically, socially, and environmentally sustainable solutions. Case study documentation of these efforts will be critical in establishing the influence of wastewater and biochar variability on the efficacy of this treatment approach.
DECLARATIONS
Authors’ contributions
Conceptualization: Gough, H. L.; James, C. A.
Writing - original drafting and literature review: Santos, P. A.; Gough, H. L.
Writing - review and editing: James, C. A.; Gough, H. L.
Availability of data and materials
Not applicable.
Financial support and sponsorship
This material is based upon work partially supported by the National Science Foundation Graduate Research Fellowship Program under Grant No. DGE-2140004, awarded to PAS. Any opinions, findings, and conclusions or recommendations expressed in this material are those of the author(s) and do not necessarily reflect the views of the National Science Foundation. This work was not supported by a funded research grant.
Conflicts of interest
All authors declared that there are no conflicts of interest.
Ethical approval and consent to participate
Not applicable.
Consent for publication
Not applicable.
Copyright
© The Author(s) 2025.
REFERENCES
1. Abbasi, G.; Buser, A. M.; Soehl, A.; Murray, M. W.; Diamond, M. L. Stocks and flows of PBDEs in products from use to waste in the U.S. and Canada from 1970 to 2020. Environ. Sci. Technol. 2015, 49, 1521-8.
2. Arkoosh, M. R.; Boylen, D.; Dietrich, J.; et al. Disease susceptibility of salmon exposed to polybrominated diphenyl ethers (PBDEs). Aquat. Toxicol. 2010, 98, 51-9.
3. Arkoosh, M. R.; Van Gaest, A. L.; Strickland, S. A.; et al. Dietary exposure to a binary mixture of polybrominated diphenyl ethers alters innate immunity and disease susceptibility in juvenile Chinook salmon (Oncorhynchus tshawytscha). Ecotoxicol. Environ. Saf. 2018, 163, 96-103.
4. Akortia, E.; Okonkwo, J. O.; Lupankwa, M.; et al. A review of sources, levels, and toxicity of polybrominated diphenyl ethers (PBDEs) and their transformation and transport in various environmental compartments. Environ. Rev. 2016, 24, 253-73.
5. Arkoosh, M. R.; Van Gaest, A. L.; Strickland, S. A.; Hutchinson, G. P.; Krupkin, A. B.; Dietrich, J. P. Alteration of thyroid hormone concentrations in juvenile Chinook salmon (Oncorhynchus tshawytscha) exposed to polybrominated diphenyl ethers, BDE-47 and BDE-99. Chemosphere 2017, 171, 1-8.
6. Kim, T.; Kwon, Y.; Kwon, S. Bromination effect of polybrominated diphenyl ethers on the graphyne surface on enhanced adsorption characteristics using density functional theory study. AIP. Adv. 2020, 10, 075117.
7. Carey, A. J.; Wese, J. E.; Fisk, R. J.; Langness, M.; Ylitalo, G.; O’Neill, S. Location and source of PBDE exposure in juvenile Chinook salmon along their out-migrant pathway through the Snohomish River, WA. 2019. https://wdfw.wa.gov/publications/02241. (accessed on 2025-03-17).
8. Kim, M.; Han, J. Treatment techniques for removal of polybrominated diphenyl ethers (PBDEs) from real wastewater: Limitations, challenges, and future research directions. J. Water. Process. Eng. 2024, 63, 105463.
9. James, C. A.; Sofield, R.; Faber, M.; et al. The screening and prioritization of contaminants of emerging concern in the marine environment based on multiple biological response measures. Sci. Total. Environ. 2023, 886, 163712.
10. Xu, G.; Zhao, X.; Zhao, S.; et al. Insights into the occurrence, fate, and impacts of halogenated flame retardants in municipal wastewater treatment plants. Environ. Sci. Technol. 2021, 55, 4205-26.
11. Sun, Y.; Francois, R.; Pawlowicz, R.; Maldonado, M. T.; Stevens, S. W.; Soon, M. Distribution, sources and dispersion of polybrominated diphenyl ethers in the water column of the Strait of Georgia, British Columbia, Canada. Sci. Total. Environ. 2023, 873, 162174.
12. Zhang, Y.; Wang, W.; Song, J.; et al. Environmental characteristics of polybrominated diphenyl ethers in marine system, with emphasis on marine organisms and sediments. Biomed. Res. Int. 2016, 2016, 1317232.
13. Lee, H. K.; Kim, S. J.; Jeong, Y.; et al. Polybrominated diphenyl ethers in thirteen shark species from offshore and coastal waters of Korea. Mar. Pollut. Bull. 2015, 95, 374-9.
14. Mongillo, T. M.; Holmes, E. E.; Noren, D. P.; et al. Predicted polybrominated diphenyl ether (PBDE) and polychlorinated biphenyl (PCB) accumulation in southern resident killer whales. Mar. Ecol. Prog. Ser. 2012, 453, 263-77.
15. Morales, P.; Roscales, J. L.; Muñoz-Arnanz, J.; Barbosa, A.; Jiménez, B. Evaluation of PCDD/Fs, PCBs and PBDEs in two penguin species from Antarctica. Chemosphere 2022, 286, 131871.
16. Kim, U. J.; Jo, H.; Lee, I. S.; Joo, G. J.; Oh, J. E. Investigation of bioaccumulation and biotransformation of polybrominated diphenyl ethers, hydroxylated and methoxylated derivatives in varying trophic level freshwater fishes. Chemosphere 2015, 137, 108-14.
17. West, J. E.; O’Neill, S. M.; Ylitalo, G. M. Time trends of persistent organic pollutants in benthic and pelagic indicator fishes from Puget Sound, Washington, USA. Arch. Environ. Contam. Toxicol. 2017, 73, 207-29.
18. Rayne, S.; Ikonomou, M. G.; Ross, P. S.; Ellis, G. M.; Barrett-Lennard, L. G. PBDEs, PBBs, and PCNs in three communities of free-ranging killer whales (Orcinus orca) from the northeastern Pacific Ocean. Environ. Sci. Technol. 2004, 38, 4293-9.
19. Ikonomou, M. G.; Fernandez, M. P.; Hickman, Z. L. Spatio-temporal and species-specific variation in PBDE levels/patterns in British Columbia’s coastal waters. Environ. Pollut. 2006, 140, 355-63.
20. Td, MacRae JD. Polybrominated diphenyl ethers in fish and wastewater samples from an area of the Penobscot River in central Maine. Chemosphere 2006, 62, 1153-60.
21. O’Neill, S. M.; Carey, A. J.; Harding, L. B.; West, J. E.; Ylitalo, G. M.; Chamberlin, J. W. Chemical tracers guide identification of the location and source of persistent organic pollutants in juvenile Chinook salmon (Oncorhynchus tshawytscha), migrating seaward through an estuary with multiple contaminant inputs. Sci. Total. Environ. 2020, 712, 135516.
22. Wong, S. Chemicals of emerging concern in pretreated industrial wastewater in northwestern Washington State: screening study results, 2021. https://apps.ecology.wa.gov/publications/documents/2203013.pdf. (accessed on 2025-03-17).
23. Xu, H.; Cai, Q.; An, Q.; et al. Aerobic degradation characteristics of decabromodiphenyl ether through rhodococcus ruber TAW-CT127 and its preliminary genome analysis. Microorganisms 2022, 10, 1441.
24. Paliya, S.; Mandpe, A.; Bhisikar, D.; Kumar, M. S.; Kumar, S. Polybrominated diphenyl ethers (PBDEs) in Indian wastewater treatment plant: occurrence, mass flow and removal. Chemosphere 2022, 303, 135055.
25. Shen, J.; Smyth, S. A.; Droste, R.; Delâge, D. Variability of release rate of flame retardants in wastewater treatment plants. Environ. Sci. Pollut. Res. Int. 2018, 25, 34740-52.
26. Chang, R.; Jien, S.; Weng, C.; Lee, T.; Liao, C. Fast Removal of polybrominated diphenyl ethers from aqueous solutions by using low-cost adsorbents. Sustainability 2017, 9, 102.
27. Duan, F. A.; Wang, J.; Ismail, S.; Sung, S.; Cui, Z.; Ni, S. Q. Hydroxypropyl-β-cyclodextrin improves the removal of polycyclic aromatic hydrocarbons by aerobic granular sludge. Environ. Technol. 2022, 43, 3262-8.
28. Mohamed, B. A.; Hamid, H.; Montoya-Bautista, C. V.; Li, L. Y. Circular economy in wastewater treatment plants: treatment of contaminants of emerging concerns (CECs) in effluent using sludge-based activated carbon. J. Clean. Prod. 2023, 389, 136095.
29. Hu, D.; Wu, J.; Fan, L.; Li, S.; Jia, R. Aerobic degradation characteristics and mechanism of decabromodiphenyl ether (BDE-209) using complex bacteria communities. Int. J. Environ. Res. Public. Health. 2022, 19, 17012.
30. Gu, C.; Wang, L.; Jin, Z.; et al. Congener-specificity, dioxygenation dependency and association with enzyme binding for biodegradation of polybrominated diphenyl ethers by typical aerobic bacteria: Experimental and theoretical studies. Chemosphere 2023, 314, 137697.
31. Ni, S. Q.; Cui, Q.; Zheng, Z. Interaction of polybrominated diphenyl ethers and aerobic granular sludge: biosorption and microbial degradation. Biomed. Res. Int. 2014, 2014, 274620.
32. He, J.; Robrock, K. R.; Alvarez-Cohen, L. Microbial reductive debromination of polybrominated diphenyl ethers (PBDEs). Environ. Sci. Technol. 2006, 40, 4429-34.
33. Zhao, S.; Fan, S.; He, Y.; Zhang, Y. Microbial debromination of polybrominated diphenyl ethers by dehalococcoides-containing enrichment culture. Front. Microbiol. 2021, 12, 806795.
34. Zhao, S.; Rogers, M. J.; Cao, L.; Ding, C.; He, J. Identification of reductive dehalogenases that mediate complete debromination of penta- and tetrabrominated diphenyl ethers in Dehalococcoides spp. Appl. Environ. Microbiol. 2021, 87, e0060221.
35. Xu, G.; Zhao, S.; He, J. Underexplored organohalide-respiring bacteria in sewage sludge debrominating polybrominated diphenyl ethers. Environ. Sci. Technol.2024.
36. Yu, Y.; Yin, H.; Huang, W.; Peng, H.; Lu, G.; Dang, Z. Cellular changes of microbial consortium GY1 during decabromodiphenyl ether (BDE-209) biodegradation and identification of strains responsible for BDE-209 degradation in GY1. Chemosphere 2020, 249, 126205.
37. Zhou, Q.; Ge, C.; Ahmad, H. A.; et al. Production of more toxic hexa-brominated diphenyl ether from rapid biotransformation of decabromodiphenyl ether in anaerobic granular sludge. Int. Biodeterior. Biodegrad. 2018, 134, 7-15.
38. Wang, S.; Bai, N.; Wang, B.; et al. Characterization of the molecular degradation mechanism of diphenyl ethers by Cupriavidus sp. WS. Environ. Sci. Pollut. Res. Int. 2015, 22, 16914-26.
39. Robrock, K. R.; Korytár, P.; Alvarez-Cohen, L. Pathways for the anaerobic microbial debromination of polybrominated diphenyl ethers. Environ. Sci. Technol. 2008, 42, 2845-52.
40. Kent, J.; Tay, J. H. Treatment of 17α-ethinylestradiol, 4-nonylphenol, and carbamazepine in wastewater using an aerobic granular sludge sequencing batch reactor. Sci. Total. Environ. 2019, 652, 1270-8.
41. Kryst, K.; Karamanev, D. G. Aerobic phenol biodegradation in an inverse fluidized-bed biofilm reactor. Ind. Eng. Chem. Res. 2001, 40, 5436-9.
42. Lendenmann, U.; Spain, J. C.; Smets, B. F. Simultaneous biodegradation of 2,4-dinitrotoluene and 2,6-dinitrotoluene in an aerobic fluidized-bed biofilm reactor. Environ. Sci. Technol. 1998, 32, 82-7.
43. Swain, A. K.; Sahoo, A.; Jena, H. M.; Patra, H. Industrial wastewater treatment by Aerobic Inverse Fluidized Bed Biofilm Reactors (AIFBBRs): a review. J. Water. Process. Eng. 2018, 23, 61-74.
44. Cui, L.; Yan, J.; Yang, Y.; et al. Influence of biochar on microbial activities of heavy metals contaminated paddy fields. BioRes 2013, 8, 5536-48.
45. Chen, Y.; Liu, Y.; Li, Y.; et al. Influence of biochar on heavy metals and microbial community during composting of river sediment with agricultural wastes. Bioresour. Technol. 2017, 243, 347-55.
46. Pommier, T.; Merroune, A.; Bettarel, Y.; et al. Off-site impacts of agricultural composting: role of terrestrially derived organic matter in structuring aquatic microbial communities and their metabolic potential. FEMS. Microbiol. Ecol. 2014, 90, 622-32.
47. Kambo, H. S.; Dutta, A. A comparative review of biochar and hydrochar in terms of production, physico-chemical properties and applications. Renew. Sustain. Energy. Rev. 2015, 45, 359-78.
48. Xiang, W.; Zhang, X.; Chen, J.; et al. Biochar technology in wastewater treatment: a critical review. Chemosphere 2020, 252, 126539.
49. Colantoni, A.; Evic, N.; Lord, R.; et al. Characterization of biochars produced from pyrolysis of pelletized agricultural residues. Renew. Sustain. Energy. Rev. 2016, 64, 187-94.
50. Xiong, X.; Yu, I. K.; Tsang, D. C.; et al. Value-added chemicals from food supply chain wastes: state-of-the-art review and future prospects. Chem. Eng. J. 2019, 375, 121983.
51. Ming, J.; Wang, Q.; Yoza, B. A.; et al. Bioreactor performance using biochar and its effect on aerobic granulation. Bioresour. Technol. 2020, 300, 122620.
52. El Shahawy, A.; Mohamed, A.; El-Shatoury, S.; et al. Using phragmites australis biochar bio-augmented with actinomycetes for enhancing UASB reactor performance: a field study. J. Water. Process. Eng. 2024, 68, 106461.
53. Chakraborty, I.; Sathe, S. M.; Dubey, B. K.; Ghangrekar, M. M. Waste-derived biochar: applications and future perspective in microbial fuel cells. Bioresour. Technol. 2020, 312, 123587.
54. Lin, S.; Rong, K.; Lamichhane, K. M.; Babcock, R. W.; Kirs, M.; Cooney, M. J. Anaerobic-aerobic biofilm-based digestion of chemical contaminants of emerging concern (CEC) and pathogen indicator organisms in synthetic wastewater. Bioresour. Technol. 2020, 299, 122554.
55. Chan, Y. J.; Chong, M. F.; Law, C. L.; Hassell, D. A review on anaerobic–aerobic treatment of industrial and municipal wastewater. Chem. Eng. J. 2009, 155, 1-18.
56. Qiu, M.; Chen, X.; Deng, D.; et al. Effects of electron donors on anaerobic microbial debromination of polybrominated diphenyl ethers (PBDEs). Biodegradation 2012, 23, 351-61.
57. Lust, M. J.; Ziels, R. M.; Strand, S. E.; Gough, H. L.; Stensel, H. D. Biodegradation kinetics of 17α-ethinylestradiol in activated sludge treatment processes. Environ. Eng. Sci. 2015, 32, 637-46.
58. Zhou, N. A.; Lutovsky, A. C.; Andaker, G. L.; Ferguson, J. F.; Gough, H. L. Kinetics modeling predicts bioaugmentation with Sphingomonad cultures as a viable technology for enhanced pharmaceutical and personal care products removal during wastewater treatment. Bioresour. Technol. 2014, 166, 158-67.
59. Zhou, N. A.; Lutovsky, A. C.; Andaker, G. L.; Gough, H. L.; Ferguson, J. F. Cultivation and characterization of bacterial isolates capable of degrading pharmaceutical and personal care products for improved removal in activated sludge wastewater treatment. Biodegradation 2013, 24, 813-27.
60. Ziels, R. M.; Lust, M. J.; Gough, H. L.; Strand, S. E.; Stensel, H. D. Influence of bioselector processes on 17α-ethinylestradiol biodegradation in activated sludge wastewater treatment systems. Environ. Sci. Technol. 2014, 48, 6160-7.
61. Shao, Y.; Ye, W.; Sun, C.; Liu, C.; Wang, Q. Visible-light-induced degradation of polybrominated diphenyl ethers with AgI–TiO2. RSC. Adv. 2017, 7, 39089-95.
62. Wang, R.; Tang, T.; Wei, Y.; et al. Photocatalytic debromination of polybrominated diphenyl ethers (PBDEs) on metal doped TiO2 nanocomposites: mechanisms and pathways. Environ. Int. 2019, 127, 5-12.
Cite This Article
How to Cite
Download Citation
Export Citation File:
Type of Import
Tips on Downloading Citation
Citation Manager File Format
Type of Import
Direct Import: When the Direct Import option is selected (the default state), a dialogue box will give you the option to Save or Open the downloaded citation data. Choosing Open will either launch your citation manager or give you a choice of applications with which to use the metadata. The Save option saves the file locally for later use.
Indirect Import: When the Indirect Import option is selected, the metadata is displayed and may be copied and pasted as needed.
About This Article
Special Issue
Copyright
Data & Comments
Data
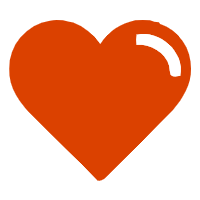
Comments
Comments must be written in English. Spam, offensive content, impersonation, and private information will not be permitted. If any comment is reported and identified as inappropriate content by OAE staff, the comment will be removed without notice. If you have any queries or need any help, please contact us at [email protected].