Polymer engineering in hydrogel-based moisture-electric generators for green energy harvesting
Abstract
Hydrogel-based moisture-electric generators (HMEGs) have emerged as a promising technology for sustainable energy harvesting by utilizing ambient moisture. This article highlights recent advancements in HMEG development, focusing on innovative hydrogel designs to enhance energy output and practical applicability. Hydrogels provide a highly efficient medium for water absorption and ion transport, but their limited moisture generation performance necessitates polymer engineering strategies. Protonation doping and the incorporation of other cations, such as sodium ions, have been shown to significantly improve electrical output. Furthermore, dual-network hydrogels enhance both mechanical robustness and energy conversion efficiency. Future research should focus on improving scalability through large-scale fabrication techniques, enhancing durability under varying environmental conditions, and optimizing hydrogel properties for wearable and implantable applications. With continued material and engineering innovations, HMEGs hold great potential for advancing self-powered electronics and sustainable energy solutions.
Keywords
INTRODUCTION
As concerns over the energy crisis continue to rise, a diverse range of energy-harvesting technologies, such as osmotic energy harvesting[1,2], photovoltaic[3-5], piezoelectric[6-8], and thermoelectric systems[9-11], have been extensively studied. These technologies aim to meet the increasing demand for electricity required by biomedical devices[12-14], wearable electronics[15-18], smart city infrastructures[19-21], etc. Among these technologies, moisture-electric generators (MEGs) have emerged as a promising technology that harvests moisture from the environment to generate electricity without the need for mechanical, thermal, or solar inputs, which utilize the chemical potential difference induced by moisture absorption to drive ionic migration[22-25].
Since the phenomenon of moisture-generated electricity was first discovered in 2015 in a single graphene oxide (GO) film[26], recent years have witnessed the rapid development of MEGs. MEG offers significant advantages over conventional energy-harvesting methods[27-29]. For example, unlike solar cells that rely on sunlight or triboelectric devices that require mechanical input, MEGs can continuously generate electricity by utilizing ambient humidity, making them suitable for all-weather and indoor applications[30]. Moreover, MEGs can be fabricated from a wide range of materials, including polymers[31-33], metal oxides[34-36], carbon-based materials[37-40], and two-dimensional materials[41,42]. Their environmentally friendly and cost-effective fabrication process enables their widespread application in various fields, including sensing[43-46], medical treatment[47], and other cutting-edge technologies [Figure 1][18,47,48].
Hydrogels, as highly hydrophilic and ion-conductive materials[49-51], have received much attention in the development of high-performance MEGs. Their three-dimensional polymer network provides an efficient medium for fast water absorption and ion transport[52-55], making them ideal candidates for improving energy conversion efficiency. The excellent hydrophilicity and water retention capacity of hydrogels enable hydrogel-based MEGs (HMEGs) to sustain energy output even under extremely low ambient humidity conditions. Recent advances in HMEGs have focused on improving power output, operational stability, and mechanical robustness through innovative material designs, such as dual-network hydrogels, supramolecular hydrogels, and conductive hydrogels. HMEGs are engineered with more innovative designs, enabling them to be increasingly suitable for a wide range of practical applications. Here, we highlight the recent efforts in developing high-performance HMEGs and discuss future directions in developing next-generation HMEGs with better performance and wider applicability.
RECENT PROGRESS IN HMEGS
Figure 2 schematically illustrates the key milestones in the development trajectory of HMEGs. In 2020, He et al. first reported an HMEG based on a tannic acid (TA)–carbon nanotube (CNT)–glycerol–polyvinyl alcohol (PVA) conductive hydrogel, which exhibits excellent anti-freezing (-30 °C), moisturizing (retaining 70% weight after 10 days), mechanical strength (~1,000 kPa) and conductivity (5.13 S/m) properties[56]. This hydrogel utilizes PVA as the functional component, which releases protons upon moisture absorption, creating a proton concentration gradient that drives ionic migration, leading to electricity generation. The fabricated HMEG achieved an open-circuit voltage of 80 mV. A similar phenomenon was also observed in cellulose or polyacrylamide (PAM)-based conductive hydrogels[57,58]. These studies demonstrate the feasibility of using hydrogels in the fabrication of MEGs; however, the electrical performance of the output still requires further improvement.
Figure 2. Representative advancements in the development trajectory of HMEGs. Reproduced with permission[56]. Copyright©2020, Royal Society of Chemistry. Reproduced with permission[59]. Copyright©2022, Wiley-VCH, under CC BY 4.0. Reproduced with permission[55]. Copyright©2023, Wiley-VCH. Reproduced with permission[62]. Copyright©2024, Nature Publishing Group. HMEGs: Hydrogel-based moisture-electric generators.
Subsequently, Yang et al. further introduced phytic acid and glycerol into the PVA network[59]. Phytic acid provides abundant protons for the hydrogel, while the hydrophilic properties of glycerol enhance the affinity between the hydrogel and moisture. The HMEGs developed from this material exhibit excellent moisture collection ability and efficient ion transport, generating a stable direct current (DC) output of approximately 0.8 V for over 1,000 h. Its short-circuit current density reaches up to 0.24 mA·cm2 with a maximum power density of 35 µW·cm2, enabling power supply for small electronic devices such as calculators, electronic paper displays, and LED arrays. They found that in HMEG, proton conduction primarily arises from the vehicle mechanism. Under this mechanism, the transport of ions or protons is driven by the movement of mobile species such as H3O+ or H+·(H2O)n, which are dissociated from acid within the HMEG. These water clusters serve as conductive channels, effectively acting as “vehicles” for proton transport. This finding also highlights the crucial role of ionic conductivity in the generation of moisture-enabled electricity. This approach has achieved high-performance and all-weather operation of HMEG, but the mechanical properties of the hydrogel remain limited. Cheng et al. incorporated phytic acid into PAM hydrogels and observed similar enhancements in MEG performance[60]. The generator demonstrated outstanding electrical properties across a humidity range of 40%-90%, with its open-circuit voltage increasing from 0.675 to 0.838 V and a short-circuit current reaching 635.543 μA. These findings underscore the growing importance of proton doping as a key strategy for optimizing the performance of HMEGs.
In addition to incorporating small molecule acids (such as phytic acid), hydrogels with a high proton content can be synthesized by directly copolymerizing organic acids into the network. For example,
Besides proton, Yang et al. found that other ions (e.g., Na+) can similarly enhance power generation performance[62]. They reported that a supramolecular hydrogel composite of sodium alginate and PVA, featuring a high dissociable ion capacity, could achieve current output at the milliampere level
The generator maintains high performance even in harsh environments (e.g., cold and salty water). It successfully powers optical alarms and wireless communication systems, demonstrating the potential for use in wearable electronics, Internet of Things (IoT), and emergency field applications. The work emphasizes the potential of multi-source energy harvesting from water and proposes a path for improving the practicality and output of moisture-based generators.
SUMMARY AND OUTLOOK
In recent years, HMEGs have garnered significant attention due to their potential for sustainable energy harvesting from ambient moisture. This review highlights key advancements in HMEGs, with a focus on innovative designs in hydrogel materials. Hydrogels alone usually exhibit limited moisture generation performance and typically require polymer engineering to achieve superior energy output. Protonation doping has proven to be a highly effective approach, as the direct introduction of protons into the hydrogel network can significantly enhance both output voltage and current. Furthermore, copolymerizing proton-containing monomers with other mechanically robust monomers to form dual-network hydrogels results in HMEGs with outstanding mechanical properties and power generation capabilities. Existing studies also provide evidence that cations other than protons, such as sodium ions, can be incorporated into supramolecular hydrogel networks, enabling milliampere-level current outputs.
Although significant effort has been made, several key areas will require further development to unlock the full potential of HMEGs: Firstly, improving the output performance of HMEGs remains a critical objective (The output performance of reported HMEGs is summarized in Table 1)[56-75]. New strategies, such as combining concentration gradients with streaming potential or incorporating renewable energy sources such as solar or wind energy, may help improve voltage output and power density; Secondly, the development of flexible HMEGs suitable for wearable and even implantable electronics is crucial. While hydrogel materials are typically soft and stretchable, the fabrication of high-performance HMEGs still requires the integration of high-performance electrodes. The combination of novel flexible electrodes, such as silver nanowires, conductive polymers, and liquid metals, may prove to be beneficial; Thirdly, the compatibility of the fabrication processes of HMEGs with large-scale production technologies, such as screen printing and roll-to-roll printings. By aligning with these efficient and scalable manufacturing techniques, HMEGs can be produced at a significantly lower cost, enabling mass production and facilitating their integration into various industries. Indeed, the preparation of large-scale hydrogels still faces several challenges, including issues with uniformity, and stability, and the technical difficulties in achieving a stable interface with the electrodes. Additionally, the integration of circuit management techniques will play a crucial role in promoting practical applications; Fourthly, the durability and stability of HMEGs is a critical issue that requires attention. To ensure long-term performance, HMEGs must be capable of generating stable output across a range of temperatures and humidity levels. It is well known that hydrogels are highly sensitive to drying or freezing. Future efforts should focus on optimizing the water retention capacity of hydrogels and enhancing their ability to function reliably in both dry and extreme temperature conditions, whether high or low. Fifthly, ion migration plays an important role in moisture harvesting, which often results in slower response times for HMEGs. Therefore, further development of hydrogels with enhanced ion migration rates is needed. Sixthly, the process of moisture harvesting lacks a precise theoretical model description. The establishment of an energy harvesting equation will provide important theoretical guidance for future technological innovations.
Summary of the output performance of HMEGs
Ref. | Materials | RH (%) | Output voltage (V) | Current density (mA/cm2) | Maximum power density (µW/cm2) | Internal resistance |
[56] | TCGP | / | 0.08 | / | / | / |
[57] | ICOH | 90 | 0.17 | / | / | / |
[58] | MCTP | / | 0.164 | / | / | / |
[59] | PVA-phytic acid-glycerol hydrogel | 80 | 0.8 | 0.24 | 35 | 10 kΩ |
[60] | PAAGLE | 90 | 0.838 | 0.635 | 31.9 | 2 kΩ |
[55] | PAM-AMPS | 80 | 0.8 | 0.48 | 53.3 | 10 kΩ |
[61] | SCNF-PVA | 90 | 0.9 | 0.09 | 24 | 200 kΩ |
[62] | Sodium alginate-PVA | 80 | 1.3 | 1.31 | 110 | 1 kΩ |
[63] | SSG | 80 | 0.6 | 0.14 | / | / |
[65] | PAM-AMPS | 90 | 0.89 | 0.173 | / | / |
[66] | PPC/Li-PMC | 80 | 1 | / | 26.5 | 10 MΩ |
[67] | Natural rubber latex with acrylamide | 95 | 0.24 | 0.161 | 3.86 | / |
[68] | PAM-AMPS | 93 | 1.25 | 0.3 | 71.35 | 6 kΩ |
[69] | CPVPN | 80 | 0.34 | 0.033 | / | / |
[70] | LTH | 90 | ~0.8 | 0.065 | 14.35 | 12 kΩ |
[71] | Agarose-PAM | 40 | 0.3 | / | 0.7 | 2 kΩ |
[72] | PCP | 80 | 0.815 | 0.101 | 15.7 | 50 kΩ |
[73] | PAM | 60 | 1.28 | / | / | / |
[74] | Polyacrylic acid | 55 | 0.55 | 0.003 | / | 200 kΩ |
[75] | Poly(acrylamide-acrylamide) | 90 | 1.3 | 55.7 | 2.34 | 500 kΩ |
In summary, the future of MEGs holds immense potential, with significant prospects for further improvements in performance, durability, and versatility. Ongoing innovation in material design, fabrication techniques, and integration strategies will be key to ensuring that MEGs meet the diverse demands of applications ranging from wearable electronics to environmental sensors. At the same time, these advancements will contribute to the global shift toward more sustainable energy solutions.
DECLARATIONS
Authors’ contributions
Data analysis and interpretation: Zhang, H.; Sun, M.; Meng, Q.; Li, H.
Provided administrative, technical, and material support: Tian, Y.
Wrote and reviewed draft: Zhang, H.; Sun, M.; Tian, Y.
Funding aquarian: Tian, Y.; Zhang, H.
Availability of data and materials
Not applicable.
Financial support and sponsorship
This work was supported by the National Natural Science Foundation of China (Grant No. 52175300), Heilongjiang Province Key Research and Development Program (Grant No. 2022XJ03C07), and State Key Laboratory of Precision Welding & Joining of Materials and Structures (MSWJ-24M11).
Conflicts of interest
All authors declared that there are no conflicts of interest.
Ethical approval and consent to participate
Not applicable.
Consent for publication
Not applicable.
Copyright
© The Author(s) 2025.
REFERENCES
1. Mohan, B.; Singh, K.; Ahmadov, E.; Pombeiro, A. J.; Ren, P. Harvesting sustainable osmotic energy: the art of nanofluidic hydrogel membranes. J. Energy. Chem. 2025, 105, 577-94.
2. Mohan, B.; Singh, K.; Gupta, R. K.; Pombeiro, A. J.; Ren, P. Advanced materials for energy harvesting: exploring the potential of MOFs and MXene membranes in osmotic energy applications. Prog. Mater. Sci. 2025, 152, 101457.
3. Cui, Y.; Xu, Y.; Yao, H.; et al. Single-junction organic photovoltaic cell with 19% efficiency. Adv. Mater. 2021, 33, 2102420.
4. Aydin, E.; Allen, T. G.; De Bastiani, M.; et al. Pathways toward commercial perovskite/silicon tandem photovoltaics. Science 2024, 383, eadh3849.
5. Chen, J.; Deger, C.; Su, Z. H.; et al. Magnetic-biased chiral molecules enabling highly oriented photovoltaic perovskites. Natl. Sci. Rev. 2024, 11, nwad305.
6. Zhang, W.; Zhang, Y.; Yan, X.; Hong, Y.; Yang, Z. Challenges and progress of chemical modification in piezoelectric composites and their applications. Soft. Sci. 2023, 3, 19.
7. Jarkov, V.; Califano, D.; Tsikriteas, Z. M.; Bowen, C. R.; Adams, C.; Khanbareh, H. 3D piezoelectric cellulose composites as advanced multifunctional implants for neural stem cell transplantation. Cell. Rep. Phys. Sci. 2025, 6, 102368.
8. Chen, A.; Su, J.; Li, Y.; et al. 3D/4D printed bio-piezoelectric smart scaffolds for next-generation bone tissue engineering. Int. J. Extrem. Manuf. 2023, 5, 032007.
9. Liu, X.; Huang, J.; Du, Y.; Wang, L.; Eklund, P. Enhanced thermoelectric properties of flexible self-supporting carbon nanotube film/polypyrrole composites. Cell. Rep. Phys. Sci. 2024, 5, 102163.
10. Tang, X.; Qi, C.; Sun, Q. Recent progress of biosensors based on thermoelectric effects for monitoring physical activity and environment monitoring. Soft. Sci. 2025, 5, 11.
11. Chang, Z.; Liu, K.; Sun, Z.; et al. First-principles investigation of the significant anisotropy and ultrahigh thermoelectric efficiency of a novel two-dimensional Ga2I2S2 at room temperature. Int. J. Extrem. Manuf. 2022, 4, 025001.
12. Chen, S.; Zhu, P.; Mao, L.; et al. Piezocatalytic medicine: an emerging frontier using piezoelectric materials for biomedical applications. Adv. Mater. 2023, 35, e2208256.
13. Ji, J.; Yang, C.; Shan, Y.; et al. Research trends of piezoelectric nanomaterials in biomedical engineering. Adv. NanoBiomed. Res. 2023, 3, 2200088.
14. Pan, X.; Wu, Y.; Wang, Y.; Zhou, G.; Cai, H. Mechanical energy harvesting based on the piezoelectric materials: recent advances and future perspectives. Chem. Eng. J. 2024, 497, 154249.
15. Mahanty, B.; Kumar Ghosh, S.; Lee, D. Advancements in polymer nanofiber-based piezoelectric nanogenerators: revolutionizing self-powered wearable electronics and biomedical applications. Chem. Eng. J. 2024, 495, 153481.
16. Tsikriteas, Z. M.; Roscow, J. I.; Bowen, C. R.; Khanbareh, H. Exploring lead-free materials for screen-printed piezoelectric wearable devices. Cell. Rep. Phys. Sci. 2024, 5, 101962.
17. Zhang, C.; Fan, W.; Wang, S.; Wang, Q.; Zhang, Y.; Dong, K. Recent progress of wearable piezoelectric nanogenerators. ACS. Appl. Electron. Mater. 2021, 3, 2449-67.
18. Li, Q.; Qin, Y.; Cheng, D.; et al. Moist-electric generator with efficient output and scalable integration based on carbonized polymer dot and liquid metal active electrode. Adv. Funct. Mater. 2023, 33, 2211013.
19. Izadgoshasb, I. Piezoelectric energy harvesting towards self-powered Internet of Things (IoT) sensors in smart cities. Sensors 2021, 21, 8332.
20. Guo, L.; Wang, H. Non-intrusive movable energy harvesting devices: materials, designs, and their prospective uses on transportation infrastructures. Renew. Sustain. Energy. Rev. 2022, 160, 112340.
21. Akin-Ponnle, A. E.; Carvalho, N. B. Energy harvesting mechanisms in a smart city - a review. Smart. Cities. 2021, 4, 476-98.
22. Cao, Y.; Xu, B.; Li, Z.; Fu, H. Advanced design of high-performance moist-electric generators. Adv. Funct. Mater. 2023, 33, 2301420.
23. Feng, J.; Wen, J.; Wang, S.; et al. Graphene oxide-based planar hygroelectric generator and its applications in a flexible self-powered sensing system. ACS. Appl. Nano. Mater. 2024, 7, 1646-54.
24. Feng, J.; Xiao, M.; Hui, Z.; et al. High-performance magnesium-carbon nanofiber hygroelectric generator based on interface-mediation-enhanced capacitive discharging effect. ACS. Appl. Mater. Interfaces. 2020, 12, 24289-97.
25. Shen, D.; Li, F.; Zhao, J.; et al. Ionic hydrogel-based moisture electric generators for underwater electronics. Adv. Sci. 2024, 11, e2408954.
26. Zhao, F.; Cheng, H.; Zhang, Z.; Jiang, L.; Qu, L. Direct power generation from a graphene oxide film under moisture. Adv. Mater. 2015, 27, 4351-7.
27. Sun, Z.; Wen, X.; Wang, L.; et al. Emerging design principles, materials, and applications for moisture-enabled electric generation. eScience 2022, 2, 32-46.
28. Ni, F.; Xiao, P.; Zhang, C.; Chen, T. Hygroscopic polymer gels toward atmospheric moisture exploitations for energy management and freshwater generation. Matter 2022, 5, 2624-58.
29. Wei, Q.; Ge, W.; Yuan, Z.; et al. Moisture electricity generation: mechanisms, structures, and applications. Nano. Res. 2023, 16, 7496-510.
30. Shen, D.; Duley, W. W.; Peng, P.; et al. Moisture-enabled electricity generation: from physics and materials to self-powered applications. Adv. Mater. 2020, 32, e2003722.
31. Yan, H.; Liu, Z.; Qi, R. A review of humidity gradient-based power generator: devices, materials and mechanisms. Nano. Energy. 2022, 101, 107591.
32. Wang, H.; Sun, Y.; He, T.; et al. Bilayer of polyelectrolyte films for spontaneous power generation in air up to an integrated 1,000 V output. Nat. Nanotechnol. 2021, 16, 811-9.
33. He, T.; Wang, H.; Lu, B.; et al. Fully printed planar moisture-enabled electric generator arrays for scalable function integration. Joule 2023, 7, 935-51.
34. Liu, C.; Wan, T.; Guan, P.; et al. Unveil the triple roles of water molecule on power generation of MXene derived TiO2 based moisture electric generator. Adv. Energy. Mater. 2024, 14, 2400590.
35. Shen, D.; Xiao, M.; Zou, G.; Liu, L.; Duley, W. W.; Zhou, Y. N. Self-powered wearable electronics based on moisture enabled electricity generation. Adv. Mater. 2018, 30, 1705925.
36. Akbarisehat, A.; Zangari, G. Electricity generation via metal oxide-air moist interaction. Mater. Today. Commun. 2024, 40, 109744.
37. Dinh Trung, V.; Chen, S.; Xia, H.; Natsuki, T.; Ni, Q. A moisture-induced electric generator with high output voltage for self-powered wearable electronics. ChemNanoMat 2022, 8, e202200395.
38. Liu, H.; Han, Y.; Zhang, X.; et al. Graphene oxide sponge with gradient porosity for moisture-electric generator. J. Bionic. Eng. 2025, 22, 783-92.
39. Qi, X.; Miao, T.; Chi, C.; et al. Ultralight PEDOT:PSS/graphene oxide composite aerogel sponges for electric power harvesting from thermal fluctuations and moist environment. Nano. Energy. 2020, 77, 105096.
40. Huang, Y.; Cheng, H.; Shi, G.; Qu, L. Highly efficient moisture-triggered nanogenerator based on graphene quantum dots. ACS. Appl. Mater. Interfaces. 2017, 9, 38170-5.
41. Feng, Z.; Hu, G.; Zhu, R.; et al. Two-dimensional nanomaterials for moisture-electric generators: a review. ACS. Appl. Nano. Mater. 2022, 5, 12224-44.
42. Zhao, K.; Lee, J. W.; Yu, Z. G.; et al. Humidity-tolerant moisture-driven energy generator with MXene aerogel-organohydrogel bilayer. ACS. Nano. 2023, 17, 5472-85.
43. Li, L.; Chen, Z.; Hao, M.; et al. Moisture-driven power generation for multifunctional flexible sensing systems. Nano. Lett. 2019, 19, 5544-52.
44. Feng, J.; Wei, N.; Sun, Z.; Li, S.; Li, X.; Xia, H. Multifunctionally moist-electric generator for self-powered ultra-fast sensing based on laterally dual gradient. Nano. Energy. 2024, 123, 109409.
45. Zhang, R.; Chen, X.; Wan, Z.; et al. High-performance, flexible moist-electric generator for self-powered wearable wireless sensing. Chem. Eng. J. 2024, 502, 157695.
46. Gao, H.; Feng, Z.; Li, W.; et al. High-performance biomass moisture-electric generators derived from Distillers’ spent grains: synthesis, photothermal enhancement, and environmental sensing applications. Chem. Eng. J. 2025, 504, 158880.
47. Yan, R.; Zhang, X.; Wang, H.; et al. Autonomous, moisture-driven flexible electrogenerative dressing for enhanced wound healing. Adv. Mater. 2025, 37, e2418074.
48. Zhang, H.; He, N.; Wang, B.; et al. High-performance, highly stretchable, flexible moist-electric generators via molecular engineering of hydrogels. Adv. Mater. 2023, 35, 2300398.
49. Wang, Z.; Wang, S.; Zhang, L.; Liu, H.; Xu, X. Highly strong, tough, and cryogenically adaptive hydrogel ionic conductors via coordination interactions. Research 2024, 7, 0298.
50. Khan, M.; Rahman, T. U.; Sher, M.; et al. Flexible ionic conductive hydrogels with wrinkled texture for flexible strain transducer with language identifying diversity. Chem. Mater. 2024, 36, 4703-13.
51. Chen, D.; Bai, H.; Zhu, H.; Zhang, S.; Wang, W.; Dong, W. Anti-freezing, tough, and stretchable ionic conductive hydrogel with multi-crosslinked double-network for a flexible strain sensor. Chem. Eng. J. 2024, 480, 148192.
52. Wu, Z.; Liu, X.; Xu, Q.; et al. Poly(vinyl alcohol)/polyacrylamide double-network ionic conductive hydrogel strain sensor with high sensitivity and high elongation at break. J. Polym. Sci. 2024, 62, 4599-611.
53. Lei, T.; Wang, Y.; Feng, Y.; et al. PNIPAAm-based temperature responsive ionic conductive hydrogels for flexible strain and temperature sensing. J. Colloid. Interface. Sci. 2025, 678, 726-41.
54. Ara, L.; Sher, M.; Khan, M.; Rehman, T. U.; Shah, L. A.; Yoo, H. M. Dually-crosslinked ionic conductive hydrogels reinforced through biopolymer gellan gum for flexible sensors to monitor human activities. Int. J. Biol. Macromol. 2024, 276, 133789.
55. Chen, M.; Liu, H.; Chen, X.; et al. A novel multifunction of wearable ionic conductive hydrogel sensor for promoting infected wound healing. Appl. Mater. Today. 2024, 39, 102298.
56. He, P.; Wu, J.; Pan, X.; et al. Anti-freezing and moisturizing conductive hydrogels for strain sensing and moist-electric generation applications. J. Mater. Chem. A. 2020, 8, 3109-18.
57. Pan, X.; Wang, Q.; Guo, R.; et al. An adaptive ionic skin with multiple stimulus responses and moist-electric generation ability. J. Mater. Chem. A. 2020, 8, 17498-506.
58. He, P.; Guo, R.; Hu, K.; et al. Tough and super-stretchable conductive double network hydrogels with multiple sensations and moisture-electric generation. Chem. Eng. J. 2021, 414, 128726.
59. Yang, S.; Tao, X.; Chen, W.; et al. Ionic Hydrogel for efficient and scalable moisture-electric generation. Adv. Mater. 2022, 34, e2200693.
60. Cheng, Y.; Yang, C.; Zhu, T.; Wu, C.; Huang, J.; Lai, Y. Light-assisted polyproton dissociated PAAm-PA hydrogel-based moisture-driven electricity generator with a broad operating range. Adv. Funct. Mater. 2025, 35, 2415533.
61. Mo, J.; Wang, X.; Lin, X.; et al. Sulfated cellulose nanofibrils-based hydrogel moist-electric generator for energy harvesting. Chem. Eng. J. 2024, 491, 152055.
62. Yang, S.; Zhang, L.; Mao, J.; et al. Green moisture-electric generator based on supramolecular hydrogel with tens of milliamp electricity toward practical applications. Nat. Commun. 2024, 15, 3329.
63. Huang, Z.; Li, C.; Ying, W.; et al. A hydrogel-based moist-electric generator with superior energy output and environmental adaptability. Nano. Energy. 2024, 126, 109673.
64. Li, F.; Zhao, J.; Li, B.; et al. Water-triboelectrification-complemented moisture electric generator. ACS. Nano. 2024, 18, 30658-67.
65. Cheng, Y.; Zhu, T.; He, Q.; et al. Hydrogel-based moisture electric generator with high output performance induced by proton hopping. Adv. Funct. Mater. 2025, 2500186.
66. Huang, G.; Liu, J.; Zhang, H.; Zhang, W.; Deng, Y.; Sha, J. A double-gradient structured hydrogel for an efficient moisture-electric generator. Chem. Eng. J. 2025, 504, 158878.
67. Guchait, A.; Pramanik, S.; Goswami, D. K.; Chattopadhyay, S.; Mondal, T. Elastomeric ionic hydrogel-based flexible moisture-electric generator for next-generation wearable electronics. ACS. Appl. Mater. Interfaces. 2024, 16, 46844-57.
68. Fang, J.; Zhang, X.; Duan, P.; et al. Efficient and cold-tolerant moisture-enabled power generator combining ionic diode and ionic hydrogel. Mater. Horiz. 2024, 11, 1261-71.
69. Ma, G.; Li, W.; Zhou, X.; et al. PVA–PNIPAM hydrogel-based moisture-electric generators with tunable pore structures for enhanced power generation. ACS. Appl. Polym. Mater. 2024, 6, 7066-76.
70. Yu, F.; Wang, L.; Yang, X.; et al. Moisture-electric generators working in subzero environments based on laser-engraved hygroscopic hydrogel arrays. ACS. Nano. 2025, 19, 3807-17.
71. Wen, X.; Sun, Z.; Xie, X.; et al. High-performance fully stretchable moist-electric generator. Adv. Funct. Mater. 2024, 34, 2311128.
72. Zhang, H.; Qin, L.; Zhou, Y.; Huang, G.; Cai, H.; Sha, J. High-performance and anti-freezing moisture-electric generator combining ion-exchange membrane and ionic hydrogel. Small 2025, 21, e2410609.
73. Duan, W.; Shao, B.; Wang, Z.; et al. Silicon nanowire/ionic hydrogel-based hybrid moist-electric generators with enhanced voltage output and operational stability. Energy. Environ. Sci. 2024, 17, 3788-96.
74. Zhang, J.; Zhuang, J.; Lei, L.; Hou, Y. Rapid preparation of a self-adhesive PAA ionic hydrogel using lignin sulfonate–Al3+ composite systems for flexible moisture-electric generators. J. Mater. Chem. A. 2023, 11, 3546-55.
Cite This Article

How to Cite
Download Citation
Export Citation File:
Type of Import
Tips on Downloading Citation
Citation Manager File Format
Type of Import
Direct Import: When the Direct Import option is selected (the default state), a dialogue box will give you the option to Save or Open the downloaded citation data. Choosing Open will either launch your citation manager or give you a choice of applications with which to use the metadata. The Save option saves the file locally for later use.
Indirect Import: When the Indirect Import option is selected, the metadata is displayed and may be copied and pasted as needed.
About This Article
Copyright
Data & Comments
Data
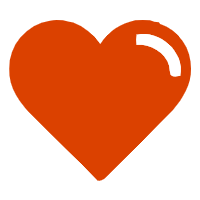
Comments
Comments must be written in English. Spam, offensive content, impersonation, and private information will not be permitted. If any comment is reported and identified as inappropriate content by OAE staff, the comment will be removed without notice. If you have any queries or need any help, please contact us at [email protected].