Ibuprofen and diclofenac in the marine environment - a critical review of their occurrence and potential risk for invertebrate species
Abstract
The presence of the two main non-steroidal anti-inflammatory drugs (NSAIDs), diclofenac (DCF) and ibuprofen (IBU), in marine and estuarine systems has been reported. Although the available information about the toxicity of these compounds in aquatic organisms has increased in recent years, the database about marine organisms, specifically invertebrates, is limited. Consequently, the assessment of potential risks posed by these compounds to aquatic species should be improved, given their relevance for aquatic life and the trophic chain. To fill this gap, we analyze the potential risk of IBU and DCF in marine invertebrates. To assess the risk, the database was built with available information from the scientific literature about the occurrence, bioaccumulation, and toxicity of both compounds in the estuaries and marine environments. Risk assessment of both compounds in these environments is scarce and based essentially on their acute toxicity. Data compiled in this review, including environmental concentrations and toxicity thresholds, were used to calculate risk quotients and classify the risk for invertebrate communities in coastal areas with different contamination levels. The results indicated a higher risk for DCF than IBU. Additionally, the simultaneous presence of the two NSAIDs in the aquatic environment increases the risk for exposed organisms. The use of classical methods (e.g., biochemical markers, gene expression), new approaches (e.g., adverse outcome pathway, AOP), and omic techniques has contributed to understanding the underlying toxicity mechanisms and improving the risk assessment. However, in a global change scenario involving multiple drivers and pressures such as ocean acidification, heat waves, climate change, etc., the risk assessment for these emerging pollutants needs improvement. This can be achieved by increasing our knowledge about the metabolic pathway and biotransformation routes of these compounds in marine species and understanding how these changes can affect the bioaccumulation, toxicity, fate, and behavior of both NSAIDs.
Keywords
INTRODUCTION
Marine pollution is a global environmental problem that requires joint action at the international level to minimize the impact on marine ecosystems and biodiversity. It arises as a consequence of anthropogenic activities on both land and sea. The chemicals released into the sea correspond to regulated and unregulated products. Many of them are known as emerging pollutants. This term includes many chemical compounds such as fire retardants, musks, industrial solvents, pesticides, personal care products, nanomaterials, microplastics, and pharmaceuticals, among others.
The most relevant legislations for the protection and regulation of the aquatic environment at the European level concerning the presence of contaminants are the Water Framework Directive (WFD, EC 2000) and the Marine Strategy Framework Directive (MSFD, 2008/56/EC and amendment EU 2017/845). The first one establishes a list of substances (the priority list) to assess the chemical status of water bodies (up to 12 nautical miles from the coastal line). In contrast, the MSFD is based on Descriptor 8, which states, “Concentrations of contaminants are at levels not giving rise to pollution effects.” Other initiatives to control pollution, including seafood, are related to regulatory agencies (ECHA, EFSA) and regional or international programs or conventions. Tornero and Hanke[1] summarized the potential chemical contaminants in the marine environment to monitor and assess the chemical pollution in European marine waters. Many substances are considered emerging pollutants and are not included in the routine monitoring program yet. Among the emerging pollutants, pharmaceuticals are a relevant group.
Pharmaceutical drug consumption from human or veterinary use can reach several thousands of tons in some countries[2-4]. Their presence has been reported in more than 70 countries representing all continents, and more than 600 substances have been detected in the aquatic environment[5]. Pharmaceutical compounds can be released into aquatic systems from different sources. After human or veterinary administration, drugs are metabolized and excreted as unaltered parental forms or metabolites (or metabolite mixtures). For this reason, wastewater treatment plants (WWTPs), hospital discharges, aquaculture facilities, and animal farming are considered the major pathways of drug entry into the aquatic environment[6-11]. Conventional WWTPs are typically designed for solids and organic loadings removal by primary and secondary treatments. However, these processes only partially remove the majority of pharmaceuticals from influents, and effluents discharge a complex mixture of unchanged compounds and metabolites/transformation products into the aquatic compartments, which can exhibit higher toxicity than the parental compounds[12,13]. Additional sources of drugs are industrial (pharmaceutical manufacturing) discharges, incorrect disposal of expired or unused medicines, and runoff of soils where sewage sludge or animal manures are applied as fertilizers[10]. The continuous input of these substances into the aquatic environment, coupled with their design to be biologically active also at low doses, can provoke negative effects for aquatic fauna, ecosystems, and in the end, even for human health.
Among pharmaceuticals, non-steroidal anti-inflammatory drugs (NSAIDs) are one of the main groups detected in aquatic ecosystems. This fact is related to their extensive prescription and over-the-counter commercialization worldwide, as well as their specific properties, which influence the inflow of these compounds to WWTPs and/or aquatic systems, their removal efficiency in WWTPs, mobility through WWTPs and natural waters, degradation rates, and persistence into the aquatic media[14,15]. They are employed to reduce pain or inpatient recovery from a medical condition or ailment. Two of the more prescribed NSAIDs are diclofenac (DCF) and ibuprofen (IBU) (chemical properties for both compounds are shown in Supplementary Table 1), and they have been detected in surface water, groundwater, estuaries, and coastal waters[16-18]. Most pharmaceuticals are traditionally considered pseudo-persistent pollutants due to the continuous release from various sources that exceed the natural degradation rate (half-life time for DCF and IBU are reported in Supplementary Table 1). Hernández-Tenorio and coauthors[19] reviewed the occurrence of DCF and IBU in different aqueous matrices (surface water, WWTPs influents, hospital wastewater, groundwater, drinking water, and raw water) worldwide, reporting a wide range of concentrations of both compounds: 0.08-19,300 ng/L for DCF (0.08-44 ng/L drinking water, 0.1-19,300 ng/L for surface water, 6-2,770 ng/L groundwater, 12-15,087 raw water, 26-10,340 ng/L WWTPs influents, and 40-3,040 ng/L hospital wastewater) and 3.7-141,000 ng/L for IBU (3.7-6,297 ng/L for surface water,
The available information about the IBU and DCF occurrence in aquatic environments and their effects on organisms is mainly focused on freshwater environments. At the same time, the database about seawater systems and species is scarce[22,23]. Invertebrates represent the overwhelming majority of animals living in marine systems, both in terms of biomass and number of species, with worldwide distribution and extraordinary ecological and biological diversity. They have a key role in the ecosystem functioning, participating in nutrient recycling and the transfer of energy from primary producers and higher levels of the food web[24,25]. Additionally, many species are economically and commercially relevant, being widely used for human consumption. The ability of these organisms to accumulate a wide variety of contaminants present in the aquatic environments means that they play an important role in the transfer of contaminants along the food web, posing a risk to both animal and human health. All these characteristics, coupled with high sensitivity to environmental stresses, small size, short life cycle, relatively easy to culture and manipulate in the laboratory or collection in the field, and the existence of standardized protocols, make invertebrates widely used in field monitoring and laboratory studies of contaminant toxicity[26-28].
This work summarizes and analyses the information available in the literature about DCF and IBU in terms of occurrence and effects. First, we analyzed the environmental occurrence in water and sediments from coastal and estuarine areas, as well as open seawater, to identify the range of concentrations in which the two compounds occur and the areas of particular interest, especially those with high levels of DCF and IBU. The occurrence in the marine invertebrate was also evaluated to assess the bioconcentration potential of DCF and IBU, considering laboratory and field studies. Finally, we examined the possible effects induced by the two pharmaceuticals in marine invertebrates at different organization levels, encompassing acute effects at the organism level to sublethal alterations at the sub-cellular level (biochemical, genetic, protein expression, etc.). These data are essential for understanding the toxicity of selected compounds, determining the concentrations at which the different effects occur, elucidating the possible mechanisms of action, and the risks associated with environmental exposure. A systematic literature search was conducted from September 2022 to December 2022 using the Google Scholar, Scopus and ScienceDirect databases, focusing on scientific publications from 2004 to 2023. A total of 107 studies were included in the database used for this review. For each chemical, we used different combinations of keywords, including ibuprofen, diclofenac, occurrence, marine, aquatic, invertebrates, effects, bioaccumulation, toxicity, and risk assessment. The risk associated with the presence of DCF and IBU in the coastal and estuarine ecosystems was studied using data from the literature, along with risk quotients calculated based on the data compiled in this review. Individual and mixture potential risks have been considered, along with exploring how new approaches (e.g., adverse outcome pathway, AOP) can improve the risk assessment.
OCCURRENCE
The presence of pharmaceuticals and their metabolites has been reported in aquatic ecosystems worldwide, with a wide range of concentrations (ranging from µg/L to ng/L). WWTPs are the most relevant sources of these contaminants. Adeleye and coauthors[5] reviewed the occurrence of pharmaceutical compounds in wastewaters around the world, observing that analgesics, particularly acetaminophen, ibuprofen, diclofenac, aspirin, and ketoprofen are commonly detected in wastewaters and occur at very high concentrations. The highest concentrations have been reported to be 1,090,000 ng/L for acetaminophen in Kenya, 603,000 and 8,560 ng/L, respectively, for ibuprofen and ketoprofen in Spain, 115,100 ng/L for diclofenac in South Africa, and 1,407,000 for aspirin in South Korea. As indicated above, WWTPs are designed to remove nutrients, pathogens, and particulate matter from influents, and typically, specific treatments for pharmaceutical removal were not available. In addition to the type and conditions of removal treatment, numerous factors influence the removal percentage and the environmental presence of drugs. These factors include the physicochemical properties of compounds, climatic conditions (precipitation and temperature), population density, pharmaceutical consumption rate, and the presence of hospitals and drug manufacturers. Hence, the variation in these factors results in drug presence to vary according to the compound, country, and season[29-31]. Adeleye and coauthors[5] indicated that the concentrations of DCF and IBU in WWTP effluents vary between 0.1-15,000 ng/L and 1-21,000 ng/L, respectively, with the highest concentrations detected in South Africa and Korea for both compounds.
Pharmaceuticals and their metabolites enter surface water and are transported by rivers, reaching estuaries and, finally, the sea, where the concentrations decrease significantly due to dilution effects, sorption to sediments, and transformation processes[32-34]. In recent years, several reviews on pharmaceutical levels in marine environments have been published[5,27,35,36]. The concentrations in seawater (estuary, bays, coastal, and offshore) and sediment are summarized in Table 1.
Occurrence of DCF and IBU in the marine environment (seawater and sediment)
Drug | Country | Studysite | Matrix | Study period | Concentration | Analytical method | LOQ (ng/L) | LOD (ng/L) | Refs |
(aqueous matrix: ng/L, sediment: ng/g dw) | (aqueous matrix: ng/L, sediment: ng/g dw) | ||||||||
DCF | Germany | Baltic Sea | Nr | 2009 (May) | 9.2 | LC-MS/MS | 2 | [37] | |
Poland | Baltic Sea (Gulf of Gdańsk) | Estuary seawater | 2012 | 92.6 | LC-MS/MS | 0.5 | 0.2 | [38] | |
Poland | Baltic Sea (Gulf of Gdańsk) | Coastal seawater | 2012 (October and December) | 79-102 | GC-MS | 9 | 3 | [39] | |
Poland | Baltic Sea (Bay of Puck) | Coastal seawater | 2017-2018 | 196 | LC-MS/MS | 1 | 0.3 | [40] | |
UK | North Sea (Humber estuary) | Estuary seawater | 2016-2017 | 32.35-205.8 | UHPLC-MS/MS | 5.91 | 1.77 | [41] | |
UK | North Sea (North Scotland) | Nr | 2005 (November) | < 0.12 | LC-MS/MS | 0.41 | 0.12 | [42] | |
Belgium | North Sea | Coastal seawater | 2017 (February and April) | 10 | UHPLC-Q-Orbitrap-HRMS | 0.4 | [43] | ||
Harbor seawater | 12.3-69 | ||||||||
Norway | Arctic regions | Seawater | 2007 | 1-48 | nr | nr | nr | [44] | |
Antarctica | Antarctic Peninsula | Coastal seawater | 2012-2013 | 77-15,087 | LC-MS/MS | 14.3 | 4.3 | [45] | |
Ireland | East and West coast of Ireland | Coastal seawater | 2011-2012 | 0.06-0.55 | LC-MS/MS | 22 | [46] | ||
France | Seine estuary | Estuary seawater | 2002 (from March to November) | 7.7-63.4 | GC-MS | 0.5 | [46] | ||
France | Mediterranean Sea | Coastal seawater | Nr | < 1500 | GC-MS | 2.6 | [47] | ||
Portugal | Arade river estuary | Estuary seawater | 2010 (from August to November) | 4-31 | LC-MS/MS | nr | nr | [48] | |
Portugal | Atlantic Ocean | Coastal seawater | 2013 (from June to September) | 1.14-241 | UHPLC-MS/MS | 0.02 | [49] | ||
Portugal | Atlantic Ocean (Oporto) | Coastal seawater | 2013 (July) | 3-33 | UHPLC-MS/MS | 0.02 | 0.06 | [50] | |
Spain | Atlantic Ocean (Cadiz Bay and Huelva estuary) | Coastal seawater | 2011(October) | 3.1-27.6 | UHPLC-MS/MS | 0.2 | 0.1 | [51] | |
Estuary seawater | 0.3-9.2 | ||||||||
Spain | Atlantic Ocean (Bay of Cadiz) | Sediment | 2011 (March and September) | < 0.10-1.5 | UHPLC-MS/MS | < 0.1 | [52] | ||
Spain | Atlantic Ocean (Gran Canaria Island) | Coastal seawater | 2011-2012 | 23.7-343.6 | LC-MS/MS | 4.6 | 1.4 | [53] | |
Spain | Atlantic Ocean | Sediment | 2014 (summer) | 10 | GC-MS | 0.7 | [54] | ||
Spain | Atlantic Ocean (Bay of Cadiz) | Coastal seawater | 2015 (summer) | 1.5-24.3 | UHPLC-QqQ-MS/MS | 0.2 | 0.1 | [55] | |
Ocean seawater | 0.3-2.5 | ||||||||
Spain | Mediterranean Sea | Coastal seawater | Nr | 4 | UHPLC_MS/MS | 2.4 | 0.7 | [56] | |
Israel | Mediterranean Sea | July 2009 and January 2011 | 6.1 | LC-MS/MS | 2.0 | [38] | |||
Tunisia | Mediterranean Sea | Coastal seawater | 2017-2018 | 3-23 | UHPLC-MS/MS | nr | nr | [57] | |
Italy | Mediterranean Sea | Open seawaters | 2014 (November) | 0.02 | UHPLC-MS/MS | 0.02 | [58] | ||
Italy | Ionian Sea (Augusta Bay) | Coastal seawater | October 2017 and April 2018 | 0.5-441 | LC-MS/MS | 0.38 | [59] | ||
Sediment | 1.1 | 0.56 | |||||||
Greece and Turkey | Aegen Sea and Dardanelles | 2010, 2011 | 9.7 | LC-MS/MS | 2.0 | [38] | |||
Greece | Aegean Sea | Offshore and coastal seawater | 2013 (December) | < 4.1-16.3 | UHPLC-MS/MS | 4.1 | 1.4 | [60] | |
Greece | Aegean Sea (Lesvol Island) | Coastal seawater | 2014 (April and May) | 2.2-14 | LC-MS/MS | 2.0 | 0.7 | [61] | |
Turkey | Sea of Marmara | Coastal seawater | 2019 (April and October) | < 27-1,300 | LC | 93 | 28 | [62] | |
Turkey | Sea of Marmara (Golden Horn Estuary) | Estuary seawater | 2019-2020 | 110-1,120 | LC | 92 | 27 | [63] | |
Sediment | < 30.6 | 102 | 30.6 | ||||||
Saudi Arabia | Red Sea | Coastal waters | 2016 (March and May) | 1.6-10,221.1 | LC-MS/MS | 1.60 | [4] | ||
Taiwan | China Sea | Coastal seawater | 2009 (June and July) | 2.42-57.10 | LC-MS/MS | 2.5 | [64] | ||
China | China Sea (Jiulong River) | Estuary seawater | 2014 (from March to December) | 0.819-11.0 | LC-MS/MS | 0.1 | [65] | ||
China | Pearl River Delta | Sediment | October 2014 and March 2015 | nd-0.03 | UHPLC-MS/MS | 0.1 | 0.03 | [66] | |
Malaysia | China Sea (PulauKukup) | Sediment | Nr | < 0.093-0.228 | LC-MS/MS | 0.062 | 0.093 | [67] | |
Singapore | Indian Ocean | Nr | Nr | 4-38 | UHPLC-MS/MS | 0.93 | [68] | ||
Singapore | Pacific Ocean | Coastal seawater | 2011 (June and July) | < 1.5-11.6 | LC-MS/MS | 1.5 | [69] | ||
Singapore | Estuary seawater | Nr | 10 | LC-MS/MS | 0.01 | [70] | |||
Singapore | Pacific Ocean | Mangrove waters | 2012-2013 | < 0.04-1.2 | LC-MS/MS | [71] | |||
USA | Pacific Ocean (Southern California) | Coastal seawater | 2006- 2007 | 0.6 | LC-MS/MS | 2.5 (Reporting limit) | [72] | ||
Canada | Atlantic Ocean (Nova Scotia) | Marine watershed | 2005 (from May to October) | 4-6 | GC-MS | 3 | [73] | ||
Brazil | Atlantic Ocean (Santos Bay) | Coastal seawater | 2014 (March) | < 7.4-19.4 | LC-MS/MS | 7.4 | 1.0 | [74] | |
Brazil | (Santos Bay) | Coastal seawater | 2016 (December) | 4.01-4.78 | LC-MS/MS | 3.0 | 0.81 | [75] | |
Brazil | Atlantic Ocean (Santos Bay and North Coast of Salvador) | Sediment estuary | Nr | < 0.10-1.06 | LC-MS/MS | 0.1 | [18] | ||
IBU | Germany | Baltic Sea | Nr | 2009 (May) | 109 | LC-MS/MS | 3.6 | [37] | |
Poland | Baltic Sea (Gulf of Gdańsk) | Estuary seawater | 2012 | 34.9 | LC-MS/MS | 10.0 | 3.3 | [38] | |
Poland | Baltic Sea (Gulf of Gdańsk) | Coastal seawater | 2012 (October and December) | 48 | GC-MS | 5 | 2 | [39] | |
UK | North Sea (North Scotland) | Nr | 2005 (November) | < 0.52 | LC-MS/MS | 1.72 | 0.52 | [42] | |
UK | North Sea (Humber estuary) | Estuary seawater | 2016-2017 | 24.93-6,297.14 | UHPLC-MS/MS | 4.83 | 1.45 | [41] | |
Norway | Arctic Ocean | Seawater | 2002 | 0.7 | GC-MS | 0.09 | [76] | ||
Norway | Arctic regions | Seawater | 2007 | 0.4-52 | nr | nr | nr | [44] | |
Norway, Germany and Belgium | North Sea | Coastal and offshore seawater | 2014 (October) | 0.57-22 | LC-MS/MS | 0.28 | [77] | ||
Antarctica | Antarctic Peninsula | Coastal seawater | 2012-2013 | 37-10,053 | LC-MS/MS | 24 | 7.2 | [45] | |
France | Seine estuary | Estuary seawater | 2002 (from March to November) | < 2.0-26.1 | GC-MS | 0.4 | [78] | ||
France | Mediterranean Sea | Coastal seawater | Nr | < 1,600 | GC-MS | 1.7 | [47] | ||
Portugal | Atlantic Ocean | Coastal seawater | 2013 (from June to September) | 1.25-222 | UHPLC-MS/MS | 0.08 | [49] | ||
Portugal | Atlantic Ocean (Oporto) | Coastal seawater | 2013 (July) | 5-110 | UHPLC-MS/MS | 0.26 | 0.08 | [50] | |
Portugal | Arade river estuary | Estuary seawater | 2010 (from August to November) | 9-28 | LC-MS/MS | nr | nr | [48] | |
Spain | Atlantic Ocean (Cadiz Bay and Huelva estuary) | Coastal seawater | 2011 (October) | 2.8-18.3 | UHPLC-MS/MS | 3.5 | 1 | [51] | |
Estuary seawater | 4.3-195.0 | ||||||||
Spain | Atlantic Ocean (Bay of Cadiz) | Sediment | 2014 (summer) | 97.1-101.3 | GC-MS | 0.1 | [54] | ||
Coastal seawater | 10 | 11 | |||||||
Spain | Atlantic Ocean (Bay of Cadiz) | Coastal seawater | 2015 (summer) | 3.5-1,219.7 | UHPLC-QqQ-MS/MS | 3.5 | 1 | [55] | |
Ocean seawater | < 3.5-32.3 | ||||||||
Spain | Mediterranean Sea | Coastal seawater | Nr | 16 | UHPLC-MS/MS | 3.3 | 1 | [56] | |
Israel | Mediterranean Sea | Coastal seawater | July 2009 and January 2011 | 7.5 | LC-MS/MS | 3.6 | [37] | ||
Italy | Mediterranean | Open seawater | 2014 (November) | 0.063-1.08 | UHPLC-MS/MS | 0.056 | [58] | ||
Italy | North Adriatic Sea (Venice) | Coastal seawater | 2010 (May) | 70 | LC-MS/MS | 3.6 | [37] | ||
Italy | Adriatic Sea (Venice) | Coastal seawater | 2011(February and March) | < 0.049-1.146 | UHPLC-QTRAP-MS/MS | 0.049 | [79] | ||
Italy | Ionian Sea (Augusta Bay) | Coastal seawater | October 2017 and April 2018 | 2.7-2,550 | LC-MS/MS | 2.15 | [59] | ||
Greece and Turkey | Aegean Sea and Dardanelles | Coastal seawater | 2010, 2011 | 35 | LC-MS/MS | 3.6 | [37] | ||
Greece | Aegean Sea (Lesvol Island) | Coastal seawater | 2014 (April and May) | 9.4-16 | LC-MS/MS | 3.6 | 1.2 | [61] | |
Turkey | Sea of Marmara | Coastal seawater | 2019 (April and October) | < 15-2,130 | LC | 47 | 15 | [62] | |
Turkey | Sea of Marmara (Golden Horn Estuary) | Estuary seawater | 2019-2020 | 60-2,460 | LC | 50 | 15 | [63] | |
Sediment | < 91-215 | 91 | 27.2 | ||||||
Saudi Arabia | Red Sea | Coastal seawater | 2016 (March and May) | 46.2-508.7 | LC-MS/MS | 26.7 | [4] | ||
Estuary seawater | 0.261 | ||||||||
Taiwan | China Sea | Coastal seawater | 2009 (June and July) | < 2.50-53.60 | LC-MS/MS | 2.5 | [64] | ||
Taiwan | China Sea | Coastal seawater | 2010 (October) | 12.1 | LC-MS/MS | 2.5 | [80] | ||
Taiwan | China Sea (Xiamen Bay) | Coastal seawater (bay and estuary) | 2019 (August) | 9.4 | LC-MS/MS | 3.1 | 0.92 | [81] | |
China | Jiulong River | Estuary seawater | 2014 (from March to December) | < 0.1-20.7 | LC-MS/MS | 0.1 | [65] | ||
Malaysia | China Sea (Kota Kinabalu) | Coastal seawater | 2018- 2019 | 10.2-42.7 | LC-MS/MS | 3.1 | 0.92 | [82] | |
Singapore | Pacific Ocean | Coastal seawater | 2011 (June and July) | < 2.2-9.1 | LC-MS/MS | 2.2 | [69] | ||
Singapore | Indian Ocean | Nr | Nr | 41-121 | LC-MS/MS | 1 | [68] | ||
South Africa | Indian Ocean (Durban coast) | Coastal seawater | 2018 (September) | 0.166 | LC | 35 | 11 | [83] | |
USA | Pacific Ocean (San Francisco Bay) | Coastal seawater | 2010 (February) | 12 | LC-MS/MS | 3.6 | [37] | ||
USA | Salish Sea (Bellingham Bay) | Sediment | 2010 (April and June) | 21.70 | LC-MS/MS | 21.7 | [84] | ||
USA | Pacific Ocean (Southern California) | Coastal seawater | 2006-2007 | 30 | LC-MS/MS | 50 (Reporting Limit) | [72] | ||
Canada | Atlantic Ocean (Nova Scotia) | Marine watershed | 2005 (from May to October) | 3-230 | GC-MS | 6 | [73] | ||
Brazil | Atlantic Ocean (Santos Bay) | Coastal seawater | 2014 (March) | 326.1-2,094.4 | LC-MS/MS | 35 | 10 | [74] | |
Brazil | Atlantic Ocean (Santos Bay and North Coast of Salvador) | Sediment estuary | Nr | 0.77-18.8 | LC-MS/MS | 0.1 | [18] |
DCF and IBU presence in saltwater has been reported worldwide, although most available data correspond to the North Hemisphere and the European coasts. Very little information has been found on the occurrence and distribution of DCF and IBU in South America, Africa, and Asia. Waleng and Nomngongo[83] highlighted the growing concern about pharmaceutical contamination in Africa and Asia due to the increasing pharmaceutical consumption rate, improper wastewater treatment resources, reckless use and disposal of pharmaceuticals, poor sanitation, and lack of drug return programs. Similarly, very little is known about the presence of DCF and IBU in more remote areas such as the Antarctic and Arctic regions. Only four research works can be found in the bibliography on this subject[44,45,76,85].
Estuaries are transition areas between freshwater and seawater environments with high ecological and economic relevance, as they provide habitat for many species and recreation activities. They receive water from one or more rivers, acting as a confluence for contaminants, particularly pharmaceuticals, which pose a potential risk to these systems[86].
In European estuaries, the concentrations of DCF and IBU are between 0.3-1,120 ng/L and <
Also, in coastal seawater, wide variations have been reported for both compounds. The concentrations ranged between 0.06-10,221.1 ng/L for DCF and 0.049-2,550 ng/L for IBU. The highest values recorded in this matrix [Table 1] correspond to the Red Sea (10,221.1 ng/L, Saudi Arabia) for DCF and to the Augusta Bay (2,550 ng/L, Italy, Ionic Sea), the Santos Bay (2,094.4 ng/L, Brazil, Atlantic Ocean), and the Sea of Marmara (2,130 ng/L, Turkey) for IBU[4,59,62,74]. As for estuaries, the high levels recorded for both drugs in these areas have a common cause: the discharge of untreated or insufficiently treated wastewater into the coastal water. A direct discharge of municipal raw sewages occurs in Augusta Bay[59], while both Santos Bay and Red Sea wastewaters are insufficiently treated. This is due to the absence of primary and secondary treatments in the Brazilian WWTPs[74] and to the overload of the WWTPs treatment capacity. The overload is a consequence of the development and the demographic increase that the eastern coast of the Red Sea has suffered in the last decades[4]. In the case of the Sea of Marmara, in addition to the presence of deep-sea discharge points of municipal WWTPs, hydrodynamic factors, such as a low rate of mixing and renewal of water masses, play an important role in the accumulation of selected compounds in the coastal waters[62].
Both pharmaceuticals were also detected in coastal waters of remote areas, such as the Antarctic Peninsula, isolated from human influence. González-Alonso et al. reported concentrations of 15,087 and 10,053 ng/L for DCF and IBU, respectively, in seawater samples collected from Hope Bay[45]. It is important to note that the samples were taken at a discharge point for urban wastewater into the sea, which explains the high recorded values.
The variability observed for both coastal and estuary concentrations of DCF and IBU could also be related to the seasonal fluctuation of drug levels. Studies reported in Table 1 refer to both punctual sampling and series of sampling over a period of time (one or more months, one year, etc.). It is known that variations in pharmaceutical concentrations in aquatic environments are often related to changes in usage (higher usage in winter to treat seasonal illnesses) and local environmental conditions (lower concentrations in the wet season due to dilution by precipitation)[41,49,87].
Data on DCF and IBU presence in open seawaters are scarce. As expected, the concentration ranges of both compounds reported in Table 1 (0.02 to 16.3 ng/L for DCF and 0.063 to 32.3 ng/L for IBU) are smaller compared to those observed in coastal and estuarine waters when considering sorption, degradation, and dilution[55].
In aquatic systems, pharmaceuticals are subjected to sorption processes on sediment that depend on the properties of the sediments (e.g., organic matter content and pH values) and the physicochemical properties of pharmaceuticals (e.g., dissociation constant pKa and octanol-water partition coefficient, log Kow). According to the classification reported by Rogers[88], an octanol-water partition coefficient (log Kow) below 2.5 denotes low sorption, while the range of 2 < log Kow < 4 signifies medium sorption, and log Kow above 4 indicates high sorption. The octanol-water partition coefficients of DCF and IBU are 4.51 and 3.97, respectively (https://pubchem.ncbi.nlm.nih.gov). Hence, DCF and IBU sorptions on sediment are high and medium, respectively, which allows them to be detected in both water and sediment[63]. Similarly, the pKa values, ranging from 3.99 to 4.3 for DCF and from 4.45 to 5.3 for IBU, indicate that both compounds in seawater (pH close to 8.2) could be negatively ionized, showing low sorption on the surface sediments[59,89]. Very few studies have been conducted to determine the concentration of DCF and IBU in marine sediments. The highest values for both compounds were found in sediments from the Golden Horn estuary in the Sea of Marmara (< 30.6 ng DCF/g dw and < 91-215 ng IBU/g dw, as reported in Table 1), which can be related to high levels of DCF and IBU present in seawater, as previously reported[63]. Other studies were carried out in bays from different world areas, including the Atlantic Ocean (Bay of Cádiz in Spain and Santos Bay in Brazil), the Ionic Sea (Augusta Bay, Italy), the Pacific Ocean (Bellingham Bay in the Salish Sea, USA), and the South China Sea [Table 1]. DCF concentrations measured at a depth between 0 and 10 cm are similar in all study areas, ranging from < 0.093 to 1.5 ng/g dw, except Cadiz Bay, where a value of
BIOACCUMULATION
The DCF and IBU concentrations vary widely in water and sediments depending on the sampling area [Table 1]. One must consider the exposure, physicochemical conditions, and lifestyles involved to assess the hazardous effects of both compounds in their respective environmental compartments. While avoidance is an option for pelagic species to reduce exposure, it is a limited option for benthic species. Consequently, establishing a general pattern regarding the preferential exposure of pelagic or benthic marine invertebrates to NSAIDs is not an easy task, as it depends on several variables that need to be considered. In Tables 2 and 3, we have summarized data on DCF and IBU concentrations in various marine invertebrates from laboratory studies [Table 2] and field studies [Table 3].
Bioconcentration factors for DCF and IBU in invertebrate marine species (all species included in the table belong to the Mollusca phylum) under laboratory-controlled exposure (in vivo assays)
Drug | Species | Exposure condition | Drug concentrations in organisms | Analytical method | LOQ (ng/g) | LOD (ng/g) | BCF | Refs |
DCF | Mytilus galloprovincialis | Exposure: 0.5 μg/L, 14 d | 1.19 ng/g ww | LC | 1 | 2.4 L/kg ww | [90] | |
Exposure: 1 and 100 μg/L, 3 d | 6.5 and 520 ng/g ww (1.0 and 100 μg/L respectively) | LC-MS | 10 | 6.84 and 5.0 L/kg ww (1.0 and 100 μg/L respectively) | [91] | |||
Exposure: 1 μg/L, 28 d | 7.1 ng/g ww | LC | 15 | 5 | 7.4 L/kg ww | [92] | ||
Exposure: 1 μg/L, 28 d | 9.7 ng/g ww | LC | 5 | 10.1 L/kg ww | [93] | |||
Exposure: 2.5 μg/L, 60 d | 0.41, 0.91, 0.56 ng/g ww (respectively at 14, 30 and 60 d) | LC | 1 | 0.16, 0.36, 0.22 L/kg ww (respectively at 14, 30 and 60 d) | [94] | |||
Exposure: 10.0 ± 1.6 μg/L, 58 d | 132.6 ± 16.6 ng/g ww (visceral mass) | LC-MS/MS | 29 | 13 L/kg ww | [95] | |||
Exposure: 25 μg/L, 14 d | 14.90 ± 7.89 ng/g ww | LC | 1 | 0.28-0.912 L/kg ww* | [26] | |||
Exposure: 100 and 600 μg/L, 7 d | 502 and 1,836 ng/g ww (100 and 600 μg/L respectively) | LC-MS | 1 | 16.3 and 11.03 L/kg ww (100 and 600 μg/L respectively) | [96] | |||
Mytilus trossulus | Exposure: 1 and 10,000 μg/L, 8 d | 180 and 82,000 ng/g ww (respectively at 1 and 10,000 μg/L) | LC-MS/MS | nr | nr | 180 and 10 L/kg ww (respectively at 1 and 10,000 μg/L) | [97] | |
Exposure: 133.3 μg/L, 5 d | 2.22 ng/g dw | GC-MS | 20 | 10 | 57.43 L/kg dw | [98] | ||
Ruditapesdecussatus | Exposure: 1 μg/L, 7 d | 24.3 ng/g dw | LC | 5 | 24.3 L/kg dw | [99] | ||
Ruditapesphilippinarum | 60.3 ng·g-1 dw | 60.3 L/kg dw | [99] | |||||
IBU | Crassostrea gigas | Exposure: 1 and 100 μg/L, 7 d | 0.2 and 29.4 ng/g ww (respectively at 1 and 100 μg/L) | LC | nr | nr | 0.2 and 0.294 L/kg ww (respectively at 1 and 100 μg/L)* | [100] |
Mytilus galloprovincialis | Exposure: 2.5 μg/L, 60 d | 6.4, 6.1, 11.3 ng/g ww (respectively at 14, 30 and 60 d) | LC | 0.5 | 2.6, 2.5, 4.5 L/kg ww (respectively at 14, 30 and 60 d) | [94] | ||
Exposure: 25 μg/L, 14 d | 1.63 ± 1.00 ng/g ww | LC | 0.025-0.105 L/kg ww* | [26] |
The concentration of DCF and IBU in invertebrate marine species (all species included in the table belong to the Mollusca phylum) from field studies
Drug | Species | Study location | Study period | Detected concentration | Analytical method | LOQ (ng/g) | LOD (ng/g) | Refs |
DCF | Anomalocardiabrasiliana | Northeast of Brazil (estuary and coastal areas) | July-August 2019 | nd-2.1 ng/g ww | LC-MS | 0.81 | 0.24 | [101] |
Mytilus edulis | Northeast of Brazil (estuary and coastal areas) | July-August 2019 | nd-3.0 ng/g ww | LC-MS | 0.81 | 0.24 | [101] | |
Mytilus galloprovincialis | Atlantic Ocean (Portugal, coastal areas) | January-October 2015 | 0.5-4.5 ng/g dw | LC-MS/MS | 0.5 | 0.2 | [102] | |
Central Adriatic Sea (Portonovo Bay, coastal areas) | July, August and September 2014 | 16.11 ± 14.72 ng/g dw | LC | 1 | [26] | |||
Adriatic Sea (Italy, coastal areas) | 2014-2017 | < LOQ-231 ± 67.2 ng/g dw | LC | 1.37 | [103] | |||
Tyrrhenian Sea (Italy, coastal areas) | 2014-2017 | < LOQ-280.1 ± 161.8 ng/g dw | LC | 1.37 | [103] | |||
Northwest Adriatic Sea (Italy, coastal lagoon)a | 2.1-4.6 ng/g ww | GS-MS | 1 | [104] | ||||
Mytilus trossulus | Gulf of Gdansk (southern Baltic Sea) | 560 ± 130 ng/g dw | GC-MS | 5 | 2 | [105] | ||
Neritealineata | Klang River (Malaysia, estuary) | 0.93-7.41 ng/g ww | LC-MS | 0.35 | [106] | |||
Ruditapesphilippinarum | Ria Formosa lagoon (Portugal, coastal lagoon) | June to July 2016, 2017, and 2018 | 1.5-1.6 ng/g ww | LC-MS/MS | 0.02 | 0.006 | [107] | |
IBU | Anomalocardia brasiliana | Northeast of Brazil (estuary and coastal areas) | July-August 2019 | nd-8.2 ng/g ww | LC-MS | 0.72 | 0.22 | [101] |
Mytilus edulis | Northeast of Brazil (estuary and coastal areas) | July-August 2019 | nd-5.4 ng/g ww | [101] | ||||
Mytilus galloprovincialis | Central Adriatic Sea (coastal areas) | July, August and September 2014 | 9.39 ± 0.59 ng/g dw | LC | 0.5 | [26] | ||
Adriatic (Italy, coastal areas) | 2014-2017 | < LOQ-143.7 ± 242 ng/g dw | LC | 8 | 2.4 | [103] | ||
Tyrrhenian Sea (Italy, coastal areas) | 2014-2017 | < LOQ-22.99 ± 26.01 ng/g dw | LC | 8 | 2.4 | [103] | ||
Mytilus trossulus | Gulf of Gdansk (southern Baltic Sea) | 730 ± 290 ng/g dw | GC-MS | 3 | 1 | [105] | ||
Ostrea lurida | Coos and Netarts Bays (Oregon coastal estuaries) | Summer 2013, fall 2013, spring 2014 | nd-10.5 ng/g ww | LC-MS/MS | nr | nr | [108] | |
Ruditapesphilippinarum | Ria Formosa lagoon (Portugal, coastal lagoon) | June to July 2016, 2017, and 2018 | 0.9-1 ng/g ww | LC-MS/MS | 1.05 | 0.32 | [107] |
The analytical method employed for the bioaccumulation assessment of both compounds has mainly been LC-MS, as shown in Tables 2 and 3. However, some results have been obtained using LC and GC-MS. The reported differences for LOD or LOQ vary based on the analytical technique employed. Thus, lower detection limits have been reported for both compounds when using LC-MS or LC-MS/MS.
Laboratory assays evaluate the pharmaceutical accumulation capacity in target organisms under controlled conditions. The knowledge generated with this type of assay can help us to understand the mechanisms of drug accumulation (e.g., toxicokinetic and toxicodynamic properties), identify hazards associated with exposure, and provide a basis for understanding the accumulation in the field.
According to the EU regulation 253/2011[109], the bioconcentration factor (BCF) represents the compound accumulation in the whole body solely from the water. Its value can be used to identify and classify bioaccumulative substances. A chemical is considered bioaccumulative when its BCF is greater than 2000 and classified as very bioaccumulative when its BCF exceeds 5000. According to the BCF data reported in Table 2, DCF and IBU can be considered substances with low bioconcentration potential in mollusks. The same conclusion can be reached by evaluating the logKow. The logKow values for DCF and IBU are 4.26 and 3.97, respectively, which are below the threshold limit (log Kow ≥ 4.5) indicated by the European Medicines Agency for the identification of bioaccumulative substances in human pharmaceuticals[110].
Bivalves, particularly mussels, were widely used to test pharmaceutical bioaccumulation[98]. The DCF accumulation in bivalve tissues has been studied in Mytilus and Ruditapes species, highlighting their differences [Table 2]. The resulting BCF values were 6.84-10.1, 180, 24.3, and 60.3 L/kg ww for Mytilus galloprovincialis, Mytilus trossulus, Ruditapes decussatus, and Ruditapes philippinarum, respectively, suggesting the existence of different mechanisms of drug uptake, detoxification, and excretion mechanisms in different species. Most studies have focused on Mytilus galloprovincialis, and they have tested different concentrations and exposure times. The trend in the DCF accumulation seems to be directly proportional to the exposure concentration. The BCF values varied from 2.4 after 14 days of exposure at 0.5 μg/L of DCF[90] to 16.3 L/kg ww after 7 days of exposure at 100 μg/L[96]. However, some authors observed a decrease in BCF values at higher exposure concentrations. Mezzelani et al.[26] obtained BCF values ranging between 0.28 and 0.912 L/kg ww for mussels exposed at 25 μg/L of DCF for 14 days, and Bonnefille et al.[96] observed that exposure to 600 μg/L of DCF during 8 days resulted in a BCF of 10 L/kg ww, a lower value compared to
In general, information about bioaccumulation in invertebrates in the field is scarce and primarily focused on mollusk species. This focus is likely due to their sedentary lifestyle, which facilitates their sampling[113] and allows researchers to obtain data representative of the area where they have been collected.
In freshwater, the residues of NSAIDs detected in invertebrates are relatively higher than those in other pharmaceutical classes. The most frequent NSAIDs detected are DCF and IBU[114]. Swiacka and coauthors[28] reviewed the occurrence of pharmaceuticals in biota from freshwater and seawater environments. They concluded that antibiotics and NSAIDs are the most relevant classes detected in marine biota, reaching concentrations above 500 ng/g. In our bibliographic review, we found concentrations in this range in Mytilus trossulus from the Gulf of Gdansk in the Baltic Sea for both DCF and IBU (560 ± 130 ng/g dw and 730 ± 290 ng/g dw, respectively, as shown in Table 3). These levels reflect the high concentrations detected in seawater, as discussed in Section 2. Swiacka and coauthors[28] indicated that according to UNESCO and HELCOM[115], the Baltic Sea is one of the most contaminated seas in the world, with anti-inflammatories and analgesics being the substances of greatest concern. Within these groups, DCF, IBU, and paracetamol are the most frequently detected compounds in all seawater compartments.
High DCF and IBU concentrations of 109-280.1 ng/g dw and 143.7 ng/g dw, respectively, have also been detected by Mezzelani and coauthors[26,103] in Mytilus galloprovincialis from the Adriatic and Tyrrhenian coastal areas during 2014-2017. They sampled different sites along the coast, including natural parks, mussel farms, touristic areas, etc., and detected both compounds in all sites, with a higher frequency of detection for DCF compared to IBU (31.15% vs. 9.35%). These authors detected the most elevated values in tourist areas with recreational beaches, mussel farms, and canal harbors. Additionally, they recorded seasonal changes with higher levels in spring and summer, which they related to the greater anthropogenic pressure in tourist areas.
Both compounds were also detected in mollusk species from estuaries and coastal areas in Portugal[102,107], Brazil[101], the USA[108], and Malaysia[106], with concentrations in the low nanogram per gram range (under
EFFECTS
As previously indicated, aquatic invertebrates are continuously exposed to active substances released into the environment, which accumulate in their tissues, producing a wide range of effects. These effects include acute effects, which are less likely and usually occur during accidental discharge of drugs, and chronic effects, which are related to prolonged exposure to different concentrations (generally low) of contaminants over an extended period of time.
Studies published between 2010 and 2023 on the toxic effects of DCF and IBU on marine invertebrates under laboratory-controlled exposure are summarized in Tables 4 and 5, respectively. The toxicities of both compounds have been assessed on diverse invertebrate species, including crustaceans, echinoderms, mollusks, and polychaeta.
Ecotoxicological effects of DCF in marine invertebrate species under laboratory conditions
Taxonomic | Species | Exposure concentrations (μg/L) | Exposure time | Tissue | Endpoints | Effects | Refs |
Crustacean | Carcinus maenas | 0.01, 0.1 | 7 d | Hemolymph | Physiology | ↑ hemolymph osmolality and osmoregulation capacity (both concentrations) | [116] |
Crustacean | Palaemon serratus | 36, 900 | 50 d | Larvae | Embriotoxicity | ↑ specific growth rate (LOEC: 900 μg/L) | [117] |
Crustacean | Siriella armata | 250, 20,000 | 2, 4 d | Neonates | Mortality | NOEC: 8,000 (2 d) and 250 (4 d) μg/L LOEC: 10,000 (2 d) and 500 (4 d) μg/L LC50: 10.43 μg/L (2 d) and 2,919 μg/L (4 d) | [118] |
Crustacean | Tisbe battagliai | 0-120,000 | 2 d | Nauplii | Mortality | LC50: 9,500 μg/L (2 d) | [119] |
2 d | LC50: 15,800 μg/L (2 d) | [120] | |||||
Echinoderm | Arbacia lixula | 50, 500, 5,000, 50,000 | 180 min | Embryos | Embryotoxicity | ↓ number of correctly developed embryos (50,000 μg/L) | [121] |
Gametes | Reproduction | ↑ number of degenerated eggs (5,000 and 50,000 μg/L) | |||||
Echinoderm | Asterias rubens | 0.01, 0.1, 1, 10, 100, 1,000 | 120 min | Gametes | Reproduction | ↓ sperm motility (≥ 1 from 20 min) and swimming speed (≥ 0.1 from 20 min), EC50 (60 min): 2,335.8 μg/L ↓ % fertilization, EC50 (60 min): 616.48-2610 μg/L | [122] |
Echinoderm | Parocentrotus lividus | 12.5, 125, 1,250, 12,500 | 2 d | Embryos | Embryotoxicity | ↓ larval length (all exposure concentrations) ↑ abnormal development (all exposure concentrations) | [123] |
Echinoderm | Psammechinus miliaris | 0.01, 0.1, 1, 10, 100, 1,000 | 120 min | Gametes | Reproduction | ↓ sperm motility (exposure concentrations ≥ 1, after 20 min), EC50 (60 min): 378.22 μg/L ↓ % fertilization, EC50 (60 min): 247.31-429.37 μg/L | [122] |
Mollusc | Mytilus edulis | 1, 1,000 | 1, 4 d | Digestive gland | Oxidative stress | ↑ GST, (4 d, 1 and 1,000 μg/L), LPO (↓ 1 d and ↓ 4 d, 1 and 1,000 μg/L) | [120] |
Genotoxicity | ↑ DNA damage (4 d, 1,000 μg/L) | ||||||
1, 1,000 | 14 d | Digestive gland | Oxidative stress | ↓ GST (1,000 μg/L) | [124] | ||
down-regulation of GADPH (1 and 1,000μg/L, 14 d) upregulation of BRAFLDRAFT_282392 (1 and 1,000 μg/L, 7 and 14 d), class 1 ADH (1,000 μg/L, 7 d) | |||||||
200, 1,000 | 7 d | Digestive gland | Oxidative stress | ↓ GR, CAT (1,000 μg/L) | [125] | ||
gills | oxidative stress | ↑ GR (1,000 μg/L) | |||||
oxidative stress, alteration of energy metabolism, cytoskeleton, protein modification and transport | oxidation and changes in protein abundance (caspase, HSP, SOD, etc.) | ||||||
Mollusc | Mytilus galloprovincialis | 1, 10 | 2 d | Larvae | Embryotoxicity | shell deformation and disturbance in shell growth (both exposure concentrations) | [126] |
alteration in gene expression (genes involved in shell formation and biotransformation) (both exposure concentrations) | |||||||
0.01, 0.1, 1, 10, 100, 1,000 | 2 d | Larvae | Embryotoxicity | ↑ shell malformations and ↓ embryo development (LOEC: 0.01 μg/L) | [127] | ||
1 | 28 d | Whole body | Oxidative stress and detoxification | ↑ SOD, CAT, GPx and GST, ↓ LPO and GSH/GSSG ratio | [92] | ||
0.250 | 15 d | Digestive gland | Oxidative stress | ↑ SOD (7 d), ↑ GST (7 d), CAT (3d and ↓ 15 d), and LPO (↑ 3 d and ↓ 7 d) | [128] | ||
Gills | Oxidative stress | ↑ SOD (3 d), ↑ GR (3 d), ↓ LPO (7 d) | |||||
Neurotoxicity | AChE (↑ 3 and 7 d) | ||||||
Gonads | Vitellogenesis/endocrine disruption | female: ↑ ALP (3 and 7 d) male: ↑ ALP (3 d) | |||||
0.5 | 14 d | Digestive gland | Lysosomal responses | ↑ lipofuscin, ↓ neutral lipids | [90] | ||
1, 100 | 3 d | Digestive gland, gills | DCF mechanism of action | ↓ PG biosynthesis (both exposure concentrations) | [91] | ||
100 | 7 d | Digestive gland | Metabolism alteration | tyrosine and tryptophan metabolism modulation, steroid hormone biosynthesis modulation | [129] | ||
0.005, 0.01, 0.1 | 60 min | Hemocytes | Oxidative stress | ↑ LPO (allexposureconcentrations) | [130] | ||
Immunotoxicity | ↓ LMS (all exposure concentrations) | ||||||
Genotoxicity | ↑ DNA damage (0.005 and 0.01 μg/L) | ||||||
Cytotoxicity | ↑ ∙O2- and NO (all exposure concentrations) | ||||||
2.5 | 14, 30 and 60 d | Hemocytes | Immunotoxicity | ↓ granulocytes/hyalinocytes ratio (from 30 d) | [11] | ||
Genotoxicity | ↑ DNA fragmentation (14 and 60 d) and MN frequency (30 d) | ||||||
25 | 14 d | Hemocytes | Immunotoxicity | ↓ LMS | [26] | ||
Genotoxicity | ↑ DNA fragmentation, ↑ MN frequency | ||||||
Mollusc | Mytilus trossulus | 1, 100, 1,000, 5,000, 10,000 | 21 d | Physiology | ↓ byssus strength and threads abundance (LOEC: 10,000 μg/L) | [97] | |
↓ scope for growth (LOEC: 100 μg/L) | |||||||
↑ mortality (LOEC: 10,000 μg/L) | |||||||
energy balance alteration (LOEC: 100 μg/L) | |||||||
4, 40 | 25 d (12 d exposure + 12 d depuration) | Various tissues | Tissue abnormality | accumulation of lipofuscin in gills, mantle and digestive gland (4 and 40 μg/L) necrosis (4 and 40 μg/L) gonadal artresia (4 and 40 μg/L) hepatopancreas atrophy (40 μg/L) hepatopancreas vacuolization (4 and 40 μg/L) gill deformations (4 and 40 μg/L) | [131] | ||
68.22 | 7 d | Digestive gland | Tissue abnormality | necrosis, local inflammation, digestive tubules | [132] | ||
Gills | Oxidative stress | ↓ GR ↑ cytosolic protein content | |||||
Tissue abnormality | gill deformations (edema, enlarged gills, inflammation and necrosis) | ||||||
Gonads | Tissue abnormality | atresia, lesions (granulocitoma), inflammation and necrosis | |||||
Mollusc | Perna perna | 10, 100, 1,000, 10,000, 100,000 | 2 d | Larvae | Embryotoxicity | ↑ inhibition of larval development (100000 μg/L) NOEC: 10,000μg/L LOEC: 100,000μg/L IC50: 18,000μg/L | [75] |
0.02, 0.2, 2 | 2, 4 d | Digestive gland | Oxidative stress | ↓ LPO (0.2 μg/L, 4 d) | [75] | ||
Genotoxicity | ↑ DNA damage (2 μg/L, 2 d) | ||||||
DCF mechanism of action | ↑ COX (0.2 μg/L, 4 d) | ||||||
Gills | Oxidative stress | ↓ GST (0.02, 0.2 and 2 μg/L after 2 d, 2 μg/L after 4 d), ↓ GPx (0.2 μg/L after 2 d, 2 μg/L after 4 d), LPO (↑ 0.2 and 2 μg/L after 2 d, ↓ 0.02 and 0.2 μg/L after 4 d) | |||||
Neurotoxicity | ↑ AChE (2 μg/L, 2 d) | ||||||
Xenobiotic biotransformation | ↓ EROD (0.2 μg/L, 4 d) | ||||||
Genotoxicity | ↓ DNA damage (0.2 and 2 μg/L, 2 d) | ||||||
DCF mechanism of action | ↓ COX (0.02 and 0.2 μg/L after 2 d, 0.2 and 2 μg/L after 4 d) | ||||||
0.02, 0.2, 2 | 2, 4 d | Hemocytes | Immunotoxicity | ↓ LMS (all exposure concentrations and times) | [75] | ||
10, 1,000, 31,250, 100,000, 250,000, 1,000,000 | 1 d | Sperm | Reproduction | ↑ inhibition of fertilization LOEC: 31,250 μg/L EC50: 389,000μg/L | [75] | ||
Mollusc | Ruditapes decussatus | 1 | 30 d | Whole body | Oxidative stress | ↑ SOD, CAT and GPx ↓ GSH/GSSG ratio | [99] |
Mollusc | Ruditapes philippunarum | 1 | 30 d | Whole body | Physiology | ↓ respiration rate | [99] |
Metabolic capacity and energy-related metabolism | ↓ protein content ↑ glycogen content | ||||||
Oxidative stress | ↑ GSH/GSSG ratio | ||||||
15 | 14 d | Digestive gland | Oxidative stress and detoxification | ↑ SOD (1d), CAT (1 d), GR (1 and 7 d), GST (1 and 7 d), LPO (1 d) and MT (7 d) ↓ T-GPx (3 h) | [133] | ||
Neurotoxicity | ↑ AChE (1, 7 and 21 d) | ||||||
Gills | Oxidative stress | ↑ SOD (7d) and CAT (7 d) ↓ LPO (14 d) and MT (14 d) | |||||
Neurotoxicity | ↑ AChE (7 d) | ||||||
Polychaete | Arenicola marina | 0.01, 0.1, 1, 10, 100, 1,000 | 120 min | Reproduction | ↓ sperm motility and swimming speed (exposure concentrations higher than 1 μg/L, after 90 min), EC50 (120 min): 106.77 μg/L ↓ % fertilization, EC50 (120 min): 112.61-565.53 μg/L | [122] | |
Polychaete | Hediste diversicolor | 0.5, 1, 2 | 28 d | Whole body | Oxidative stress and detoxification | ↑ GST (1 and 2 μg/L) | [134] |
Ecotoxicological effects of IBU in marine invertebrate species under laboratory conditions
Taxonomic | Species | Exposure concentrations (μg/L) | Exposure time | Tissue | Endpoints | Effects | Refs |
Crustacean | Amphelisca brevicornis | 0.05, 0.5, 5, 50, 500 ng/g* | 10 d | Whole body | Oxidative stress | ↑ DBF (0.05, 0.5 ng/g), GST (0.05, 0.5, 50 ng/g) and GPx (0.05 ng/g) ↓ LPO (0.05 ng/g) | [135] |
Genotoxicity | ↓ DNA damage (0.5, 50, 500 ng/g) | ||||||
Crustacean | Carcinus maenas | 0.1, 5, 10, 50 | 28 d | Hemolymph | ↓ LMS (exposure concentrations > 5 μg/L), LOEC: 5 μg/L | [136] | |
0.1, 5, 10, 50 | 28 d | Hepatopancreas | Oxidative stress and detoxification | ↑ DBF (5, 10 and 50 μg/L), GST (5, 10 and 50 μg/L), GPx (5, 10 and 50 μg/L) and LPO (all exposure concentrations) | [137] | ||
Genotoxicity | ↑ DNA damage (50 μg/L) | ||||||
Gills | Oxidative stress and detoxification | ↑ EROD (50 μg/L), DBF (5,10 and 50 μg/L), GST (5, 10 and 50 μg/L), GPx (10 and 50 μg/L) and LPO (all exposure concentrations) | |||||
Genotoxicity | ↑ DNA damage (5, 10 and 50 μg/L) | ||||||
Muscle | Oxidative stress and detoxification | ↑ DBF (10 μg/L), GST (10 and 50 μg/L), GPx (5, 10 and 50 μg/L) and LPO (0.1, 5 μg/L) | |||||
Genotoxicity | ↑ DNA damage (0.1, 5 and 50 μg/L) | ||||||
Gonads | Oxidative stress and detoxification | ↑ GST (5, 10 and 50 μg/L), GPx (0.1, 5, 10 and 50 μg/L), LPO (0.1, 5 and 50 μg/L) | |||||
Crustacean | Tisbe battagliai | 0-120,000 | 2 d | Nauplii | Mortality | LC50: 49,700 μg/L (2 d) | [119] |
Echinoderm | Lytechinus variegatus | 1.5, 15, 150.8, 1,508 ng/g dry weight* | 2 d | Larvae | Embryotoxicity | ↓ embrio-larval development (exposure concentrations ≥ 15 ng/g dw) | [138] |
Echinoderm | Parocentrotus lividus | 0.01, 0.1, 1, 5, 10 | 2 d | Larvae | Embryotoxicity | ↓ larval development (0.01, 0.1, 5, 10 μg/L), EC50: 0.01 μg/L (2 d), LOEC: 0.01 μg/L | [139] |
Echinoderm | Parocentrotus lividus | 0.1, 1, 10, 100,000, 500,000, 1,000,000, 100,000,000 | 60 min | Gametes | Reproduction | ↓ % fertilization (10,000,000μg L-1), EC50: 2,065,000 μg/L (60 min) | [139] |
Echinoderm | Psammechinus miliaris | 0.01, 0.1, 1, 10, 100, 1,000 | 120 min | Reproduction | ↓ sperm motility (exposure concentrations ≥ 1 μg·L-1 afterfrom 20 min) and swimming speed exposure concentrations ≥ 1 μg·L-1 after (≥ 1 from 20 min), EC50 (60 min): 845.98 μg/L ↓ % fertilization, EC50 (60 min): 437.03-4.56 × 106 μg/L | [122] | |
Mollusc | Crassostrea gigas | 1, 100 | 7 d | Gills | Oxidative stress and detoxification | ↑ CAT (1μg/L, 4 d), and GST (100 μg/L, 4 d) ↓ GR (1 and 100 μg/L, 4 and 7 d) | [100] |
Gene transcription | ↑ CYP2AU2 (1 μg/L, 7 d), CYP356A1 (1μg/L, 7 d), CYP3071A1 (1 μg/L, 7 d), GST-ω (100 μg/L at 1 d and 1 μg/L at 7 d), GST-π (100 μg/L at 4 d and 1 μg/L at 7 d), COX-like (100 μg/L, 7 d), FABP-like (1μg/L, 1 and 7 d) | ||||||
Mollusc | Mytella charruana | 0.02, 0.15, 1.51 1.5, 15, 150.8, 1,508 ng/g dry weight* | 24 h 2 d | Hemolymph | Immunocytotoxicity | ↓ LMS (0.15150.8 and 1.51508 ng/g dry weight) | [138] |
Mollusc | Mytilus galloprovincialis | 0.01, 0.1, 1, 10, 100, 1,000 | 2 d | Larvae | Embryotoxicity | alteration of embryo development (↑ shell malformations) (LOEC: 100 μg/L) | [127] |
Mollusc | Mytilus galloprovincialis | 0.250 | 15 d | Digestive gland | Oxidative stress | ↑ SOD (7d), GR (3 and 7 d), CAT (3 and 7 d) and LPO (3 and 7 d) | [140] |
Gonads | Vitellogenesis/endocrine disruption | Female: ↑ ALP (7 and 15 d) Male: ↑ ALP (3, 7 and 15 d) | |||||
0.250 | 15 d | Gills | Oxidative stress | ↓ GR (3, 7 and 15 d), CAT (3, 7 and 15 d), and GST (3 d) ↑ LPO (7 d) | [140] | ||
0.5 | 14 d | Digestive gland | Lysosomal responses | ↓ neutral lipids | [90] | ||
Hemocytes | Immunotoxicity | ↓ LMS | |||||
2.5 | 14, 30 and 60 d | Digestive gland | Lysosomal responses | ↑ neutral lipids (30 d) | [11] | ||
Hemocytes | Immunotoxicity | ↓ LMS (from 14 d) | |||||
Hemocytes | Immunotoxicity | ↓ granulocytes/hyalinocytes ratio (from 1,430 d) | |||||
↑ phagocytosis (from 30 d) | |||||||
Genotoxicity | ↑ MN frequency (14 and 30 d) | ||||||
25 | 14 d | Digestive gland | Oxidative stress and detoxification | ↓ GST | [26] | ||
Genotoxicity | ↑ DNA fragmentation and MN frequency | ||||||
Hemocytes | Immunotoxicity | ↓ LMS | |||||
250,000 | 15 d | Digestive gland | modulation of NF-kB pathway (up or down-expression of various genes) | [141] | |||
modulation of chitinase and GM2 activator protein genes | |||||||
Mollusc | Mytilus trossulus | 1, 100, 1,000, 5,000, 10,000 | 21 d (14 d of exposure+7 d of depuration) | Physiology | ↓ scope for growth (100 and 1,000 μg/L after 14 d of exposure) ↑ mortality (1,000, 5,000 and 10,000 μg/L) energy balance alteration (LOEC: 1,000 μg/L) | [97] | |
Mollusc | Perna perna | 1.5, 15, 150.8, 1,508 ng/g dry weight* | Larvae | Larvae | Embryotoxicity | ↓ embryo-larval development (≥ 15 ng/g dw) | [138] |
Mollusc | Ruditapes philippinarum | 0.1, 5,10, 50 | 14 d | Hemocytes | Immunotoxicity | ↓ LMS (all exposure concentrations) | [142] |
Digestive gland | Oxidative stress | ↑ GPx (0.1, 5μg·L-1), ↑ LPO (5, 10, 50 μg/L), GST (↑ 0.1, 10, 50 μg/L, ↓ 5 μg/L) | |||||
Genotoxicity | ↓ DNA damage (10, 50 μg/L) | ||||||
Neurotoxicity | ↑ AChE (5, 50 μg/L) | ||||||
0.1, 5, 10, 50 | 35 d | Hemocytes | Immunotoxicity | ↓ LMS (all exposure concentrations from 14 d) | [143] | ||
15 | 14 d | Digestive gland | Oxidative stress and detoxification | ↑ T-GPx (7 d), GR (1 d), GST (1 d), LPO (1 d) and, MT (1 d) ↓ SOD and GR (both after 14 d) | [133] | ||
Neurotoxicity | ↑ AChE (1 d) | ||||||
Gills | Oxidative stress and detoxification | ↑ SOD, GR, LPO, and MT (all after 7 d) ↓GST (3 h) | |||||
Neurotoxicity | ↓ AChE (7 d) | ||||||
100, 1,000 | 7 d | Digestive gland | Oxidative stress | ↓ SOD (: 100 and 1,000 μg/L after 5 and 7 d) | [144] | ||
Gills | Neurotoxicity | ↓ AChE (after 1d at 100 μg/L and after 7 d at all concentrations), ↑ AChE (1,000 μg/L after 3 d) | |||||
100, 1,000 | 7 d | Hemocytes | Immunotoxicity | ↓ total hemocytes count (1,000 μg/L) | [145] | ||
↓ pinocytosis (1,000 μg/L) | |||||||
↑ haemocyte proliferation | |||||||
Cytotoxicity | ↑ lactate dehydrogenase activity (1,000 μg/L) | ||||||
Polychaete | Arenicola marina | 0.01, 0.1, 1, 10, 100, 1,000 | 120 min | Reproduction | ↑ sperm swimming (exposure concentrations ≥ 10 μg/L after 30 min), EC50 not applicable | [122] | |
Polychaete | Hediste diversicolor | 0.05, 0.5, 5, 50, 500 ng/g* | 14 d | Whole body | Oxidative stress | ↑ LPO (5 ng/g) | [146] |
Neurotoxicity | ↑ AChE (500 ng/g) | ||||||
Cellular energy status | ↓ mitochondrial electron transport (5 ng/g) | [147] | |||||
Metabolism of monoamines | ↓ monoamine oxidase activity (0.5 ng/g) | ||||||
Inflammation | ↓ COX activity (all exposure concentrations) |
Acute toxicity data are very scarce for both NSAIDs. Survival under drug exposure was evaluated in the early life stages of the shrimp Siriella armata and the copepod Tisbe battagliai, showing LC50 values ranging from 0.01 to 15.8 mg/L for DCF, depending on the species and the exposure time, and an LC50 value of
Some studies have been conducted to evaluate the DCF and IBU effects on the larval development of sea urchins (Arbacia lixula, Lytechinus variegatus, and Paracentrotus lividus), marine bivalves (Mytilus galloprovincialis and Perna perna), and shrimp (Palaemon serratus), as reported in Tables 4 and 5. DCF negatively impacts the embryo development of all studied organisms, showing different sensitivity levels between species. Mytilus galloprovincialis showed increased shell malformation and reduced larval development at the lowest exposure concentration, closest to environmentally relevant concentrations (LOEC: 0.01 μg/L, 48 h), followed by Parocentrotus lividus (LOEC: 12.5 μg/L, 48 h), Palaemon serratus (LOEC: 900 μg/L, 50 days), Arbacia lixula (LOEC: 50,000 μg/L, 3 h), and finally Perna perna, which exhibited growth alterations at exposure concentrations 107 times higher compared to Mytilus galloprovincialis (LOEC: 100,000 μg/L, 48 h) [Table 4][75,117,121,123,126,127]. IBU was also shown to induce embryotoxicity in different species. It was classified as highly toxic for embryo-larval development by Pusceddu[138] and Aguirre- Martínez[139], who studied its effects on sea urchins and mussels. Species-specific differences were also observed in invertebrates exposed to IBU. Sea urchins (Parocentrotus lividus) showed decreased embryo development at lower exposure concentrations compared to Mytilus galloprovincialis (LOEC: 0.01 and 100 μg/L, respectively)[127,139]. On the other hand, the sea urchin Lytechinus variegatus and the mussel Perna perna exposed to IBU-spiked sediments showed similar inhibition of larval development from an exposure concentration of 15 ng/g dw[138]. The different responses observed in marine invertebrates exposed to DCF and IBU can be attributed to differences in species-specific sensitivity and distinct mechanisms of action and bioaccumulation for each compound[149].
Studies on adult organisms revealed that DCF and IBU affect reproduction and physiology. Mohd Zanuri et al. studied the effects of exposure to concentration gradients of DCF and IBU (0.01-1,000 μg/L, 120 min) on the reproductive success of the polychaete Arenicola marina, the sea star Asteria rubens, and the sea urchin Psammechinus miliaris, as reported in Tables 4 and 5[122]. Under DCF exposure, a common effect on the three species was the reduction of swimming speed and sperm motility at low exposure concentrations (0.1 and 1 μg/L), with a faster response observed in Asterias rubens and Psammechinus miliaris
DCF and IBU also alter physiological parameters in Mytilus trossulus[97]. The exposure negatively affected feeding and respiration rates (LOEC: 100 μg/L for both IBU and DCF), as well as the byssus strength and thread abundance (LOECDCF: 10,000 μg/L), with significant consequences on organism survival.
Adverse effects of DCF and IBU on the physiology, larval development, fertility, and reproductive success of invertebrates can lead to significant ecological impacts on populations and ecosystems. These impacts may include reduced survival, decline of populations, and effects on higher trophic levels. Furthermore, species like Ruditapes philippinarum and Mytilus galloprovincialis represent important economic resources in many coastal areas worldwide. Therefore, negative impacts at the population level could also have economic consequences.
Most of the studies found in our review focus on DCF and IBU responses at the sub-organism level, including tissue alterations, immune, geno-, and neurotoxicity, as well as modulation of the activity of various metabolic and protective enzymes and the expression of different genes. These endpoints are particularly relevant because most offer greater sensitivity than growth and reproduction effects, making them useful in determining the contribution of pollutants toward sub-lethal toxicity in real scenarios.
The only data available on tissue alterations in marine invertebrates are for Mytilus trossulus exposed to DFC, which show that this compound is able to induce damage to the mantle, gills, gonads, and digestive gland (e.g., inflammation, necrosis, deformations, etc.) at a relatively low concentration of 4 μg/L and an exposure time of 12 days, with possible consequences on respiratory, digestive, and reproductive systems[131,132].
Immuno- and genotoxic effects and antioxidant and detoxification responses associated with DCF and IBU exposure are the most investigated aspects in marine invertebrates, particularly in mollusk species.
The immunotoxicity studies in marine invertebrates are focused on the adverse effects of drugs on hemocytes, cells that play a key role in internal defense. The most studied marker is lysosomal membrane stability (LMS). Destabilization of the lysosomal membrane may result in activation and liberation of hydrolytic enzymes to the cytosol with possible alteration of immunological functions, the integrity of cells, and higher levels of biological organization (e.g., embryogenesis and larval development)[15,151]. The minimum effective concentrations on LMS were 0.02 μg/L for DCF (Perna perna, 24 h) and 0.1 μg/L for IBU (Ruditapes philippinarum, 14 days), indicating that immunotoxic effects can be produced in coastal and estuarine environments on resident invertebrates in the short and medium term[80,142]. Studies on immunotoxicity of DCF and IBU were conducted on various organisms, exposing them to different concentrations and durations. The results revealed that: (i) both DCF and IBU can modulate immunological parameters in marine bivalves (Mytilus galloprovincialis, Perna perna, Mytella charruana, and Ruditapes philippinarum) and crabs (Carcinus maenas); (ii) the alteration of immunotoxic parameters (LMS, granulocytes/hyalinocytes ratio, phagocytosis capacity, neutral lipid level, capability to perform pynocitosis, hemocytes proliferation, and lactate dehydrogenase activity) is species-, dose-, and time-dependent; (iii) some effects persist for an extended period up to 30 and 60 days, as reported in Tables 4 and 5[11,26,75,90,130,136,142,143].
In humans, NSAIDs act by inhibiting the cyclooxygenase enzymes COX-1 and/or COX-2, thereby blocking the formation of prostaglandin. The mechanism of NSAIDs action is presumed to be similar also in marine bivalves[152]. The modulation of COX activity and prostaglandin production was observed in Mytilus galloprovincialis (1 and 100 μg/L, 3 days) and Perna perna (LOEC: 0.2 μg/L for digestive gland and 0.02 μg/L for gills, 4 days) exposed to DCF, as reported in Table 4[75,96,140]. A possible effect of IBU on prostaglandin and COX was suggested by Milan et al. in Ruditapes philippinarum[144]. The decrease of COX activity was also observed by Maranho et al. in the polychaete Hediste diversicolor exposed to IBU spiked sediments (LOEC: 0.05 ng/g, 14 days exposure), as reported in Table 5[147]. Even though the role of prostaglandins in invertebrates has not yet been fully elucidated, they apparently participate in numerous biological functions, including development and reproduction, homeostatic cellular balance, ion transport, oogenesis, spermatogenesis, and immune defense. The participation in the noted biological functions could be disrupted under exposure to DCF and IBU[120,130,149,153]. Gonzalez-Rey and Bebianno[140] hypothesized a possible increase in H2O2 production as a consequence of a decrease in COX activity and accumulation of arachidonic acid that leads to the alteration of the oxidative state of cells. It is well recognized that NSAIDs can promote the formation of oxygen-reactive species (ROS) and, consequently, the induction of oxidative, cytotoxic, and genotoxic damages[154].
The presence of ROS in cells implies the activation of antioxidant defense, which involves a group of enzymes that work to inactivate ROS and are widely used as biomarkers of oxidative stress. These enzymes include catalase (CAT), superoxide dismutase (SOD), glutathione reductase (GR), and peroxidase (GPx), as well as reduced (GSH) and oxidized (GSSG) glutathione and ethoxyresorufin-O-deethylase (EROD). Glutathione S-transferase (GST) is also included in this group since it reduces the ROS lipid hydroperoxides to alcohol and H2O and participates in detoxification processes by conjugation with xenobiotic[154]. Finally, the measure of lipid peroxidation (LPO) is used as a biomarker of cellular oxidative damage. The alteration of enzyme activities and LPO levels after DCF exposure was observed in the mussels Mytilus edulis, Mytilus galloprovincialis, Mytilus trossulus, Perna perna, the clams Ruditapes philippinarum and Ruditapes decussatus, the crab Carcinus maenas, and the ragworm Hediste diversicolor, as reported in Table 4. In Table 5, we can observe that exposure to IBU also led to changes in antioxidant enzyme activity and lipid peroxidation (LPO) levels in various bivalve mollusk species (Mytilus galloprovincialis, Ruditapes philippinarum, and Crassostrea gigas), as well as in the amphipod Amphelisca brevicornis and the worm Hediste diversicolor. When the defense system is functioning correctly, we can observe the activation of antioxidant and detoxification enzyme, leading to a consequent reduction of LPO levels (or early LPO induction followed by reduction). This was observed particularly in the digestive gland of various Mytilus species exposed to different DCF concentrations (from 0.250 to 1,000 μg/L) and in the gills of Ruditapes philippinarum (15 μg/L), as reported in Table 4. These observations imply that the antioxidant defenses effectively counteract the toxic effects induced by DCF. When the antioxidant defenses become overwhelmed, cellular oxidative stress is established, which can lead to the inactivation of enzymes (decreased activity) and cellular damage (indicated by increased LPO levels)[155]. A reduction of enzyme activity was observed particularly in the gills of organisms exposed to DCF (Mytilus trossulus and Perna perna). However, in these organisms, the decrease in enzyme activity is not followed by oxidative damage. A beneficial action of DCF cannot be excluded, as its anti-inflammatory properties could counteract the inflammation produced in cells by the increase in reactive oxygen species (ROS)[156,157]. The most frequent responses observed in IBU-exposed organisms, regardless of species, tissue, time, and concentration of exposure, were the increase of both enzyme activity and LPO levels. As previously indicated, the persistence of high LPO levels may be a sign of the failure of antioxidant defenses to detoxify the excess ROS production and the production of significant oxidative damage that is usually accompanied by other effects such as DNA damage or protein degradation and cell death. Indeed, DCF and IBU showed the ability to induce genotoxic effects in various invertebrate species, as reported in Tables 4 and 5. An increase in DNA fragmentation was observed in hemocytes of Mytilus galloprovincialis exposed in vitro to low DCF concentration (0.005 μg/L). However, in vivo exposure resulted in a more pronounced effect of concentration, depending on species and tissue, with 2.5 μg/L in hemocytes of Mytilus galloprovincialis,
Another biomarker affected by the presence of DCF and IBU in the aquatic medium is acetylcholinesterase (AChE), which is commonly studied as a marker of neurotoxic effects. The most common effect observed in the studies reported in this review is the enhancement of its activity, which can be associated with cell apoptosis, where AChE is released from cells after membrane disruption. This was reported in Mytilus galloprovincialis, Perna perna, and Ruditapes philippinarum after DCF exposure at concentrations of 0.250, 2, and 15 μg DCF/L, respectively, as well as after IBU exposure in Ruditapes philippinarum in a concentration range of 10-1,000 μg IBU/L and Hediste diversicolor at a concentration of 500 ng IBU/g, as reported in Tables 4 and 5. The alterations in the structure and functions of the nervous system can have important consequences at different levels of biological organization, including cell development, inflammation, apoptosis, sensory alteration, neuromuscular dysfunctions, paralysis, and death of organisms. However, the neurotoxic effects of DCF and IBU on marine invertebrates, as well as the role of AChE in this group of organisms, are still poorly understood.
Finally, few studies focused on gene expression, protein, and metabolite modulation. Serrano et al. observed increased transcription in genes encoding antioxidant and auxiliary enzymes (GST, COX, CAT, heat shock protein, and cytochrome P450) in the oyster Crassostrea gigas exposed to IBU (1 and 100 μg/L) for 7 days[100]. IBU exposure at a concentration of 250,000 μg/L for 15 days also induced the modulation of chitinase and GM2 activator genes, which are supposed to have a role in general stress responses, as well as many genes related to the NF-kB pathway, a group of transcription factors involved in cellular responses to stress and the regulation of immune response and inflammation in Mytilus galloprovincialis[141]. Proteomic analyses were used to evaluate the effect of DCF on Mytilus edulis, revealing possible oxidation and changes in the abundance of proteins belonging to the family of caspases and heat shock proteins, well-known for their involvement in responses to stress conditions[125]. Additionally, changes were observed in proteins GADPH and BRAFLADRAFT-282392[124]. To date, Bonnefille and coauthors[129] are the only ones who have tested the potentially toxic effects of DCF on the metabolic pathways of the Mediterranean mussel (Mytilus galloprovincialis). They observed that this compound modulates the metabolism of tyrosine, tryptophan, and prostaglandins at a concentration of 100 μg/L for 7 days. They related these alterations to the potential impairment of biological functions such as byssus formation, osmoregulation, and reproduction. Results from omic analyses are very useful in understanding the mode of action of drugs and the biological and metabolic processes affected by exposure.
The review of available data on DCF and IBU effects on marine invertebrates, particularly at concentrations close to the ranges detected in coastal/estuarine areas and open seawaters, reveals a wide range of responses that depend on factors such as the target organism and tissue, exposure time, and concentration. This produces variable patterns that are sometimes difficult to interpret. In addition, gaps in our knowledge regarding both DCF and IBU mechanisms of action and the biology of marine invertebrates (including biochemical and molecular mechanisms and metabolic pathways) are evident.
RISK ASSESSMENT
The environmental risk assessment of a substance involves different steps to determine whether its occurrence at a certain level can negatively affect the environment. These steps include (i) identifying substances capable of producing adverse effects (hazard identification); (ii) establishing the relationship between dose-response assessment; and (iii) determining the exposure conditions. It is essential to know the exposure concentrations and routes to identify the potential risk.
The Technical Guidance Document on Risk Assessment of the European Commission (EU TGD) describes the evaluation procedure[158]. In summary, the procedure is based on comparing the predicted environmental concentration (PEC) or measured environmental concentration (MEC) with the concentration that does not represent any hazard for exposed organisms (predicted no effect concentration, PNEC). The ratio between these parameters allows to establish a risk quotient (RQ), as given by:
The PEC or MEC can be modified depending on the site, season, and physicochemical characteristics of aquatic ecosystems. In summary, these changes produce variations in the PEC or MEC and, consequently, in the RQ.
The PNEC calculation is carried out by using single-test toxicity data. In this laboratory assay, parameters such as EC50, LC50 or NOEC are determined using an assessment factor (AF), as given by:
If the RQ value exceeds 1, it implies a potential risk. On the contrary, there is no apparent risk if RQ is less than 1.
The critical point is to establish the assessment factor (AF). Different criteria are employed depending on the nature of the toxicity data. Thus, the AF lies in the range of 10-1,000 and its application accounts for the degree of uncertainty when extrapolating from lab toxicity test data for a limited number of species to a “real environment”. However, the selection of the AF is very inconsistent among studies, showing marked differences even when a similar toxicity database is used[159]. Durán and Beiras[160] recommended a test battery of five taxonomic groups, including algae, mollusks, crustaceans, echinoderms, and chordates, using toxicity thresholds (LOEC and NOEC) rather than EC50 values. They also emphasized prioritizing information originating from sublethal endpoints and sensitive early life stages. Although the most generalized protocol to establish the risk assessment is the TGD of the EU[148]. Other methods, such as the OECD[161], propose similar AF under similar database availability. Hansen[162] proposed using a bioanalytical system as an additional tool to conventional bioassays.
Table 6 shows the RQs collected from several scientific publications for DCF and IBU. The quotients were calculated for specific areas with the highest measured concentration in marine waters from sampled areas (MEC or MMC, except for two studies that used PEC) to represent the “worst case scenario”, following the TGD 2003 of the EU[158]. Data for the occurrence of these compounds were measured in seawater ecosystems. However, all PNEC values from the three trophic levels (algae, crustacean, and fish) used for RQ calculations are from freshwater systems. The lack of data on toxicity for marine species has supported the use of freshwater data.
Summary of RQs calculated for DCF and IBU in coastal seawater and sediment
Drug | Matrix (sampling site) | Target organisms for PNEC data | RQ | AF | Drug concentration used for RQ calculation | Risk classification | Refs |
DCF | Seawater (Baltic Sea, Bay of Puck) | Vibrio fisheri, Pseudokirchneriella subcapitata, Ceridaphnia dubia, Salmo trutta | 3.51 | 100-1,000 | MEC (196 ng/L) | High risk | [40] |
Seawater (Baltic Sea, Germany) | Synechococcus leopoliensis | 0.0048 | 10,000 | MEC (4.3 ng/L) | Insignificant risk | [163] | |
Seawater (China Sea, Taiwan) | Pseudokirchneriella subcapitata, Daphnia magna, Danio rerio | 0.004 | NR | MMC (53.6 ng/L) | Insignificant risk | [64] | |
0.165 | NR | PEC (2,230 ng/L) | Moderate risk | ||||
Seawater (Aegean Sea, Greece) | Pseudokirchneriella subcapitata, Daphnia magna, Danio rerio | 0 | 1,000 | MMC (16.3 ng/L) | Insignificant risk | [60] | |
Seawater (Atlantic Ocean, Bay of Cadiz) | Synechococcus leopoliensis, Daphnia magna, Pimephales promelas | < 0.01 | 1,000 | MEC (24.3 ng/L) | Insignificant risk | [55] | |
Seawater (Atlantic Ocean, Portugal) | Algae, crustacean, and fish (species not reported) | 0.0001-10 | 1,000 | MEC (1.14-241) | Insignificant-high risk | [49] | |
Seawater (Mediterranean Sea, Tunisia) | Pseudokirchneriella subcapitata, Daphnia magna,Pimephales promelas | < 0.01 | 1,000 | MEC (23 ng/L) | Insignificant risk | [57] | |
Seawater (Sea of Marmara, Turkey) | Pseudokirchneriella subcapitata , Daphnia magna, Salmo trutta | 0.31-26 | 10 | MEC (1,120 ng/L) | Moderate-high Risk | [62] | |
Seawater (Ionian Sea, Italy) | Synechococcus leopoliensis, Daphnia magna, Danio rerio | < 0.01 | 1,000 | MEC (441 ng/L) | Insignificant risk | [59] | |
Sediment (Ionian Sea, Italy) | < 0.01* | MEC (1.1 ng/g dw) | Insignificant risk | ||||
IBU | Seawater (China Sea, Taiwan) | Vibrio fisheri, Daphnia magna | 0.006 | NR | MMC (57.1 ng/L) | Insignificant risk | [64] |
2.165 | NR | PEC (19,700 ng/L) | High risk | ||||
Seawater (Atlantic Ocean, Bay of Cadiz) | Desmodesmus subspicatus, Daphnia magna, Pimephales promelas | 0.01-0.3 | 1,000 | MEC (1,219.7 ng/L) | Insignificant-moderate risk | [55] | |
Seawater (Atlantic Ocean, Portugal) | Algae, crustacean, and fish (species not reported) | < 1 | 1,000 | MEC (1.25-222) | Insignificant-high risk | [49] | |
Seawater (Sea of Marmara, Turkey) | Pseudokirchneriella subcapitata , Daphnia magna, Pimephales promelas | 0.05-0.43 | 1,000 | MEC (2,130 ng/L) | Low-moderate risk | [62] | |
Seawater (China Sea, Xiamen Bay) | NR | 0.00143 | NR | MEC (9.4 ng/L) | Low risk | [81] |
The RQs in coastal seawater generally show insignificant to moderate risk for both NSAIDs, as shown in Table 6. Most of these quotients are calculated for relatively low environmental concentrations
Table 7 compiles the DCF and IBU toxicity thresholds for marine species from different taxonomic groups. We utilized these thresholds to calculate PNEC and corresponding RQ values for the risk assessment of coastal and estuarine environments based on data reported in this review.
Ecotoxicity data for DCF and IBU towards selected marine and freshwater species representing different trophic levels
Drug | Taxon | Species | Endpoint | Toxicity threshold | mg/L | Refs |
DCF | Algae | Dunaliella tertiolecta | Population growth (96 h) | EC50/3 | 61.9 | [164] |
Skeletonema costatum | Population growth (72 h) | EC50/3 | 1.6 | [120] | ||
Isochrysis galbana | Population growth (72 h) | NOEC | > 5 | [159] | ||
Mollusc | Mytilus trossulus | Byssus strength (7 d) | LOEC | 10 | [97] | |
Perna perna | Larval development | NOEC | 10 | [75] | ||
Crustacean | Artemia salina | EC50/3 | 33.3 | [165] | ||
Siriella armata | Neonate mortality (48 h) | EC50/3 | 3.5 | [118] | ||
Tisbe battagliai | Neonate mortality | EC50/3 | 5.3 | [120] | ||
Neonate mortality (48 h) | EC50/3 | 3.2 | [119] | |||
Palaemon serratus | Larval growth (50 d) | LOEC | 0.9 | [117] | ||
Echinoderm | Parocentrotus lividus | Larval length (48 h) | LOEC | 0.0125 | [123] | |
Early larval growth (48 h) | LOEC | 0.0125 | [123] | |||
Fish | Pimephales promelas | EC50/3 | 177.3 | [166] | ||
Oryzias latipes | Adult mortality (96 h) | EC50/3 | 3.36 | http://cfpub.epa.gov/ecotox | ||
Danio rerio | Larval abnormalities (80 h) | LOEC | 12.5 | [159] | ||
IBU | Algae | Isochrysis galbana | Population growth (72 h) | EC10 | 22.6 | [159] |
Skeletonema costatum | Population growth (96 h) | EC50/3 | 2.7 | [159] | ||
Mollusc | Mytilus galloprovincialis | Larval morphology (48 h) | LOEC | 0.1 | [127] | |
Mytilus trossulus | SFG reduction (14 d) | LOEC | 0.1 | [98] | ||
Crustacean | Tisbe battagliai | Neonate mortality (48 h) | EC50/3 | 16.6 | [119] | |
Fish | Pimephales promelas | EC50/3 | 1.6 | [166] | ||
Embryo/larval development (33 d) | NOEC | 3 | [159] | |||
Oryzias latipes | Larval mortality (96 h) | EC50/3 | > 33.3 | [159] | ||
Leomis macrochinus | Survival (96 h) | EC50/3 | 57.7 | [159] |
As recommended by Beiras[159] and Durán and Beiras[160], we used five taxonomic levels (algae, crustaceans, and fish plus two additional marine taxonomic groups) to enhance the sensitivity and protective values of RQs. Since data for marine fish are unavailable, we combined data sets of marine and freshwater species to derive environmental quality standards for transitional and coastal waters, following the EU TGD[158]. Threshold values reported in the bibliography for freshwater fish were used in these cases. Additionally, sublethal endpoints (NOEC and LOEC) were used for calculation. In cases where endpoints were unavailable, EC50/3 was used, following the approach suggested by Durán and Beiras[160]. According to the Manual on the Methodological Framework to Derive Environmental Quality Standards (EQS Manual)[167], we used an AF of 10 for PNEC calculation for both DCF and IBU. Regarding MEC, we used data from Table 1. The reported data cover a wide range of concentrations, spanning from a few ng/L to a few μg/L. To cover various contamination levels, we selected a range of values representative of low, medium, and high contamination levels. The RQ values and their corresponding risk classifications are reported in Table 8. We established a safe level for DCF below 100 ng/L. Concentrations of DCF higher than 102 μg/L and 1,500 μg/L present moderate and high risks, respectively, for organisms living in coastal areas, as indicated in Table 8. On the other hand, IBU presents a low to moderate risk at higher environmental concentrations than DCF (ranging from 109 μg/L to 6,297 μg/L, as shown in Table 8), suggesting lower IBU toxicity for organisms living in coastal and estuarine areas.
RQ calculation and risk classification for coastal and estuarine environments
Drug | MEC (ng/L) | RQ | Risk classification | Reference for environmental concentrations |
DCF | 0.02 | 0.000016 | Insignificant | [58] |
102 | 0.0816 | Low | [39] | |
205 | 0.164 | Moderate | [41] | |
441 | 0.3528 | Moderate | [59] | |
1,120 | 0.896 | Moderate | [63] | |
1,500 | 1.2 | High | [47] | |
10,221.1 | 81.77 | High | [4] | |
15,087 | 12.07 | High | [45] | |
IBU | 0.049 | 0.0000049 | Insignificant | [79] |
109 | 0.0109 | Low | [37] | |
222 | 0.0222 | Low | [49] | |
508 | 0.0508 | Low | [4] | |
1,219.7 | 0.122 | Moderate | [55] | |
1,600 | 0.16 | Moderate | [47] | |
2,550 | 0.255 | Moderate | [59] | |
6,297 | 0.6297 | Moderate | [41] | |
10,053 | 1.005 | High | [45] |
The highest risk for both NSAIDs is detected in areas previously identified as subject to strong anthropogenic pressure, such as high pharmaceutical consumption, the presence of industrial activities and hospitals, the discharge of effluents from WWTPs, and low removal efficiency from WWTPs. In addition, a higher risk is also observed in the Red Sea, the Humber estuary, Cadiz Bay, and the Antarctic Peninsula. The risk classification from our study is consistent with the results from the literature data reported in Table 6, particularly at the highest MEC (e.g., moderate risk for IBU at Cadiz Bay and the Sea of Marmara for DCF). However, differences at the lowest MEC can be related to the AF and endpoint considered for calculation.
The risk for the sampling sites reported in this review ranged between low and high. Specifically, 80% of sites showed low risk for DCF and 83% for IBU, 10% of sites showed moderate risk for DCF and 15% for IBU, and 10% of sites showed high risk for DCF and 2% IBU.
Besides the single-toxicity test, another way to obtain information about safe levels for risk assessment is to use species sensitivity distribution (SSD). The basic premise is to assume that sensitive species can be described using a parametric distribution (e.g., logistic)[168]. This approach was employed by Trombini et al. for DCF and IBU using acute toxicity data (LC50 or EC50 values) from aquatic species, mainly freshwater species[23]. The hazard effect concentration (HEC5) calculated for DCF and IBU were 4.5 and 4.4 mg/L, respectively. Using AF of 5, we calculated the PNEC, which is close to 0.8 mg/L for both compounds. However, this value is higher than some chronic toxicity data for these compounds[168], which fall in the order of g/L, indicating that some precautions should be considered to derive safe levels for aquatic species as they may not be fully protective for the environment. Posthuma and coauthors[168] also highlighted the limitations of this approach.
Yanagihara et al. pointed out that the acute SSDs for saltwater species can be estimated using those of freshwater species[169]. However, the number of species considered will be a crucial factor in obtaining more results. The authors also mentioned that the establishment of relationships for chronic toxicity SSD data was not feasible due to the insufficient size of the database available for such an analysis.
Most pollutant-associated risk classifications are based on acute-type responses. However, as discussed in the previous section, at environmentally relevant concentrations, DCF and IBU trigger a wide range of responses at the sub-organism level that can have important consequences on the health status of organisms. These responses are not typically considered by classical environmental risk assessment models, highlighting the need for a more comprehensive approach to assessing the potential ecological impacts of these substances. Mezzelani et al. employed a multidisciplinary approach integrating the measurement of drug bioaccumulation with many biomarker responses to assess the perturbation of cellular districts and molecular pathways[26,90]. The observed variations were analyzed using a quantitative model and weighted criteria (Sediqualsoft), which generates a cellular hazard index known as the Hazard Quotient for biomarkers (HQBM)[170,171]. The HQBM takes into account the relevance and magnitude of the measured biomarkers. To calculate this index, each biomarker is assigned a specific “weight” based on its toxicological relevance, and a “threshold” is defined to establish the minimum percentage considered biologically relevant, as given by:
The exposure of Mytilus galloprovincialis to both NSAIDs showed slight to moderate hazard depending on the exposure concentration. Specifically, a slight hazard for both compounds after 14 days of exposure at
MIXTURE RISK ASSESSMENT
One of the main challenges of ecotoxicology is assessing the impact of pollutants in the real world, where the substances are not present in individual forms but as complex mixtures. The joint action of a species can be different from the sum of the individual effects, as the interaction between components can produce diverse effects. The main toxicological interactions include synergism, antagonism, potentiation, inhibition, and masking[172,173].
Models have been used to analyze the combined effect of species using data from individual compounds. The classical approach is based on concentration addition (CA) and independent action (IA). The CA model assumes that all mixtures of the component have a similar mode of action and act on the same biochemical pathways and target sites. The mixture toxicity is expressed as:
where n is the number of compounds, ECxmix is the effect of concentration of the mixture provoking an x% effect and ECxi is the concentration of component i provoking the same effect (x%) as the mixture when applied individually, and pi is the fraction of component i in the mixture.
The IA model assumes that the chemical model of action shows dissimilarity in the interaction with molecules and target sites. As a consequence, the relative effect of a compound in the mixture can be unaffected by the occurrence of other compounds. In this model, the global toxicity can be related only to specific compounds in the mixture. The equation applies for the mixture toxicity is expressed as:
where E(Cmix) is the effect of the total concentration in the mixture and E(Ci) is the effect generated by component i at the concentration Ci.
The CA model has been employed for the mixture of different chemical compounds[174-179]. Although the classical approaches have been employed widely due to their simplicity and usefulness for regulators, the results are subjected to some bias. Thus, the CA model overestimates the toxicity, and the IA model underestimates it[119]. Nonetheless, in the real world, the predictive power of both models will decrease due to the complexity of biological systems and the specificity of the pathways of chemical mixtures. Other alternative methods have been developed to overcome the limitations of CA and IA models. Among alternative methods, two-stage prediction (TSP), integrated fuzzy concentration addition-independent action model (INFCIM), toxic equivalency factors (TEF), mixture toxicity indices (MTI), median effect/combination index (CI-isobologram equation), and quantitative structure-activity relationship (QSAR) can be cited.
The risk associated with the mixtures can be assessed using the RQ. According to Backaus and Faust[180], the RQs of mixtures can be calculated assuming the individual ratios PEC/PNEC ratios as follows:
The risk can be calculated for a specific organism using data from different experiments or, in a more general way, using data from different trophic levels according to the following equation:
Information about the mixture toxicity of pharmaceuticals is very scarce. This is especially remarkable in the marine environment. However, the mixture toxicity for DCF and IBU was assessed in the marine copepod Tisbebattagliai by Trombini et al.[119]. The study was carried out on neonate nauplii (< 24 h old) exposed for 48 h to both pharmaceutical compounds, following the UK Environment Agency protocol[181]. The toxicity of both compounds expressed as LC50 has been reported to be 9.5 and 49.7 mg/L, respectively, indicating higher toxicity for DCF. According to the EU Directive 93/67/EEC[148], individual compounds can be identified as toxic and harmful. Nevertheless, the occurrence of the compounds in the environment is a mixture of components, and the estimation based on individual components can underestimate the risk. The species Tisbe battagliai showed a low risk of exposure to individual or mixture compounds[119]. However, the mixture risk was higher than individual compounds. This observation agrees with the CA model that assumes that compounds with similar modes of action increase the overall toxicity of the mixture since DCF and IBU act on the same biochemical pathways and target molecules. However, these authors questioned the accuracy of the classical models (CA and IA) in assessing the risk of the occurrence of these drugs in real scenarios at low concentrations.
Similar to the risk assessment of individual compounds, the models used for assessing the risk of contaminant mixtures are primarily based on acute toxicity data. However, there is a lack of studies focusing on the sublethal effects of mixtures, especially involving DCF and IBU, on marine invertebrates. In a study conducted by Gonzalez-Rey and coauthors[182], they investigated the effects of two mixtures, DCF and IBU (250 ng/L each), in combination with fluoxetine (75 ng/L) and copper (5 μg/L), on the mussel species Mytilus galloprovincialis. The researchers observed notable changes in biochemical responses and gene expression related to oxidative stress, neurotoxicity, and endocrine disruption. Importantly, these alterations differed from the effects observed in a single compound exposure. Biochemical alterations (SOD, CAT, LPO, MT, and AChE) were also observed in the clam Scrobicularia plana exposed to a mixture of IBU, ciprofloxacin, and flumequine during a 21-day period, as reported by Trombini[183]. Similarly, Ericson et al. reported a decrease in the scope for growth and in the ability to attach to the substrate in the mussel species Mytilus edulis when exposed to a combination of DCF, IBU, and propanolol at concentrations ranging from 1-10,000 μg/L[97]. Fabbri et al. conducted a study on the effects of a complex mixture, which included IBU and DCF at concentrations ranging from 0.01 to 1 μg/L, with a 48-hour exposure on the embryos of Mytilus galloprovincialis[184]. The researchers observed a retarded development and shell malformations in the exposed embryos. Additionally, Luna-Acosta et al. reported that a mixture of IBU and the herbicides diuron and isoproturon (at a concentration of 5 μg/L) induced early effects at both the biochemical level (resulting in a decrease of catecholase-type phenoloxidase activity in the plasma) and the cellular level (leading to a decrease of phagocytosis activity in hemolymph) in the oyster species Crassostrea gigas after a 6-hour exposure[185].
In toxicological studies, replicating a completely realistic scenario is challenging due to the complexity and variability of contaminant mixtures in the marine environment, which may include pharmaceuticals, metals, micro and nanoplastics, pesticides, and more. Additionally, the toxicity of these mixtures can be influenced by changes in physicochemical variables. Therefore, some authors have investigated the combined effects of DCF and changes in water pH, temperature, and salinity, which are alterations typically associated with climate change. Munari et al. showed that seawater acidification increases the sensitivity of the larvae of Ruditapes philippinarum to DCF, altering survival (increased mortality) and growth (increased morphological abnormalities)[153]. Similarly, González-Ortegón et al. reported an increased DCF toxicity in larvae of the marine shrimp Palaemon serratus when exposed to DCF in combination with changes in temperature and salinity[117]. However, other studies carried out with bivalve mollusks (Mytilus and Ruditapes species) did not show clear synergistic effects between DCF and changes in temperature, pH, or salinity[92,93,99,186].
NEW APPROACHES (AOPs)
The development of a new approach for assessing toxicity, which takes into account the underlying mechanisms of the toxicity process, has received great attention. This is because the classical approach relies on assessment factors that often lack ecological relevance and uncertainty. Additionally, there are economic and practical difficulties in applying it to the current chemical products in the market and the new products generated by the chemical industry. The World Health Organization (WHO), through the International Program on Chemical Safety (IPCS), is taking steps to develop a scientific foundation for chemical management that reduces the reliance on animal testing. They aim to generate risk identification tools by utilizing new technologies capable of providing high-throughput biological data at lower organizational levels (ecotoxicogenomic) while harnessing the increasing computational power capacity for data processing and prediction using Big Data and Artificial Intelligence (AI).
The Adverse Outcome Pathway (AOP) is a conceptual framework[187] that depicts the sequence of logical events or processes in a biological system. It considers understanding adverse effects and refining current risk assessment. The final objective is to develop predictive models for human and environmental toxicology.
The core principle of AOP is that exposure to chemical substances at a certain dose can trigger a molecular initiating event (MIE) (e.g., receptor binding), leading to a cascade of key events (KE), which can ultimately result in a pathological effect that is considered an adverse outcome. AOP represents the sequence of events from the exposure of an individual to a chemical, leading to an understanding of the adverse effect at the population level. The link between chemicals and MIE represents a “small jump” concerning toxicological endpoints. This approach establishes stronger connections to identify the mechanisms that underlie the toxic effect and can generate a more generalized and predictive model for aquatic species. AOPwiki (https://aopwiki.org) is a database whose objective is to be the repository of all AOPs developed as a part of the OECD AOP Development Effort, supported by the Extended Advisory Group on Molecular Screening and Toxicogenomics. Currently, there is no complete view of the MIEs and KEs and the concentration that triggers these events.
The use of omic approaches to assess the effect of pollutants on marine organisms has shown a high potential in understanding the response mechanisms without the need for any “a priori” hypothesis. Many pollutants reach the marine environment through the discharge of WWTPs, carrying a complex mixture of pollutants, posing a potential threat to the health of marine ecosystems. Environmental metabolomics has been shown to be an efficient tool for assessing the effect of multi-contamination in marine organisms[188]. It has been reported that this exposure led to alterations in a large number of metabolites, including those belonging to amino acid metabolism, neurohormones, purine and pyridine metabolism, as well as citric acid cycle intermediates and oxidative stress defenses[188]. These changes reflect the alteration of biological processes. Nevertheless, the use of a metabolomics approach to assess the exposure of marine organisms to NSAIDs is very scarce. Bonnefille et al. identified that the DCF effects on the Mediterranean mussel
The metabolomics approach has been shown to be useful in defining adverse outcome pathways for pharmaceuticals.
CONCLUSIONS
The risk assessment of individual compounds for aquatic species in the estuaries and marine environment involves analyzing the ratio between ecotoxicology information and the environmental concentrations. Currently, the presence of pharmaceutical compounds, specifically non-steroidal anti-inflammatory drugs (IBU and DCF), has increased in the marine environment. To evaluate the risk linked to these compounds, we have compiled data from the scientific literature, encompassing a diverse range of environmental concentrations and biological responses (such as bioaccumulation rates and effects of various levels of biological organization). These variations are contingent on location, environmental conditions, and the species under consideration. This suggests that the risk associated with the presence of these two drugs can vary depending on the species, site, and exposure concentrations being considered. Diclofenac showed a higher risk than ibuprofen for aquatic species. The risk for both compounds was generally low, encompassing approximately 80% of the reported sites, while a reduced number of sites showed high risk, ranging from 2% to 10%. Due to the concurrent presence of both compounds in the marine environment, with similar primary sources (e.g., wastewater), the risk of the mixture of DCF and IBU exhibited a higher risk compared to individual compounds. In addition to the conventional methods for risk assessment, alternative approaches have been considered, such as the use of adverse outcome pathways and toxicogenomic tools (including omics techniques such as transcriptomic, proteomic, and metabolomics analyses) to gain insights into the underlying mechanisms of toxicity. However, taking the research a step further will necessitate expanding the knowledge base on various species and employing tools such as Big Data and artificial intelligence. Additionally, the marine ecosystem is a dynamic environment influenced by numerous drivers and pressures (e.g., ocean acidification, heat waves, climate change, etc.) that can impact the fate, behavior, and physiological responses of organisms to these compounds. As a result, evaluating the risk of NSAIDs in the marine environment presents challenges, as it will require addressing the information gaps through further research and data collection.
DECLARATIONS
AcknowledgmentsWe would like to thank for Spanish Research Agency funding the project PID2019-110049RB-I00/AEI/10.13039/501100011033
Authors’ contributionsData curation, formal analysis and writing the manuscript: Blasco J, Trombini C
Availability of data and materialsNot applicable.
Financial support and sponsorshipNone.
Conflicts of interestAll authors declared that there are no conflicts of interest.
Ethical approval and consent to participateNot applicable.
Consent for publicationNot applicable.
Copyright© The Author(s) 2023.
Supplementary MaterialsREFERENCES
1. Tornero V, Hanke G. Potential chemical contaminants in the marine environment: An overview of main contaminant lists. JRC Technical Reports. Available from: https://mcc.jrc.ec.europa.eu/documents/201801085655.pdf. [Last accessed on 2 Aug 2023].
2. Vlachogianni T, Valavanidis A. Pharmaceuticals and personal care products as contaminants in the aquatic environment. A category of organic wastewater pollutants with special characteristics. Pharmakeftiki 2013;25:16-23. Available from: https://www.researchgate.net/publication/236229893.[Last accessed on 2 Aug 2023]
3. Liu JL, Wong MH. Pharmaceuticals and personal care products (PPCPs): a review on environmental contamination in China. Environ Int 2013;59:208-24.
4. Ali AM, Rønning HT, Alarif W, Kallenborn R, Al-Lihaibi SS. Occurrence of pharmaceuticals and personal care products in effluent-dominated Saudi Arabian coastal waters of the Red Sea. Chemosphere 2017;175:505-13.
5. Adeleye AS, Xue J, Zhao Y, et al. Abundance, fate, and effects of pharmaceuticals and personal care products in aquatic environments. J Hazard Mater 2022;424:127284.
6. Daughton CG, Ternes TA. Pharmaceuticals and personal care products in the environment: agents of subtle change? Environ Health Perspect 1999;107 Suppl 6:907-38.
7. Gros M, Petrović M, Barceló D. Wastewater treatment plants as a pathway for aquatic contamination by pharmaceuticals in the ebro river basin (northeast Spain). Environ Toxicol Chem 2007;26:1553-62.
8. Petrović M, Gonzalez S, Barceló D. Analysis and removal of emerging contaminants in wastewater and drinking water. TrAC Trends in Analytical Chemistry 2003;22:685-96.
9. Osorio V, Larrañaga A, Aceña J, Pérez S, Barceló D. Concentration and risk of pharmaceuticals in freshwater systems are related to the population density and the livestock units in Iberian Rivers. Sci Total Environ 2016;540:267-77.
10. Monteiro SC, Boxall ABA. Occurrence and fate of human pharmaceuticals in the environment. Rev Environ Contam Toxicol 2010;202:53-154.
11. Mezzelani M, Gorbi S, Regoli F. Pharmaceuticals in the aquatic environments: evidence of emerged threat and future challenges for marine organisms. Mar Environ Res 2018;140:41-60.
12. Fonseca TG, Morais MB, Rocha T, Abessa DMS, Aureliano M, Bebianno MJ. Ecotoxicological assessment of the anticancer drug cisplatin in the polychaete Nereis diversicolor. Sci Total Environ 2017;575:162-72.
13. Jelic A, Gros M, Ginebreda A, et al. Occurrence, partition and removal of pharmaceuticals in sewage water and sludge during wastewater treatment. Water Res 2011;45:1165-76.
14. Santos LHMLM, Araújo AN, Fachini A, Pena A, Delerue-Matos C, Montenegro MCBSM. Ecotoxicological aspects related to the presence of pharmaceuticals in the aquatic environment. J Hazardous Materials 2010;175:45-95.
15. Świacka K, Michnowska A, Maculewicz J, Caban M, Smolarz K. Toxic effects of NSAIDs in non-target species: a review from the perspective of the aquatic environment. Environ Pollut 2020;273:115891.
16. Zhao JL, Ying GG, Liu YS, et al. Occurrence and a screening-level risk assessment of human pharmaceuticals in the Pearl River system, South China. Environ Toxicol Chem 2010;29:1377-84.
17. Thomas KV, Hilton MJ. The occurrence of selected human pharmaceutical compounds in UK estuaries. Mar Pollut Bull 2004;49:436-44.
18. Beretta M, Britto V, Tavares TM, da Silva SMT, Pletsch AL. Occurrence of pharmaceutical and personal care products (PPCPs) in marine sediments in the Todos os Santos Bay and the north coast of Salvador, Bahia, Brazil. J Soils Sediments 2014;14:1278-86.
19. Hernández-Tenorio R, González-Juarez E, Guzmán-Mar JL, Hinojosa-reyes L, Hernández-Ramírez A. Review of occurrence of pharmaceuticals worldwide for estimating concentration ranges in aquatic environments at the end of the last decade. J Hazard Mater 2022;8:100172.
20. Commission Implementing Decision (EU) 2015/495 of 20 March 2015 establishing a watch list of substances for Union-wide monitoring in the field of water policy pursuant to Directive 2008/105/EC of the European Parliament and of the Council (notified under document C(2015) 1756) Text with EEA relevance. Available from: https://eur-lex.europa.eu/legal-content/EN/TXT/?uri=uriserv%3AOJ.L_.2015.078.01.0040.01.ENG. [Last accessed on 2 Aug 2023].
21. Commission Implementing Decision (EU) 2018/840 of 5 June 2018 establishing a watch list of substances for Union-wide monitoring in the field of water policy pursuant to Directive 2008/105/EC of the European Parliament and of the Council and repealing Commission Implementing Decision (EU) 2015/495 (notified under document C(2018) 3362). Available from: https://eur-lex.europa.eu/legal-content/EN/TXT/PDF/?uri=CELEX:32018D0840. [Last accessed on 2 Aug 2023].
22. Batucan NSP, Tremblay LA, Northcott GL, Matthaei CD. Medicating the environment? A critical review on the risks of carbamazepine, diclofenac and ibuprofen to aquatic organisms. Environ Adv 2022;7:100164.
23. Trombini C, Blasco J, Hampel M. Ibuprofen and diclofenac: effects on freshwater and marine aquatic organisms - Are they at risk? In: Gómez-Oliván LM, editor. Non-Steroidal Anti-Inflammatory Drugs in Water. The Handbook of Environmental Chemistry; 2020. p. 161-89.
24. Chaumot A, Ferrari B, Geffard O, Garric J. Ecotoxicology, aquatic invertebrates. Encyclopedia of toxicology 2014;2:284-8.
25. Parolini M. Toxicity of the Non-Steroidal Anti-Inflammatory Drugs (NSAIDs) acetylsalicylic acid, paracetamol, diclofenac, ibuprofen and naproxen towards freshwater invertebrates: a review. Sci Total Environ 2020;740:140043.
26. Mezzelani M, Gorbi S, Da Ros Z, et al. Ecotoxicological potential of non-steroidal anti-inflammatory drugs (NSAIDs) in marine organisms: bioavailability, biomarkers and natural occurrence in mytilus galloprovincialis. Mar Environ Res 2016;121:31-9.
27. Branchet P, Arpin-Pont L, Piram A, Boissery P, Wong-Wah-Chung P, Doumenq P. Pharmaceuticals in the marine environment: what are the present challenges in their monitoring? Sci Total Environ 2021;766:142644.
28. Świacka K, Maculewicz J, Kowalska D, Caban M, Smolarz K, Świeżak J. Presence of pharmaceuticals and their metabolites in wild-living aquatic organisms - Current state of knowledge. J Hazard Mat 2022;424:127350.
29. Larsson DG, de Pedro C, Paxeus N. Effluent from drug manufactures contains extremely high levels of pharmaceuticals. J Hazard Mater 2007;148:751-5.
30. Majumder A, Gupta AK, Ghosal PS, Varma M. A review on hospital wastewater treatment: a special emphasis on occurrence and removal of pharmaceutically active compounds, resistant microorganisms, and SARS-CoV-2. J Environ Chem Eng 2021;9:104812.
31. Hider-Mlynarz K, Cavalié P, Maison P. Trends in analgesic consumption in France over the last 10 years and comparison of patterns across Europe. Br J Clin Pharmacol 2018;84:1324-34.
32. Arpin-Pont L, Bueno MJM, Gomez E, Fenet H. Occurrence of PPCPs in the marine environment: a review. Environ Sci Pollut Res 2016;23:4978-91.
33. Desbiolles F, Malleret L, Tiliacos C, Wong-Wah-Chung P, Laffont-Schwob I. Occurrence and ecotoxicological assessment of pharmaceuticals: is there a risk for the Mediterranean aquatic environment? Sci Total Environ 2018;639:1334-48.
34. Ngubane NP, Naicker D, Ncube S, Chimuka L, Madikizela LM. Determination of naproxen, diclofenac and ibuprofen in Umgeni estuary and seawater: a case of northern Durban in KwaZulu-Natal Province of South Africa. Reg Stud Mar Sci 2019;29:100675.
35. Bonnefille B, Gomez E, Courant F, Escande A, Fenet H. Diclofenac in the marine environment: a review of its occurrence and effects. Mar Pollut Bull 2018;131:496-506.
36. Madikizela LM, Ncube S, Tutu H, et al. Pharmaceuticals and their metabolites in the marine environment: sources, analytical methods and occurrence. Trends Environ Anal Chem 2020;28:e00104.
37. Nödler K, Voutsa D, Licha T. Polar organic micropollutants in the coastal environment of different marine systems. Mar Pollut Bull 2014;85:50-9.
38. Borecka M, Siedlewicz G, Haliński ŁP, et al. Contamination of the southern Baltic Sea waters by the residues of selected pharmaceuticals: method development and field studies. Mar Pollut Bull 2015;94:62-71.
39. Caban M, Mioduszewska K, Lukaszewicz P, et al. A new silylating reagent - dimethyl(3,3,3-trifluoropropyl)silyldiethylamine - for the derivatisation of non-steroidal anti-inflammatory drugs prior to gas chromatography-mass spectrometry analysis. J Chromatogr A 2014;1346:107-16.
40. Szymczycha B, Borecka M, Białk-Bielińska A, Siedlewicz G, Pazdro K. Submarine groundwater discharge as a source of pharmaceutical and caffeine residues in coastal ecosystem: bay of Puck, southern Baltic Sea case study. Sci Total Environ 2020;713:136522.
41. Letsinger S, Kay P, Rodríguez-Mozaz S, Villagrassa M, Barceló D, Rotchell JM. Spatial and temporal occurrence of pharmaceuticals in UK estuaries. Sci Total Environ 2019;678:74-84.
42. Nebot C, Gibb SW, Boyd KG. Quantification of human pharmaceuticals in water samples by high performance liquid chromatography-tandem mass spectrometry. Anal Chim Acta 2007;598:87-94.
43. Vanryckeghem F, Huysman S, Van Langenhove H, Vanhaecke L, Demeestere K. Multi-residue quantification and screening of emerging organic micropollutants in the Belgian Part of the North Sea by use of Speedisk extraction and Q-Orbitrap HRMS. Marine Pollution Bulletin 2019;142:350-60.
44. Kallenborn R, Brorström-Lundén E, Reiersen LO, Wilson S. Pharmaceuticals and personal care products (PPCPs) in Arctic environments: indicator contaminants for assessing local and remote anthropogenic sources in a pristine ecosystem in change. Environ Sci Pollut Res Int 2018;25:33001-13.
45. González-Alonso S, Merino LM, Esteban S, et al. Occurrence of pharmaceutical, recreational and psychotropic drug residues in surface water on the northern Antarctic Peninsula region. Environ Pollut 2017;229:241-54.
46. McEneff G, Barron L, Kelleher B, Paull B, Quinn B. A year-long study of the spatial occurrence and relative distribution of pharmaceutical residues in sewage effluent, receiving marine waters and marine bivalves. Sci Total Environ 2014;476-7:317-26.
47. Togola A, Budzinski H. Multi-residue analysis of pharmaceutical compounds in aqueous samples. J Chromatogr A 2008;1177:150-8.
48. Gonzalez-Rey M, Tapie N, Le Menach K, Dévier MH, Budzinski H, Bebianno MJ. Occurrence of pharmaceutical compounds and pesticides in aquatic systems. Mar Pollut Bull 2015;96:384-400.
49. Lolić A, Paíga P, Santos LH, Ramos S, Correia M, Delerue-Matos C. Assessment of non-steroidal anti-inflammatory and analgesic pharmaceuticals in seawaters of North of Portugal: occurrence and environmental risk. Sci Total Environ 2015;508:240-50.
50. Paíga P, Lolić A, Hellebuyck F, Santos LH, Correia M, Delerue-Matos C. Development of a SPE-UHPLC-MS/MS methodology for the determination of non-steroidal anti-inflammatory and analgesic pharmaceuticals in seawater. J Pharm Biomed Anal 2015;106:61-70.
51. Baena-Nogueras RM, Pintado-Herrera MG, González-Mazo E, Lara-Martín PA. Determination of pharmaceuticals in coastal systems using solid phase extraction (SPE) followed by ultra performance liquid chromatography - tandem mass spectrometry (UPLC-MS/MS). Curr Anal Chem 2016;12:183-201.
52. Maranho LA, Garrido-Pérez MC, Baena-Nogueras RM, et al. Are WWTPs effluents responsible for acute toxicity? Seasonal variations of sediment quality at the Bay of Cádiz (SW, Spain). Ecotoxicology 2015;24:368-80.
53. Afonso-Olivares C, Torres-Padrón ME, Sosa-Ferrera Z, Santana-Rodríguez JJ. Assessment of the presence of pharmaceutical compounds in seawater samples from coastal area of Gran Canaria Island (Spain). Antibiotics 2013;2:274-87.
54. Pintado-Herrera MG, González-Mazo E, Lara-Martín PA. Environmentally friendly analysis of emerging contaminants by pressurized hot water extraction-stir bar sorptive extraction-derivatization and gas chromatography-mass spectrometry. Anal Bioanal Chem 2013;405:401-11.
55. Biel-Maeso M, Baena-Nogueras RM, Corada-Fernández C, Lara-Martín PA. Occurrence, distribution and environmental risk of pharmaceutically active compounds (PhACs) in coastal and ocean waters from the Gulf of Cadiz (SW Spain). Sci Total Environ 2018;612:649-59.
56. Gros M, Rodríguez-Mozaz S, Barceló D. Fast and comprehensive multi-residue analysis of a broad range of human and veterinary pharmaceuticals and some of their metabolites in surface and treated waters by ultra-high-performance liquid chromatography coupled to quadrupole-linear ion trap tandem mass spectrometry. J Chromatogr A 2012;1248:104-21.
57. Afsa S, Hamden K, Lara Martin PA, Mansour HB. Occurrence of 40 pharmaceutically active compounds in hospital and urban wastewaters and their contribution to Mahdia coastal seawater contamination. Environ Sci Pollut Res Int 2020;27:1941-55.
58. Brumovský M, Bečanová J, Kohoutek J, Borghini M, Nizzetto L. Contaminants of emerging concern in the open sea waters of the Western Mediterranean. Environ Pollut 2017;229:976-83.
59. Feo ML, Bagnati R, Passoni A, et al. Pharmaceuticals and other contaminants in waters and sediments from Augusta Bay (southern Italy). Sci Total Environ 2020;739:139827.
60. Alygizakis NA, Gago-Ferrero P, Borova VL, Pavlidou A, Hatzianestis I, Thomaidis NS. Occurrence and spatial distribution of 158 pharmaceuticals, drugs of abuse and related metabolites in offshore seawater. Sci Total Environ 2016;541:1097-105.
61. Nödler K, Tsakiri M, Aloupi M, Gatidou G, Stasinakis AS, Licha T. Evaluation of polar organic micropollutants as indicators for wastewater-related coastal water quality impairment. Environ Pollut 2016;211:282-90.
62. Korkmaz NE, Savun-Hekimoğlu B, Aksu A, Burak S, Caglar NB. Occurrence, sources and environmental risk assessment of pharmaceuticals in the Sea of Marmara, Turkey. Sci Total Environ 2022;819:152996.
63. Korkmaz NE, Caglar NB, Aksu A. Presence and distribution of selected pharmaceutical compounds in water and surface sediment of the Golden Horn Estuary, Sea of Marmara, Turkey. Reg Stud Mar Sci 2022;51:102221.
64. Fang TH, Nan FH, Chin TS, Feng HM. The occurrence and distribution of pharmaceutical compounds in the effluents of a major sewage treatment plant in Northern Taiwan and the receiving coastal waters. Mar Pollut Bull 2012;64:1435-44.
65. Sun Q, Li Y, Li M, et al. PPCPs in Jiulong River estuary (China): spatiotemporal distributions, fate, and their use as chemical markers of wastewater. Chemosphere 2016;150:596-604.
66. Xie H, Hao H, Xu N, et al. Pharmaceuticals and personal care products in water, sediments, aquatic organisms, and fish feeds in the Pearl River Delta: Occurrence, distribution, potential sources, and health risk assessment. Sci Total Environ 2019;659:230-9.
67. Ismail NAH, Wee SY, Haron DEM, Kamarulzaman NH, Aris AZ. Occurrence of endocrine disrupting compounds in mariculture sediment of Pulau Kukup, Johor, Malaysia. Mar Pollut Bull 2020;150:110735.
68. Wu J, Qian X, Yang Z, Zhang L. Study on the matrix effect in the determination of selected pharmaceutical residues in seawater by solid-phase extraction and ultra-high-performance liquid chromatography-electrospray ionization low-energy collision-induced dissociation tandem mass spectrometry. J Chromatogr A 2010;1217:1471-5.
69. Bayen S, Zhang H, Desai MM, Ooi SK, Kelly BC. Occurrence and distribution of pharmaceutically active and endocrine disrupting compounds in Singapore’s marine environment: influence of hydrodynamics and physical-chemical properties. Environ Pollut 2013;182:1-8.
70. Bayen S, Segovia E, Loh LL, Burger DF, Eikaas HS, Kelly BC. Application of Polar Organic Chemical Integrative Sampler (POCIS) to monitor emerging contaminants in tropical waters. Sci Total Environ 2014;482-3:15-22.
71. Bayen S, Estrada ES, Juhel G, Kit LW, Kelly BC. Pharmaceutically active compounds and endocrine disrupting chemicals in water, sediments and mollusks in mangrove ecosystems from Singapore. Mar Pollut Bull 2016;109:716-22.
72. Vidal-Dorsch DE, Bay SM, Maruya K, Snyder SA, Trenholm RA, Vanderford BJ. Contaminants of emerging concern in municipal wastewater effluents and marine receiving water. Environ Toxicol Chem 2012;31:2674-82.
73. Comeau F, Surette C, Brun GL, Losier R. The occurrence of acidic drugs and caffeine in sewage effluents and receiving waters from three coastal watersheds in Atlantic Canada. Sci Total Environ 2008;396:132-46.
74. Pereira CDS, Maranho LA, Cortez FS, et al. Occurrence of pharmaceuticals and cocaine in a Brazilian coastal zone. Sci Total Environ 2016;548-9:148-54.
75. Fontes MK, Gusso-Choueri PK, Maranho LA, et al. A tiered approach to assess effects of diclofenac on the brown mussel Perna perna: a contribution to characterize the hazard. Water Res 2018;132:361-70.
76. Weigel S, Berger U, Jensen E, Kallenborn R, Thoresen H, Hühnerfuss H. Determination of selected pharmaceuticals and caffeine in sewage and seawater from Tromsø/Norway with emphasis on ibuprofen and its metabolites. Chemosphere 2004;56:583-92.
77. Brumovský M, Bečanová J, Kohoutek J, et al. Exploring the occurrence and distribution of contaminants of emerging concern through unmanned sampling from ships of opportunity in the North Sea. J Mar Syst 2016;162:47-56.
78. Togola A, Budzinski H. Analytical development for analysis of pharmaceuticals in water samples by SPE and GC-MS. Anal Bioanal Chem 2007;388:627-35.
79. Loos R, Tavazzi S, Paracchini B, Canuti E, Weissteiner C. Analysis of polar organic contaminants in surface water of the northern Adriatic Sea by solid-phase extraction followed by ultrahigh-pressure liquid chromatography-QTRAP® MS using a hybrid triple-quadrupole linear ion trap instrument. Anal Bioanal Chem 2013;405:5875-85.
80. Jiang JJ, Lee CL, Fang MD. Emerging organic contaminants in coastal waters: anthropogenic impact, environmental release and ecological risk. Mar Pollut Bull 2014;85:391-9.
81. Chen H, Chen W, Guo H, Lin H, Zhang Y. Pharmaceuticals and personal care products in the seawater around a typical subtropical tourist city of China and associated ecological risk. Environ Sci Pollut Res Int 2021;28:22716-28.
82. Chen WL, Ling YS, Lee DJH, Lin XQ, Chen ZY, Liao HT. Targeted profiling of chlorinated transformation products and the parent micropollutants in the aquatic environment: a comparison between two coastal cities. Chemosphere 2020;242:125268.
83. Waleng NJ, Nomngongo PN. Occurrence of pharmaceuticals in the environmental waters: African and Asian perspectives. Environ Chem Ecotoxicol 2022;4:50-66.
84. Long ER, Dutch M, Weakland S, Chandramouli B, Benskin JP. Quantification of pharmaceuticals, personal care products, and perfluoroalkyl substances in the marine sediments of Puget Sound, Washington, USA. Environ Toxicol Chem 2013;32:1701-10.
85. Postigo C, Moreno-Merino L, López-García E, López-Martínez J, López de Alda M. Human footprint on the water quality from the northern Antarctic Peninsula region. J Hazard Mater 2023;453:131394.
86. Ridgway J, Shimmield G. Estuaries as repositories of historical contamination and their impact on shelf seas. Estuar Coast Shelf Sci 2002;55:903-28.
87. Moreno-González R, Rodríguez-Mozaz S, Gros M, Pérez-Cánovas E, Barceló D, León VM. Input of pharmaceuticals through coastal surface watercourses into a Mediterranean lagoon (Mar Menor, SE Spain): sources and seasonal variations. Sci Total Environ 2014:490,59-72.
88. Rogers HR. Sources, behaviour and fate of organic contaminants during sewage treatment and in sewage sludges. Sci Total Environ 1996;185:3-26.
89. Fent K. Progestins as endocrine disrupters in aquatic ecosystems: concentrations, effects and risk assessment. Environ Int 2015;84:115-30.
90. Mezzelani M, Gorbi S, Fattorini D, et al. Transcriptional and cellular effects of Non-Steroidal Anti-Inflammatory Drugs (NSAIDs) in experimentally exposed mussels, Mytilus galloprovincialis. Aquat Toxicol 2016;180:306-19.
91. Courant F, Arpin-Pont L, Bonnefille B, et al. Exposure of marine mussels to diclofenac: modulation of prostaglandin biosynthesis. Environ Sci Pollut Res Int 2018;25:6087-94.
92. Freitas R, Coppola F, Costa S, et al. Does salinity modulates the response of Mytilus galloprovincialis exposed to triclosan and diclofenac? Environ Pollut 2019;251:756-65.
93. Freitas R, Coppola F, Costa S, et al. The influence of temperature on the effects induced by Triclosan and Diclofenac in mussels. Sci Total Environ 2019;663:992-9.
94. Mezzelani M, Gorbi S, Fattorini D, et al. Long-term exposure of Mytilus galloprovincialis to diclofenac, Ibuprofen and Ketoprofen: Insights into bioavailability, biomarkers and transcriptomic changes. Chemosphere 2018;198:238-48.
95. Álvarez-Ruiz R, Picó Y, Campo J. Bioaccumulation of emerging contaminants in mussel (
96. Bonnefille B, Arpin-Pont L, Gomez E, Fenet H, Courant F. Metabolic profiling identification of metabolites formed in Mediterranean mussels (Mytilus galloprovincialis) after diclofenac exposure. Sci Total Environ 2017;583:257-68.
97. Ericson H, Thorsén G, Kumblad L. Physiological effects of diclofenac, ibuprofen and propranolol on Baltic Sea blue mussels. Aquat Toxicol 2010;99:223-31.
98. Świacka K, Szaniawska A, Caban M. Evaluation of bioconcentration and metabolism of diclofenac in mussels Mytilus trossulus - laboratory study. Mar Pollut Bull 2019;141:249-55.
99. Costa S, Coppola F, Pretti C, et al. The influence of climate change related factors on the response of two clam species to diclofenac. Ecotoxicol Environ Saf 2020;189:109899.
100. Serrano MA, Gonzalez-Rey M, Mattos JJ, et al. Differential gene transcription, biochemical responses, and cytotoxicity assessment in Pacific oyster Crassostrea gigas exposed to ibuprofen. Environ Sci Pollut Res Int 2015;22:17375-85.
101. Mello FV, Cunha SC, Fogaça FHS, Alonso MB, Torres JPM, Fernandes JO. Occurrence of pharmaceuticals in seafood from two Brazilian coastal areas: implication for human risk assessment. Sci Total Environ 2022;803:149744.
102. Cunha SC, Pena A, Fernandes JO. Mussels as bioindicators of diclofenac contamination in coastal environments. Environ Pollut 2017;225:354-60.
103. Mezzelani M, Fattorini D, Gorbi S, Nigro M, Regoli F. Human pharmaceuticals in marine mussels: evidence of sneaky environmental hazard along Italian coasts. Mar Environ Res 2020;162:105137.
104. Capolupo M, Franzellitti S, Kiwan A, et al. A comprehensive evaluation of the environmental quality of a coastal lagoon (Ravenna, Italy): integrating chemical and physiological analyses in mussels as a biomonitoring strategy. Sci Total Environ 2017;598:146-59.
105. Wolecki D, Caban M, Pazdro K, Mulkiewicz E, Stepnowski P, Kumirska J. Simultaneous determination of non-steroidal anti-inflammatory drugs and natural estrogens in the mussels Mytilus edulis trossulus. Talanta 2019;200:316-23.
106. Omar TFT, Aris AZ, Yusoff FM, Mustafa S. Occurrence and level of emerging organic contaminant in fish and mollusk from Klang River estuary, Malaysia and assessment on human health risk. Environ Pollut 2019;248:763-73.
107. Cravo A, Silva S, Rodriguez J, et al. Understanding the bioaccumulation of pharmaceutical active compounds by clams Ruditapes decussatus exposed to a UWWTP discharge. Environ Res 2022;208:112632.
108. Granek EF, Conn KE, Nilsen EB, et al. Spatial and temporal variability of contaminants within estuarine sediments and native Olympia oysters: a contrast between a developed and an undeveloped estuary. Sci Tot Environ 2016;557-8:869-79.
109. Commission Regulation (EU) No 253/2011 of 15 March 2011 amending Regulation (EC) No 1907/2006 of the European Parliament and of the Council on the Registration, Evaluation, Authorisation and Restriction of Chemicals (REACH) as regards Annex XIII Text with EEA relevance. Available from: https://eur-lex.europa.eu/legal-content/EN/TXT/?uri=celex%3A32011R0253. [Last accessed on 2 Aug 2023].
110. Guideline on the environmental risk assessment of medicinal products for human use. European Medicines Agency, Committee for Medicinal Products for Human Use (CHMP). Available from: https://www.ema.europa.eu/en/documents/scientific-guideline/draft-guideline-environmental-risk-assessment-medicinal-products-human-use-revision-1_en.pdf. [Last accessed on 2 Aug 2023].
111. Freitas R, Almeida A, Pires A, et al. The effects of carbamazepine on macroinvertebrate species: comparing bivalves and polychaetes biochemical responses. Water Res 2015;85:137-47.
112. Contardo-Jara V, Lorenz C, Pflugmacher S, Nuntzmann G, Kloas W, Wiegand C. Molecular effects and bioaccumulation of levonorgestrel in the non-target organism Dreissena polymorpha. Environ Pollut 2011;159:38-44.
113. Beyer J, Green NW, Brooks S, et al. Blue mussels (Mytilus edulis spp.) as sentinel organisms in coastal pollution monitoring: a review. Mar Environ Res 2017;130:338-65.
114. Miller TH, Bury NR, Owen SF, MacRae JI, Barron LP. A review of the pharmaceutical exposome in aquatic fauna. Environ Pollut 2018;239:129-46.
115. UNESCO and HELCOM. Pharmaceuticals in the aquatic environment of the Baltic Sea region: a status report. Available from: https://www.helcom.fi/wp-content/uploads/2019/08/BSEP149.pdf. [Last accessed on 4 Aug 2023].
116. Eades C, Waring CP. The effects of diclofenac on the physiology of the green shore crab Carcinus maenas. Mar Environ Res 2010;69 Suppl:S46-8.
117. González-Ortegón E, Blasco J, Le Vay L, Giménez L. A multiple stressor approach to study the toxicity and sub-lethal effects of pharmaceutical compounds on the larval development of a marine invertebrate. J Hazard Mater 2013;263 Pt 1:233-8.
118. Pérez S, Rial D, Beiras R. Acute toxicity of selected organic pollutants to saltwater (mysid Siriella armata) and freshwater (cladoceran Daphnia magna) ecotoxicological models. Ecotoxicology 2015;24:1229-38.
119. Trombini C, Hampel M, Blasco J. Evaluation of acute effects of four pharmaceuticals and their mixtures on the copepod Tisbe battagliai. Chemosphere 2016;155:319-28.
120. Schmidt W, O’Rourke K, Hernan R, Quinn B. Effects of the pharmaceuticals gemfibrozil and diclofenac on the marine mussel (Mytilus spp.) and their comparison with standardized toxicity tests. Mar Pollut Bull 2011;62:1389-95.
121. Mauro M, Cammilleri G, Celi M, et al. Effects of diclofenac on the gametes and embryonic development of
122. Zanuri NB, Bentley MG, Caldwell GS. Assessing the impact of diclofenac, ibuprofen and sildenafil citrate (Viagra®) on the fertilisation biology of broadcast spawning marine invertebrates. Mar Environ Res 2017;127:126-36.
123. Ribeiro S, Torres T, Martins R, Santos MM. Toxicity screening of diclofenac, propranolol, sertraline and simvastatin using Danio rerio and Paracentrotus lividus embryo bioassays. Ecotoxicol Environ Saf 2015;114:67-74.
124. Schmidt W, Rainville LC, McEneff G, Sheehan D, Quinn B. A proteomic evaluation of the effects of the pharmaceuticals diclofenac and gemfibrozil on marine mussels (Mytilus spp.): evidence for chronic sublethal effects on stress-response proteins. Drug Test Anal 2014;6:210-9.
125. Jaafar SN, Coelho AV, Sheehan D. Redox proteomic analysis of mytilus edulis gills: effects of the pharmaceutical diclofenac on a non-target organism. Drug Test Anal 2015;7:957-66.
126. Balbi T, Montagna M, Fabbri R, et al. Diclofenac affects early embryo development in the marine bivalve Mytilus galloprovincialis. Sci Total Environ 2018;642:601-9.
127. Fabbri R, Montagna M, Balbi T, Raffo E, Palumbo F, Canesi L. Adaptation of the bivalve embryotoxicity assay for the high throughput screening of emerging contaminants in Mytilus galloprovincialis. Mar Environ Res 2014;99:1-8.
128. Gonzalez-Rey M, Bebianno MJ. Effects of non-steroidal anti-inflammatory drug (NSAID) diclofenac exposure in mussel Mytilus galloprovincialis. Aquat Toxicol 2014;148:221-30.
129. Bonnefille B, Gomez E, Alali M, Rosain D, Fenet H, Courant F. Metabolomics assessment of the effects of diclofenac exposure on Mytilus galloprovincialis: Potential effects on osmoregulation and reproduction. Sci Total Environ 2018;613-4:611-8.
130. Toufexi E, Dailianis S, Vlastos D, Manariotis ID. Mediated effect of ultrasound treated Diclofenac on mussel hemocytes: first evidence for the involvement of respiratory burst enzymes in the induction of DCF-mediated unspecific mode of action. Aquat Toxicol 2016;175:144-53.
131. Świacka K, Smolarz K, Maculewicz J, Caban M. Effects of environmentally relevant concentrations of diclofenac in Mytilus trossulus. Sci Total Environ 2020;737:139797.
132. Świacka K, Maculewicz J, Świeżak J, Caban M, Smolarz K. A multi-biomarker approach to assess toxicity of diclofenac and 4-OH diclofenac in Mytilus trossulus mussels - First evidence of diclofenac metabolite impact on molluscs. Environ Pollut 2022;315:120384.
133. Trombini C, Hampel M, Blasco J. Assessing the effect of human pharmaceuticals (carbamazepine, diclofenac and ibuprofen) on the marine clam Ruditapes philippinarum: an integrative and multibiomarker approach. Aquat Toxicol 2019;208:146-56.
134. Nunes B, Daniel D, Canelas GG, Barros J, Correia AT. Toxic effects of environmentally realistic concentrations of diclofenac in organisms from two distinct trophic levels, Hediste diversicolor and Solea senegalensis. Comp Biochem Physiol C Toxicol Pharmacol 2020;231:108722.
135. Maranho LA, Moreira LB, Baena-Nogueras RM, Lara-Martín PA, DelValls TA, Martín-Díaz ML. A candidate short-term toxicity test using Ampelisca brevicornis to assess sublethal responses to pharmaceuticals bound to marine sediments. Arch Environ Contam Toxicol 2015;68:237-58.
136. Aguirre-Martínez GV, Buratti S, Fabbri E, Del Valls TA, Martín-Díaz ML. Stability of lysosomal membrane in Carcinus maenas acts as a biomarker of exposure to pharmaceuticals. Environ Monit Assess 2013;185:3783-93.
137. Aguirre-Martínez GV, Del Valls TA, Martín-Díaz ML. Identification of biomarkers responsive to chronic exposure to pharmaceuticals in target tissues of Carcinus maenas. Mar Environ Res 2013;87-8:1-11.
138. Pusceddu FH, Choueri RB, Pereira CDS, et al. Environmental risk assessment of triclosan and ibuprofen in marine sediments using individual and sub-individual endpoints. Environ Pollut 2018;232:274-83.
139. Aguirre-Martínez GV, Owuor MA, Garrido-Pérez C, Salamanca MJ, Del Valls TA, Martín-Díaz ML. Are standard tests sensitive enough to evaluate effects of human pharmaceuticals in aquatic biota? Facing changes in research approaches when performing risk assessment of drugs. Chemosphere 2015;120:75-85.
140. Gonzalez-Rey M, Bebianno MJ. Non-steroidal anti-inflammatory drug (NSAID) ibuprofen distresses antioxidant defense system in mussel Mytilus galloprovincialis gills. Aquat Toxicol 2011;105:264-9.
141. Maria VL, Amorim MJ, Bebianno MJ, Dondero F. Transcriptomic effects of the non-steroidal anti-inflammatory drug Ibuprofen in the marine bivalve Mytilus galloprovincialis Lam. Mar Environ Res 2016;119:31-9.
142. Aguirre-Martínez GV, DelValls TA, Martín-Díaz ML. General stress, detoxification pathways, neurotoxicity and genotoxicity evaluated in Ruditapes philippinarum exposed to human pharmaceuticals. Ecotoxicol Environ Saf 2016;124:18-31.
143. Aguirre-Martínez GV, Buratti S, Fabbr E, DelValls AT, Martín-Díaz ML. Using lysosomal membrane stability of haemocytes in Ruditapes philippinarum as a biomarker of cellular stress to assess contamination by caffeine, ibuprofen, carbamazepine and novobiocin. J Environ Sci 2013;25:1408-18.
144. Milan M, Pauletto M, Patarnello T, Bargelloni L, Marin MG, Matozzo V. Gene transcription and biomarker responses in the clam Ruditapes philippinarum after exposure to ibuprofen. Aquat Toxicol 2013;126:17-29.
145. Matozzo V, Rova S, Marin MG. The nonsteroidal anti-inflammatory drug, ibuprofen, affects the immune parameters in the clam Ruditapes philippinarum. Mar Environ Res 2012;79:116-21.
146. Maranho LA, Baena-Nogueras RM, Lara-Martín PA, DelValls TA, Martín-Díaz ML. Bioavailability, oxidative stress, neurotoxicity and genotoxicity of pharmaceuticals bound to marine sediments. The use of the polychaete Hediste diversicolor as bioindicator species. Environ Res 2014;134:353-65.
147. Maranho LA, André C, DelValls TA, Gagné F, Martín-Díaz ML. Toxicological evaluation of sediment samples spiked with human pharmaceutical products: energy status and neuroendocrine effects in marine polychaetes Hediste diversicolor. Ecotoxicol Environ Saf 2015;118:27-36.
148. CEC (Commission of the European Communities), 1996. Part II. Available from: https://echa.europa.eu/documents/10162/987906/tgdpart2_2ed_en.pdf/138b7b71-a069-428e-9036-62f4300b752f. [Last accessed on 2 Aug 2023].
149. Almeida A, Solé M, Soares AMVM, Freitas R. Anti-inflammatory drugs in the marine environment: bioconcentration, metabolism and sub-lethal effects in marine bivalves. Environ Pollut 2020;263:114442.
150. Lotterhos KE, Levitan D. Gamete release and spawning behavior in broadcast spawning marine invertebrates. Available from: https://www.researchgate.net/publication/312449344. [Last accessed on 2 Aug 2023].
151. Pereira CDS, Abessa DMS, Choueri RB, et al. Ecological relevance of Sentinels’ biomarker responses: a multi-level approach. Mar Environ Res 2014;96:118-26.
152. Rowley AF, Vogan CL, Taylor GW, Clare AS. Prostaglandins in non-insectan invertebrates: recent insights and unsolved problems. J Exp Biol 2005;208:3-14.
153. Munari M, Chemello G, Finos L, Ingrosso G, Giani M, Marin MG. Coping with seawater acidification and the emerging contaminant diclofenac at the larval stage: a tale from the clam Ruditapes philippinarum. Chemosphere 2016;160:293-302.
154. Regoli F, Giuliani ME. Oxidative pathways of chemical toxicity and oxidative stress biomarkers in marine organisms. Mar Environ Res 2014;93:106-17.
155. Winston GW, Di Giulio RT. Prooxidant and antioxidant mechanisms in aquatic organisms. Aquatic Toxicology 1991;19:137-61.
156. Leone S, Ottani A, Bertolini A. Dual acting anti-inflammatory drugs. Curr Top Med Chem 2007;7:265-75.
157. Bertolini A, Ottani A, Sandrini M. Selective COX-2 inhibitors and dual acting anti-inflammatory drugs: critical remarks. Curr Med Chem 2002;9:1033-43.
158. Technical guidance document on risk assessment. In support of Commission directive 93/67/EEC on risk assessment for new notified substances, Commission Regulation (EC) No 1488/94 on risk assessment for existing substances, Directive 98/8/EC of the European Parliament and of the council concerning the placing of biocidal products on the market. Part I. Available from: https://echa.europa.eu/documents/10162/16960216/tgdpart1_2ed_en.pdf/f0fb9a44-13c9-44d7-897f-f7b3fe8ccacb. [Last accessed on 2 Aug 2023].
159. Beiras R. Environmental risk assessment of pharmaceutical and personal care products in estuarine and coastal waters. In: Durán-Álvarez JC, Jiménez-Cisneros B, editors. Pharmaceuticals in Marine and Coastal Environments. Elsevier; 2021. p. 195-252.
160. Durán I, Beiras R. Acute water quality criteria for polycyclic aromatic hydrocarbons, pesticides, plastic additives, and 4-Nonylphenol in seawater. Environ Pollut 2017;224:384-91.
161. Report of the OECD Workshop on the extrapolation of laboratory aquatic toxicity data on the real environment. Organization for Economic Cooperation and Development (OECD), OECD Environment Monographs 1992; No.59, Paris. Available from: https://www.oecd.org/chemicalsafety/testing/34528236.pdf. [Last accessed on 2 Aug 2023].
162. Hansen P. Risk assessment of emerging contaminants in aquatic systems. TrAC Trends Anal Chem 2007;26:1095-9.
163. Kötke D, Gandrass J, Xie Z, Ebinghaus R. Prioritised pharmaceuticals in German estuaries and coastal waters: occurrence and environmental risk assessment. Environ Pollut 2019;255:113161.
164. DeLorenzo ME, Fleming J. Individual and mixture effects of selected pharmaceuticals and personal care products on the marine phytoplankton species Dunaliella tertiolecta. Arch Environ Contam Toxicol 2008;54:203-10.
165. Minguez L, Pedelucq J, Farcy E, Ballandonne C, Budzinski H, Halm-lemeille M. Toxicities of 48 pharmaceuticals and their freshwater and marine environmental assessment in northwestern France. Environ Sci Pollut Res 2016;23:4992-5001.
166. Kuzmanović M, Ginebreda A, Petrović M, Barceló D. Risk assessment based prioritization of 200 organic micropollutants in 4 Iberian rivers. Sci Total Environ 2015;503-4:289-99.
167. Manual on the methodological framework to derive environmental quality standards for priority substances. Available from: https://www.helpdeskwater.nl/@177379/manual-environmental/. [Last accessed on 2 Aug 2023].
168. Posthuma L, Suter II GW, Traas TP. General introduction to species sensitivity distribution. In: Posthuma L, Traas TP, Suter II GW, editors. Species sensitivity distributions in ecotoxicology. CRC, Boca Ratón; 2002. p. 1-10. Available from: http://www.ievbras.ru/ecostat/Kiril/R/Ecotox/Leo%20Posthuma,%20Glenn%20W.%20Suter%20II,%20Theo%20P.%20Traas-Species%20Sensitivity%20Distributions%20in%20Ecotoxicology-CRC%20Press%20(2001).pdf. [Last accessed on 2 Aug 2023].
169. Yanagihara M, Hiki K, Iwasaki Y. Can chemical toxicity in saltwater be predicted from toxicity in freshwater? A comprehensive evaluation using species sensitivity distributions. Environ Toxicol Chem 2022;41:2021-7.
170. Piva F, Ciaprini F, Onorati F, et al. Assessing sediment hazard through a weight of evidence approach with bioindicator organisms: a practical model to elaborate data from sediment chemistry, bioavailability, biomarkers and ecotoxicological bioassays. Chemosphere 2011;83:475-85.
171. Benedetti M, Ciaprini F, Piva F, et al. A multidisciplinary weight of evidence approach for classifying polluted sediments: Integrating sediment chemistry, bioavailability, biomarkers responses and bioassays. Environ Int 2012;38:17-28.
172. Larsen JC. Basic concepts and terminology used to describe the combined action of chemicals in mixtures. In: Combined actions and interactions of chemicals mixtures. The toxicological effects of exposure to mixture of industrial and environmental chemicals. FødevareRapport; 2003. p. 20-32. Available from: https://www.food.dtu.dk/english/-/media/institutter/foedevareinstituttet/publikationer/pub-2003/combined-actions-and-interactions-of-chemicals-in-mixture.pdf?la=da&hash=1B5BD41C9DE06EEABABBAEC6124EF2C9C9FEFC72. [Last accessed on 2 Aug 2023].
173. Rodea-Palomares I, González-Pleiter M, Martín-Betancor K, Rosal R, Fernández-Piñas F. Additivity and interactions in ecotoxicity of pollutant mixtures: some patterns, conclusions, and open questions. Toxics 2015;3:342-69.
174. Kortenkamp A, Backhaus T, Faust M. State of the art report on mixture toxicity. Final report, Executive summary 2009. Available from: https://www.pan-europe.info/old/Campaigns/pesticides/documents/cum_syn_effects/Kortenkamp%20state%20of%20the%20art%20mixture%20toxicity.pdf. [Last accessed on 2 Aug 2023].
175. Schnell S, Bols NC, Barata C, Porte C. Single and combined toxicity of pharmaceuticals and personal care products (PPCPs) on the rainbow trout liver cell line RTL-W1. Aquat Toxicol 2009;93:244-52.
176. Coors A, Weisbrod B, Schoknecht U, Sacher F, Kehrer A. Predicting acute and chronic effects of wood preservative products in Daphnia magna and Pseudokirchneriella subcapitata based on the concept of concentration addition. Environ Toxicol Chem 2014;33:382-93.
177. Coors A, Vollmar P, Heim J, Sacher F, Kehrer A. Environmental risk assessment of biocidal products: identification of relevant components and reliability of a component-based mixture assessment. Environ Sci Eur 2018;30:3.
178. Puckowski A, Stolte S, Wagil M, et al. Mixture toxicity of flubendazole and fenbendazole to Daphnia magna. Int J Hyg Environ Health 2017;220:575-82.
179. Sigurnjak M, Ukić Š, Cvetnić M, et al. Combined toxicities of binary mixtures of alachlor, chlorfenvinphos, diuron and isoproturon. Chemosphere 2020;240:124973.
180. Backhaus T, Faust M. Predictive environmental risk assessment of chemical mixtures: a conceptual framework. Environ Sci Technol 2012;46:2564-73.
181. UK Environment Agency (Gov UK). The direct toxicity assessment of aqueous environmental samples using the marine copepod Tisbe battagliai lethality test (2007). Methods for the Examination of Waters and Associated Materials. Available from: https://assets.publishing.service.gov.uk/government/uploads/system/uploads/attachment_data/file/755574/tisbe210_jan26_1496121.pdf. [Last accessed on 2 Aug 2023].
182. Gonzalez-Rey M, Mattos JJ, Piazza CE, Bainy AC, Bebianno MJ. Effects of active pharmaceutical ingredients mixtures in mussel Mytilus galloprovincialis. Aquat Toxicol 2014;153:12-26.
183. Trombini C, Kazakova J, Villar-Navarro M, et al. Bioaccumulation and biochemical responses in the peppery furrow shell Scrobicularia plana exposed to a pharmaceutical cocktail at sub-lethal concentrations. Ecotoxicol Environ Saf 2022;242:113845.
184. Fabbri R, Montagna M, Balbi T, Raffo E, Palumbo F, Canesi L. Adaptation of the bivalve embryotoxicity assay for the high throughput screening of emerging contaminants in
185. Luna-Acosta A, Renault T, Thomas-Guyon H, et al. Detection of early effects of a single herbicide (diuron) and a mix of herbicides and pharmaceuticals (diuron, isoproturon, ibuprofen) on immunological parameters of Pacific oyster (Crassostrea gigas) spat. Chemosphere 2012;87:1335-40.
186. Munari M, Matozzo V, Gagné F, et al. Does exposure to reduced pH and diclofenac induce oxidative stress in marine bivalves? A comparative study with the mussel
187. Ankley GT, Bennett RS, Erickson RJ, et al. Adverse outcome pathways: a conceptual framework to support ecotoxicology research and risk assessment. Environ Toxicol Chem 2010;29:730-41.
Cite This Article
Export citation file: BibTeX | EndNote | RIS
OAE Style
Blasco J, Trombini C. Ibuprofen and diclofenac in the marine environment - a critical review of their occurrence and potential risk for invertebrate species. Water Emerg Contam Nanoplastics 2023;2:14. http://dx.doi.org/10.20517/wecn.2023.06
AMA Style
Blasco J, Trombini C. Ibuprofen and diclofenac in the marine environment - a critical review of their occurrence and potential risk for invertebrate species. Water Emerging Contaminants & Nanoplastics. 2023; 2(3): 14. http://dx.doi.org/10.20517/wecn.2023.06
Chicago/Turabian Style
Julian Blasco, Chiara Trombini. 2023. "Ibuprofen and diclofenac in the marine environment - a critical review of their occurrence and potential risk for invertebrate species" Water Emerging Contaminants & Nanoplastics. 2, no.3: 14. http://dx.doi.org/10.20517/wecn.2023.06
ACS Style
Blasco, J.; Trombini C. Ibuprofen and diclofenac in the marine environment - a critical review of their occurrence and potential risk for invertebrate species. Water. Emerg. Contam. Nanoplastics. 2023, 2, 14. http://dx.doi.org/10.20517/wecn.2023.06
About This Article
Copyright
Data & Comments
Data
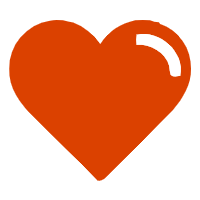
Comments
Comments must be written in English. Spam, offensive content, impersonation, and private information will not be permitted. If any comment is reported and identified as inappropriate content by OAE staff, the comment will be removed without notice. If you have any queries or need any help, please contact us at support@oaepublish.com.