Multiphase manganese-based layered oxide for sodium-ion batteries: structural change and phase transition
Abstract
Sodium-ion batteries (SIBs) are recognized as a leading option for energy storage systems, attributed to their environmental friendliness, natural abundance of sodium, and uncomplicated design. Cathode materials are crucial in defining the structural integrity and functional efficacy of SIBs. Recent studies have extensively focused on manganese (Mn)-based layered oxides, primarily due to their substantial specific capacity, cost-effectiveness, non-toxic nature, and ecological compatibility. Additionally, these materials offer a versatile voltage range and diverse configurational possibilities. However, the complex phase transition during a circular process affects its electrochemical performance. Herein, we set the multiphase Mn-based layered oxides as the research target and take the relationship between the structure and phase transition of these materials as the starting point, aiming to clarify the mechanism between the microstructure and phase transition of multiphase layered oxides. Meanwhile, the structure-activity relationship between structural changes and electrochemical performance of Mn-based layered oxides is revealed. Various modification methods for multiphase Mn-based layered oxides are summarized. As a result, a reasonable structural design is proposed for producing high-performance SIBs based on these oxides.
Keywords
INTRODUCTION
Energy is the foundation of human survival and development[1-3]. Nevertheless, as the economy has rapidly developed, energy shortages, pollution, and other issues have increased in prominence[4-6]. In recent years, clean and renewable energy has been the focus of research[7-10]. Electrochemical energy storage devices have also seen an increase in demand year after year, and energy storage systems, including secondary batteries, have received wide attention[11-13]. Currently, lithium secondary batteries have found widespread application in various domains, including portable electronic gadgets, electric vehicles, and numerous other sectors[14-16]. Nevertheless, the heightened demand for lithium resources, coupled with the uneven geographical distribution of lithium mines and the complexities associated with extraction, have spurred growing interest in alternative energy storage technologies[17-21]. Sodium-ion batteries (SIBs) are anticipated to facilitate substantial energy storage solutions, primarily because of their economical nature and the plentiful availability of sodium resources[22-26]. Being a critical element of SIBs, the cathode material significantly influences their specific capacities, energy densities, power densities, and overall lifespan[27-30]. However, due to the large size of Na+ (1.02 Å) embedded in/out of the electrode structure, the material will produce irreversible volume and structural deformation, resulting in capacity decay, limiting the development of SIBs[31-34]. Therefore, it is crucial to develop a stable cathode material.
Within the scope of cathode materials, transition metal (TM) layered oxides, symbolized as NaxMO2 (where M stands for a TM), have emerged as the most significant for SIBs. This prominence is attributed to the periodic layered architecture, straightforward synthesis methodology, and high specific capacity and voltage capabilities[35-38]. Manganese (Mn) is the most abundant raw material on earth; NaxMO2 and its derivatives synthesized from Mn as the TM have been widely studied[39-42]. Mn-based oxides mainly include the tunnel-type, spinel-type, and layered oxides. The common synthesis methods of manganese oxides include the solid phase, coprecipitation, sol-gel, and spray drying techniques[43]. Based on the coordination configuration of sodium ions in TMO6 (TM = Ni, Co, Mn, Fe) polyhedral and the stacking mode of oxygen, sodium-manganese layered oxides (NMLOs) can be divided into four main categories: O3, O2, P3, P2, etc. Nonetheless, Mn3+ is prone to the Jahn-Teller effect. High spin Mn3+ in MnO6 octahedral units in the materials leads to serious lattice distortion, which usually has a serious adverse influence on structural stability and electrochemical performance[44]. According to the coordination configuration of Na+ and the stacking mode of oxygen, NaxMnO2 exists mainly in O3-type and P2-type structures[45-48]. O3 and P2 structural materials have their own advantages[49-51]. Specifically, there is only one occupation of sodium ions in O3-type Mn-based oxides. Therefore, the initial sodium content of O3 phase material is higher than that of P2 phase material, and the capacity is larger[52-54]. While the interlayer spacing of P2-type structure is larger, the P2-type Mn-based layered material has a wider sodium ion transport channel[36]. Na+ can diffuse directly between two adjacent triangular prism sites through this channel. Therefore, P2-type Mn-based layered cathode materials can exhibit a high rate capability[55]. O3-type and P2-type materials both suffer problems such as the Jahn-Teller effect, dissolution of transition manganese, complex phase transition of crystal structure and air stability.
In order to study the mechanism of manganese oxides, in-situ X-ray diffraction (XRD) and synchrotron radiation X-ray absorption spectroscopy can effectively monitor the structural and valence changes and dig out the phase transition mechanism of the Mn-based oxides. The intricate phase transition observed in
In this review, we consolidate the findings on the structural characteristics and phase transitions of multiphase Mn-based layered oxide materials utilized in SIBs. Additionally, we delve into their evolution, structural tuning, interface alterations, and various other modification techniques [Figure 1]. By comprehensively examining the development status of multiphase Mn-based layered materials, we reveal the phase transition process of multiphase structures and clarify different modification strategies to obtain a stable circular process. Furthermore, we have proposed several recommendations and outlooks regarding the future advancement of Mn-based layered oxide materials as cathodic components in SIBs.
DEVELOPMENT OF MULTIPHASE MN-BASED LAYERED MATERIALS
Currently, various O3 or P2 phase Mn-based layered materials, both single and multiple, have been reported as cathodes for SIBs, which have shown good performance. However, there are still deficiencies in terms of capacity, stability, and power. For this reason, a comprehensive structural advantage strategy combining O3 and P2 phases was proposed in order to enhance electrochemical performance. As shown in Figure 2A,
Figure 2. (A) The SAED patterns of the P2 + O3 NaLiMNC composite: O3-type structure and P2-type structure; The STEM images: HADDF andABF images of P2 + O3 NaLiMNC composite; the blue and red rectangle represent O3 structure and P2 structure areas (Reproduced with permission from Ref.[73]. Copyright 2015, John Wiley and Sons); (B) In-situ XRD patterns collected during the first charge/discharge of NNFM-0.78 cell under 0.1 Cover a voltage range of 2.5-4.0 V, with the corresponding electrochemical charge-discharge profile (Reproduced with permission from Ref.[74]. Copyright 2017, American Chemical Society); (C) XRD patterns of
The substitution of copper (Cu) is noted to facilitate the transformation of the P2 phase to the O3 phase. By fine-tuning the quantity of Cu, a P2/O3 composite structure is achievable. Furthermore, due to the synergetic effect of the composite phase and Cu substitution, in-situ XRD analyses reveal that this composite configuration, comprising P2 and O3 phases, enables highly reversible phase transitions and minimizes lattice discrepancies during Na+ ion insertion/extraction. After cycling 100 times at a rate of 1 C, the dual-phase Na0.67Fe0.425Mn0.425Cu0.15O2 cathode exhibits a capacity retention of 87.1%. In contrast, the single-phase P2-Na0.67Fe0.425Mn0.425Cu0.15O2 electrode shows a capacity retention of only 36.4%. Consequently, the dual-phase structures are confirmed as an effective approach to implement high-performing Fe/Mn-based layered oxide cathodes.
In addition to the Fe/Mn system, comprehensive studies have been conducted on multi-component P2/O3 dual-phase materials, primarily focusing on Mn-based components. As depicted in Figure 3A, Zhang et al. have synthesized a multi-component P2/O3 dual-phase material Na0.732Ni0.273Mg0.096Mn0.63O2 comprising 78.39% P2 phase Na0.67Ni0.33Mn0.67O2 and 21.61% O3 phase NaNi0.5Mn0.5O2[66]. Although possessing identical elemental compositions, the two phases show distinct crystal structures. Structural assessments and density functional theory (DFT) calculations reveal that the composite material tends to form an atomic-level symbiotic structure. The intricate lattice configuration of the dual-phase structure acts as a barrier against undesirable ion and oxygen movement during electrode processes. As a result, this dual-phase framework notably enhances electrochemical performance while preserving exceptional reversibility in anionic oxygen redox reactions. Moreover, the coexistence of heteroepitaxial structures in P2 and O3 phases creates multiphase boundaries, which lead to an interlocking effect due to their differing electrochemical behaviors. This phenomenon helps prevent significant structural degradation and reduces the lattice strain associated with Na+ deintercalation and intercalation. As a result, the co-existing P2/O3 composites demonstrate increased capacity and cycle performance and reversible structural evolution. As presented in Figure 3B, Wang et al. have documented the creation and detailed analysis of a new P2/O3 intercalated
Figure 3. (A) TEM images of P2/O3-Com950 0.8 materials with the insert of SEAD image and partly enlarged graphic and SEM images of P2/O3-Com950 0.8 materials (Reproduced with permission from Ref.[66]. Copyright 2024, Elsevier); (B) XRD patterns of
Hong et al. successfully synthesized P2/O3 dual-phase cathode Na0.73Ni0.4Mn0.4Ti0.2O2 using the solid-phase method by mixing P2 and O3, and the content of P2 phase was 33%[78]. Based on the results from XRD refinement, it was observed that the interlayer spacing along the c-axis in the pure P2 material decreased from 5.6055 to 5.5710 Å in the dual-phase P2 phase. Conversely, the interlayer spacing in the pure O3 material expanded from 5.4502 to 5.4855 Å in the dual-phase O3 phase. This indicates varying electrostatic repulsion among the TM oxide layers in these two phases. Compared with the singular P2 or O3 phase, the dual-phase cathode exhibits a high initial Coulombic efficiency near 100%, with enhanced specific discharge capacity and average voltage. As illustrated in Figure 3C, Xu et al. analyzed the sodium ion deintercalation process in sodium-containing layered metal oxides NaxTMO2 (TM = Ni, Cu, Fe, Mn) within both the P2 and O3 phases with electrochemical collision method. Their findings highlight distinct electrochemical behaviors between the two materials[79]. In O3-type oxides, the likelihood of Na+ diffusion within a single particle is higher than that in P2-type oxides. This observation contradicts the conventional belief that P2-type cathodes generally offer greater Na+ conductivity than their O3 counterparts. As demonstrated, dual-phase and multiphase cathodes are exceedingly advantageous for high-energy SIBs despite the structural phase transitions during cycling being considerably intricate. For the stabilization of multiphase materials, it is imperative to strategically design their morphology and structure to enhance their electrochemical characteristics. Figure 3D shows the computed energy profiles for the sodium ion diffusion paths discussed above[55]. The dumbbell hop in the O3 phase shows a large activation energy,
MODIFIED METHODS
Elemental doping
Element substitution can induce a synergistic interaction between phases, consequently stabilizing dual-phase materials and augmenting their electrochemical attributes. To enhance electrochemical performance, various cations, such as Li+, Mg2+, Cu2+, Al3+, Ti4+, and others, are employed to substitute the Mn element in the base material. This substitution modifies physical properties, including the internal configuration of the layered oxide crystal structure, to boost electrochemical efficiency. Thus, element substitution emerges as a viable approach for manipulating the phase composition of NaxMnO2 layered oxides. As a notable example of layered oxide cathodes for SIBs, P2-type Na-FeMn layered oxide (NFMO) achieves high specific discharge capacity but suffers from limited cycling stability, whereas O3-type NFMO offers prolonged cycle life yet at a reduced capacity. To leverage the electrochemical complementarity of these two phases, a unique P2/O3 dual-phase structure of Na0.67Li0.11Fe0.36Mn0.36Ti0.17O2 was synthesized through a substantial
Figure 4. (A) Schematic illustration of the design of the P2/O3 biphasic structure for sodium Fe/Mn-based layered oxide cathode (Reproduced with permission from Ref.[81]. Copyright 2021, Elsevier); (B) HAADF-STEM image of the P3/O′3 composite (Reproduced with permission from Ref.[82]. Copyright 2018, Elsevier); (C) Graphic abstract of the Deciphering the Origin of High Electrochemical Performance in a Novel Ti-Substituted P2/O3 Biphasic Cathode for Sodium-Ion Batteries (Reproduced with permission from Ref.[83]. Copyright 2022, Elsevier); (D) Schematic illustration of phase transition process with increasing Ti content (Reproduced with permission from Ref.[84]. Copyright 2020, American Chemical Society); (E) HRTEM images of the P2 + T material. The amplified figure of the rectangular region (d1-d3) and the corresponding Fast Fourier Transform (FFT) diffraction (d1′-d3′) (Reproduced with permission from Ref.[85]. Copyright 2018, Royal Society of Chemistry).
The substitution also lessens the lattice mismatch between the two phases at elevated potential. While Ti substitution maintains the phase structure intact, it forms a robust Mn-O-Ti-O-Fe bond within the TM layer, bolstering structural stability. However, this comes at the cost of reduced capacity due to electrochemical inactivity of Ti. The research revealed that the effects of Li doping are largely contingent on the site of substitution: Lithium ions occupying the TM site preserve the P2 phase, and during charging, they can migrate to the Na layer, acting as a pillar to fortify cycle stability. Conversely, a substantial introduction of Li+ ions typically leads to a transformation from the P2-type to a more structurally stable O3-type configuration. However, given that Li+ ions are electrochemically inactive, excessive Li substitution can diminish the specific capacity of batteries. Utilizing Li-substituted
Figure 4D demonstrates that the fabricated dual-phase structure mitigates structural strain and significant changes in lattice volume, thereby enhancing its structural robustness under high-pressure conditions.
Through high-temperature calcination, as shown in Figure 5A, Zhang et al. doped Cu elements into Na[Mn0.6Fe0.4]O2 to synthesize O3 dual-phase layered oxides. By adjusting the amount of Cu2+ doping,
Figure 5. (A) Large area HR-TEM image showing the atomic structure of the selected grain (inset SADE pattern). The two regions denoted with A and B have different local crystallographic orientation (Reproduced with permission from Ref.[86]. Copyright 2019, John Wiley and Sons); (B) The schematic diagram of two-phase interface and the HRTEM of Fe0.10-LNM (Reproduced with permission from Ref.[87]. Copyright 2019, John Wiley and Sons); (C) ABF- and HAADF-STEM images of a P2-type Na0.67Mn0.67Ni0.33-xMgxO2 (x = 0) electrode when charging to 4.22 V at the [010] zone axis; The yellow and red rectangles indicate areas with the P2 and O2 structure; ABF- and HAADF-STEM images of a P2-type Na0.67Mn0.67Ni0.33-xMgxO2 (x = 0.05) electrode when charging to 4.22 V (Reproduced with permission from Ref.[88]. Copyright 2016, John Wiley and Sons); (D) Structural evolution of MNM-x with Mg substitution (Reproduced with permission from Ref.[89]. Copyright 2019, John Wiley and Sons); (E) Schematic illustration of M-NCMMT (Reproduced with permission from Ref.[91]. Copyright 2022, Elsevier); (F) HRTEM image at the phase boundary of NLFMTO. Inset is the corresponding FFT map. Red and blue circles are used to mark the reflection spots for P2 and O3 phases, respectively. The scale bar in the FFT map is
Coating two phases
Beyond element doping, the manipulation of interfaces is pivotal for the electrochemical characteristics of materials. Mn-based layered TM oxides often undergo several detrimental alterations, including (i) dissolution of manganese and other active TMs; (ii) surface degradation due to acidic substance erosion in the electrolyte; (iii) a thick Cathode Electrolyte Interface (CEI) layer on the electrode surface after repetitive cycles; (iv) extensive electrolyte consumption; and (v) impediment of Na diffusion. The most straightforward and effective solution to these issues is coating a protective layer on the electrode surface. This coating prevents the direct interaction between the active material and the electrolyte, mitigates acid effects such as HF, and decelerates electrolyte decomposition. Surface coating has been confirmed as a potent method for enhancing the electrochemical performance of SIBs. It achieves this by introducing an artificial second-phase solid electrolyte interface (SEI) film on the electrode while preserving the integrity of the electronic conductive network. To facilitate interface engineering and enhance the electrochemical behavior of layered oxide cathode materials in SIBs, various metal oxides and phosphate coatings (such as Al2O3, CuO, and ZrO2) are employed.
As demonstrated in Figure 6A, Kaliyappan et al. employed atomic layer deposition technology for applying varying thicknesses of Al2O3 on P2-Na2/3(Mn0.54Ni0.13Co0.13)O2[92]. This alteration improved electrochemical performance, with the material discharging 123 mAh g-1 at a current density of 1C and surpassing the original material in cycle stability. Liu et al., as seen in Figure 6B, highlighted that a thin Al2O3 surface coating can effectively curb adverse reactions at high voltages[93]. While oxide materials offer solid protection against electrolyte erosion and boost cycle stability, they are generally non-conductive to Na+ and do not facilitate Na+ diffusion or charge transfer at interfaces. Hence, an ideal coating material should not only protect the electrode but also facilitate sodium ion diffusion through a three-dimensional path, ensuring superior rate performance.
Figure 6. (A) Electrochemical performance between Na2/3(Mn0.54Ni0.13Co0.13)O2 and other cathode materials for sodium-ion batteries (Reproduced with permission from Ref.[92]. Copyright 2019, Elsevier); (B) The structure of Na2SiO3; Schematic illustration of the synthesis process for Na2SiO3@NaNi1/3Mn1/3Fe1/3O2 (Reproduced with permission from Ref.[93]. Copyright 2019, John Wiley and Sons); (C) HRTEM images of NNMO@ZnO sample (Reproduced with permission from Ref.[94]. Copyright 2019, Elsevier); (D) Schematic diagram of MgO-coated NM cathodes (Reproduced with permission from Ref.[95]. Copyright 2022, Frontiers); (E) Microscopic analysis of the Al2O3 coated Na[Ni0.6Co0.2Mn0.2]O2 particle: bright field TEM image (inset image: TEM image of Al2O3 nanoparticles), cross-sectional SEM image, and cross-sectional TEM image (Reproduced with permission from Ref.[96]. Copyright 2013, Royal Society of Chemistry).
As demonstrated in Figure 6C, Li et al. in-situ coated O3-NaNi1/3Mn1/3Fe1/3O2 with sodium ion conductor Na2SiO3, effectively preventing phase transitions[94]. This Na2SiO3 coating reduces polarization, mitigates voltage drop, and supports a larger Na+ diffusion coefficient with its three-dimensional sodium ion diffusion path. As presented in Figure 6D, Xue et al. applied a ZnO coating to P2-type Na2/3[Ni1/3Mn2/3]O2 cathode material using a simple wet chemical method[95]. ZnO serves as a barrier against electrolyte corrosion and particle detachment, enhancing Na+ kinetics during the extraction and insertion processes due to the improved electrode-electrolyte interface. Zn2+ replaces the TM layer, shortens the metal-oxygen bond length, and reinforces the material's structural stability, collectively boosting the electrochemical performance of Na2/3[Ni1/3Mn2/3]O2, with the ZnO-coated cathode showing robust capacity retention.
As displayed in Figure 6E, Hwang et al. enhanced the cycle performance of Na0.67Ni0.33Mn0.67O2 cathode material by coating it with MgO[96]. They successfully applied varying thicknesses of MgO, which exhibited very low charge transfer resistance. At a rate of 0.2 °C and in the voltage range of 2.0-4.5 V, the initial reversible discharge capacity reached 105 mAh g-1, with a cycle retention rate as high as 81.5%. Hwang et al. synthesized Al2O3 nanoparticle-coated O3-Na[Ni0.6Co0.2Mn0.2]O2 cathodes using a simple dry ball milling method, effectively reducing parasitic reactions in the electrolyte solution and enhancing Na+ migration. The modified material reveals a specific capacity of 151 mAh g-1 and outstanding cycle stability and rate capability.
Figure 7A illustrates Yong-Mook Kang's synthesis of layered oxide cathodes with multiple phases, whose characteristics shift based on the sodium ion content, significantly influencing their electrochemical performance[97]. The nonstoichiometric nature of these layered oxides with sodium ions affects not only their capacity but also their cycle stability and kinetics, underscoring the critical role of sodium ion content. However, Na2CO3 is prone to irreversible formation on the surface due to the loss of sodium ions from the lattice, leading to detrimental effects when the stoichiometric Na-containing O3 phase transitions to a non-stoichiometric P2 phase. Addressing this issue involves decomposing Na2CO3 into electrochemically active sodium ions while transforming the non-stoichiometric P2 phase back to a stoichiometric O3. The study emphasizes that minimizing sodium ion loss and maintaining a lattice structure rich in sodium ions are crucial for achieving electrochemical benefits in developing sodium-containing layered cathodes.
Figure 7. (A) In-situ XRD patterns and corresponding charge/discharge curves of 1st cycle measured at a C-rate of 0.25 C of pristine NMO and activated NMO (Reproduced with permission from Ref.[97]. Copyright 2021, John Wiley and Sons); (B) Schematic illustration of the surficial evolutions of Na0.67Zn0.1Mn0.9O2 and Al2O3@Na0.67Zn0.1Mn0.9O2 electrodes (Reproduced with permission from Ref.[98]. Copyright 2020, Elsevier).
Element doping and surface engineering can synergistically enhance the structural stability of materials in some cases. As depicted in Figure 7B, Zuo et al. engineered an Al2O3@Na0.67Zn0.1Mn0.9O2 coating through Zn2+ substitution and Al2O3 atomic layer deposition. This design capitalizes on the benefits of structural stability and surface passivation to mitigate related challenges[98]. Additionally, the uniform and resilient CEI layer formed on the Al2O3-coated electrode effectively blocks further decomposition of the organic electrolyte, enhancing the material's overall performance.
Other strategies
Sodium layered oxides often exhibit instability in deep desorption within the P2 structure and sluggish kinetics in the O3 structure. Hence, developing dual-phase materials that merge the benefits of both P2 and O3 structures is advantageous. Beyond the previously discussed strategies of element substitution and surface coating, additional methods exist for fabricating two-phase layered oxide materials. Figure 8A shows that Xiao et al. precisely manipulated structural evolution and created high-performance heterostructured dual-phase layered oxide cathodes through local chemical and orbital hybridization modulation. They employed macroscopic and atomic-scale techniques to vividly depict the layered P2 and O3-type heterostructures[99]. Owing to the synergistic advantages of symbiotic structure and local environment adjustment, local chemical modulation is utilized to foster energy and crystal structure evolution, forming layered symbiotic oxide cathodes.
Figure 8. (A) Intensity contour maps (bird’s eye view) showing the evolution of the main characteristic diffraction peaks; Schematic illustration of the crystal structural evolution during the cycling (Reproduced with permission from Ref.[99]. Copyright 2022, John Wiley and Sons); (B) Contour plots of in-situ XRD patterns at various temperatures, and evolution of lattice parameters upon Na+ extraction (Reproduced with permission from Ref.[80]. Copyright 2023, Elsevier); (C) Operando XRD patterns during the charge/discharge process in the voltage range of 1.5-4 V (vs. Na+/Na). Black asterisks represent peaks from Al window; Corresponding intensity contour maps (bird’s eye view) concerning the evolution of the main characteristic diffraction peaks (Reproduced with permission from Ref.[100]. Copyright 2021, John Wiley and Sons); (D) In-situ synchrotron-based XRD patterns collected during the first cycle at 0.1 C, with the corresponding charge/discharge curve on the right; Intensity contour map and corresponding three-dimensional (3D) maps (Reproduced with permission from Ref.[61]. Copyright 2020, John Wiley and Sons).
Figure 8B highlights that Zhou et al. put forward Na0.7Mn0.4Ni0.3Cu0.1Fe0.1Ti0.1O1.95F0.1 with a P2/O3 dual-phase structure as a novel high-entropy cathode material[80]. This composition integrates various elemental benefits, with Mn and Ni elements as primary redox species, Fe element mitigating the Jahn-Teller effect of Mn, Cu element enhancing Na+ mobility and air stability, Ti element preventing phase transitions, and F element inducing cation redistribution. The formation of the P2/O3 dual phase during synthesis was examined through high-temperature in situ XRD and electron microscopy. As shown in Figure 8C,
RESULTS AND PROSPECTS
The development of advanced characterization techniques has led to a growing understanding of layered oxide materials for SIBs. From synthesis methods to structural changes, the subtle changes of this promising cathode material during charge and discharge are revealed. However, sodium ion layered oxides still have limitations. For this reason, the design of two or more non-interacting phases will produce electrodes with intermediate phases but excellent properties, and further, multiphase materials can provide better performance than expected from simple mixtures. It is necessary to investigate the recent phase transition of layered oxide two-phase materials in the sodium ion insertion and deinsertion reaction. Exploring the core mechanisms underlying the evolution of layered dual-phase cathode materials during their charging and discharging cycles continues to be a crucial area for future research. Delving into these mechanisms is challenging, yet the amalgamation of advanced characterization techniques, offering higher precision and real-time micro-level observation, brings us closer to understanding the most authentic reaction processes. The emergence of various sophisticated in-situ characterization methods, such as in-situ X-ray, optical, and electron imaging technologies, forms a vital foundation for the detailed examination of these micro-mechanisms.
Currently, research on dual-phase cathode materials, particularly those enhanced through surface coating and elemental doping, has achieved significant strides in numerous aspects. Although element doping can improve the structural stability of the material, it will lose part of its capacity. Coating two phases can effectively improve the side reaction between the electrode and the electrolyte interface, inhibiting the Jahn-teller effect, but achieving a complete and uniform coating has become a research difficulty. Large-scale preparation of other modification schemes has not been fully achieved. Hence, it is important to recognize that a singular approach often addresses only specific issues and brings about limited enhancements, thereby falling short of fulfilling multiple simultaneous requirements. For real-world applications, cathode materials need to surmount a range of challenges. Consequently, a holistic approach that integrates and applies various modification techniques is necessary to address these challenges comprehensively and enhance the overall efficacy of layered oxide cathode materials for SIBs. Based on the existing research, the multiphase Mn-based layered oxide cathode materials for SIBs can be further optimized from the following ideas in the future [Figure 9].
Figure 9. Summary of the negative effects, suppression strategies, and prospects for future high-performance SIBs in multiphase manganese-based layered oxides.
Firstly, solid-phase, ball milling, sol-gel, and hydrothermal methods can be used to synthesize multiphase Mn-based layered oxides, but their large-scale production remains challenging. Hence, developing suitable and effective synthesis schemes is the premise of designing and constructing high-performance multiphase Mn-based layered oxide materials. A suitable synthesis scheme can prevent the H2O/CO2 in the atmospheric environment from being easily embedded into the interlayer during the synthesis, resulting in structural damage and improving electrochemical performance. At the same time, the appropriate scheme can also improve the sodium deficiency state of the multiphase Mn-based layered oxide, which can increase the energy density and promote the sodium storage ability and commercial value of Mn-based layered TM oxide cathodes.
In addition, it is necessary to develop a stable high-voltage electrolyte for multiphase Mn-based layered oxides. For most layered TM oxide cathodes, the theoretical operating voltage window can usually reach over 4.0 V. However, carbonate ester-based electrolytes usually undergo severe decomposition in the high-pressure range. Therefore, to obtain high energy density, the development of stable high-voltage electrolytes is the key to realizing the high energy density advantage of SIBs. The formation and characteristics of the SEI on the anode and the positive electrolyte interface (CEI) on the cathode play an important role in the design and matching of SIBs. Therefore, developing functional additives to improve the performance of the electrolyte and promote the formation of a stable CEI film on the electrode will become a research hotspot and a difficulty.
Furthermore, a single strategy usually only solves specific problems and achieves limited improvement, making it difficult to meet multiple needs. For practical applications, cathode materials face diverse challenges. The organic combination of various modification methods and the integration of their respective advantages is the general trend in future research and development of layered oxide cathodes. As more advanced characterization methods are developed, we can better understand the reaction mechanism and structure-activity relationship of materials. They are also vital to achieving greater breakthroughs in the field of layered oxide cathodes. Therefore, a comprehensive application and integration strategy of various modification methods should be developed to solve multiple problems and improve the overall performance of multiphase Mn-based layered oxide cathode materials for SIBs.
Finally, despite the rapid development of layered oxide cathodes for SIBs, most current research of multiphase Mn-based layered oxides is based on the performance and mechanism tests of half-cell systems. Sodium reacts at high voltage, forming a thick and unstable surface layer. As a reference electrode, it is highly dependent on the electrolyte and atmospheric composition, which produces an unstable potential and leads to an inaccurate evaluation of the cathode material. This seriously affects rate and voltage measurements. Therefore, to realize the practical application of SIBs, it is necessary to find a reference electrode with stable static potential and chemical inertness that works stably in the electrochemical window with the most commonly used electrolyte to achieve a comprehensive evaluation of layered oxide batteries' performance and finally achieve high-performance sodium ion full battery devices.
DECLARATIONS
Authors' Contributions
Developed the concept: Luo WB, Liu Z
Conducted the frame of the paper: Lai Q, Gao XW
Gathered the initial characterizations and data analysis: Liu Z, Song Y
Collected the various works of literature: An P, Dong M, Wang S
Written the manuscript, helped to polish this paper: Fu S, Luo WB
All authors discussed the results and commented on the manuscript.
Availability of Data and Materials
Not applicable.
Financial support and sponsorship
The work was financially supported by the National Natural Science Foundation of China (No. 52204308 and No. 52272194), the LiaoNing Revitalization Talents Program (Grant No. XLYC2007155), the Natural Science Foundation of Liaoning Province (2023-MSBA-101), the Postdoctoral Science Foundation of China (No. ZX20220158), and the Postdoctoral Foundation of Northeastern University.
Conflicts of interest
All authors declared that there are no conflicts of interest.
Ethical approval and consent to participate
Not applicable.
Consent for publication
Not applicable.
Copyright
© The Author(s) 2024.
REFERENCES
1. Harper G, Sommerville R, Kendrick E, et al. Recycling lithium-ion batteries from electric vehicles. Nature 2019;575:75-86.
2. Liu H, Zhu Z, Yan Q, et al. A disordered rock salt anode for fast-charging lithium-ion batteries. Nature 2020;585:63-7.
3. Zhou Y, Su M, Yu X, et al. Real-time mass spectrometric characterization of the solid-electrolyte interphase of a lithium-ion battery. Nat Nanotechnol 2020;15:224-30.
4. Wang J, Fan L, Liu Z, et al. In situ alloying strategy for exceptional potassium ion batteries. ACS Nano 2019;13:3703-13.
5. Ge J, Fan L, Wang J, et al. MoSe2/N-doped carbon as anodes for potassium-ion batteries. Adv Energy Mater 2018;8:1801477.
6. Mu J, Zhao Z, Gao X, et al. Bimetallic PdFe3 nano-alloy with tunable electron configuration for boosting electrochemical nitrogen fixation. Adv Energy Mater 2023;14:2303558.
7. Zhang Q, Wang L, Wang J, et al. Low-temperature synthesis of edge-rich graphene paper for high-performance aluminum batteries. Energy Stor Mater 2018;15:361-7.
8. Zhao L, Gao X, Gu Q, et al. Realizing a dendrite-free metallic-potassium anode using reactive prewetting chemistry. eScience 2024;4:100201.
9. Hu Z, Hao J, Shen D, et al. Electro-spraying/spinning: a novel battery manufacturing technology. Green Energy Environ 2024;9:81-8.
10. Yu W, Ge J, Hu Y, et al. Hybrid high-performance aqueous batteries with potassium-based cathode||zinc metal anode. Sci China Mater 2023;66:923-31.
11. Wang J, Wang B, Lu B. Nature of novel 2D van der Waals heterostructures for superior potassium ion batteries. Adv Energy Mater 2020;10:2000884.
12. Li L, Liu L, Hu Z, et al. Understanding high-rate K+-solvent co-intercalation in natural graphite for potassium-ion batteries. Angew Chem Int Ed 2020;59:12917-24.
13. Wang L, Menakath A, Han F, et al. Identifying the components of the solid-electrolyte interphase in Li-ion batteries. Nat Chem 2019;11:789-96.
14. Mackanic DG, Yan X, Zhang Q, et al. Decoupling of mechanical properties and ionic conductivity in supramolecular lithium ion conductors. Nat Commun 2019;10:5384.
16. Wang J, Zhang G, Liu Z, et al. Li3V(MoO4)3 as a novel electrode material with good lithium storage properties and improved initial coulombic efficiency. Nano Energy 2018;44:272-8.
17. Liu Z, Wang J, Ding H, Chen S, Yu X, Lu B. Carbon nanoscrolls for aluminum battery. ACS Nano 2018;12:8456-66.
18. Liu Z, Wang J, Jia X, et al. Graphene armored with a crystal carbon shell for ultrahigh-performance potassium ion batteries and aluminum batteries. ACS Nano 2019;13:10631-42.
19. Zhao L, Gao X, Mu J, et al. Durable integrated K-metal anode with enhanced mass transport through potassiphilic porous interconnected mediator. Adv Funct Mater 2023;33:2304292.
20. Liu Z, Wang J, Lu B. Plum pudding model inspired KVPO4F@3DC as high-voltage and hyperstable cathode for potassium ion batteries. Sci Bull 2020;65:1242-51.
21. Guo YJ, Wang PF, Niu YB, et al. Boron-doped sodium layered oxide for reversible oxygen redox reaction in Na-ion battery cathodes. Nat Commun 2021;12:5267.
22. Ji H, Ji W, Xue H, et al. Synergistic activation of anionic redox via cosubstitution to construct high-capacity layered oxide cathode materials for sodium-ion batteries. Sci Bull 2023;68:65-76.
23. Huang Z, Zhang X, Zhao X, et al. Hollow Na0.62K0.05Mn0.7Ni0.2Co0.1O2 polyhedra with exposed stable {001} facets and K riveting for sodium-ion batteries. Sci China Mater 2023;66:79-87.
24. Liu Q, Hu Z, Chen M, et al. Recent progress of layered transition metal oxide cathodes for sodium-ion batteries. Small 2019;15:e1805381.
25. Shi Q, Qi R, Feng X, et al. Niobium-doped layered cathode material for high-power and low-temperature sodium-ion batteries. Nat Commun 2022;13:3205.
26. Wang C, Liu L, Zhao S, et al. Tuning local chemistry of P2 layered-oxide cathode for high energy and long cycles of sodium-ion battery. Nat Commun 2021;12:2256.
27. Wang F, Zhang J, Lu H, et al. Production of gas-releasing electrolyte-replenishing Ah-scale zinc metal pouch cells with aqueous gel electrolyte. Nat Commun 2023;14:4211.
28. Xu GL, Liu X, Zhou X, et al. Native lattice strain induced structural earthquake in sodium layered oxide cathodes. Nat Commun 2022;13:436.
29. Deng T, Ji X, Zou L, et al. Interfacial-engineering-enabled practical low-temperature sodium metal battery. Nat Nanotechnol 2022;17:269-77.
31. Zuo W, Innocenti A, Zarrabeitia M, Bresser D, Yang Y, Passerini S. Layered oxide cathodes for sodium-ion batteries: storage mechanism, electrochemistry, and techno-economics. ACC Chem Res 2023;56:284-96.
32. Cao X, Li H, Qiao Y, et al. Stabilizing reversible oxygen redox chemistry in layered oxides for sodium-ion batteries. Adv Energy Mater 2020;10:1903785.
33. Hu Z, Geng C, Wang L, Lv W, Yang Q. Revisiting the roles of carbon in the catalysis of lithium-sulfur batteries. Adv Energy Sustain Res 2024;5:2300148.
34. Xiao Y, Abbasi NM, Zhu Y, et al. Layered oxide cathodes promoted by structure modulation technology for sodium-ion batteries. Adv Funct Mater 2020;30:2001334.
35. Hu HY, Wang H, Zhu YF, et al. A universal strategy based on bridging microstructure engineering and local electronic structure manipulation for high-performance sodium layered oxide cathodes. ACS Nano 2023;17:15871-82.
36. Liu S, Wan J, Ou M, et al. Regulating Na occupation in P2-type layered oxide cathode for all-climate sodium-ion batteries. Adv Energy Mater 2023;13:2203521.
37. Shen L, Wang Y, Lv H, et al. Ultrathin Ti2Nb2O9 nanosheets with pseudocapacitive properties as superior anode for sodium-ion batteries. Adv Mater 2018;30:e1804378.
38. Chu S, Kim D, Choi G, et al. Revealing the origin of transition-metal migration in layered sodium-ion battery cathodes: random Na extraction and Na-free layer formation. Angew Chem Int Ed 2023;62:e202216174.
39. Wang P, You Y, Yin Y, Guo Y. Layered oxide cathodes for sodium-ion batteries: phase transition, air stability, and performance. Adv Energy Mater 2018;8:1701912.
40. Xiao Y, Wang P, Yin Y, et al. A layered-tunnel intergrowth structure for high-performance sodium-ion oxide cathode. Adv Energy Mater 2018;8:1800492.
41. Zhao C, Ding F, Lu Y, Chen L, Hu YS. High-entropy layered oxide cathodes for sodium-ion batteries. Angew Chem Int Ed 2020;59:264-9.
42. Huang Q, Wang M, Zhang L, et al. Shear-resistant interface of layered oxide cathodes for sodium ion batteries. Energy Stor Mater 2022;45:389-98.
43. Lin C, Dai P, Wang X, et al. P2/O3 biphase integration promoting the enhancement of structural stability for sodium layered oxide cathode. Chem Eng J 2024;480:147964.
44. Mu J, Cai T, Dong W, Zhou C, Han Z, Huang F. Biphasic high-entropy layered oxide as a stable and high-rate cathode for sodium-ion batteries. Chem Eng J 2023;471:144403.
45. Wang SS, Liu ZM, Gao XW, Wang XC, Chen H, Luo WB. Layer-structured multitransition-metal oxide cathode materials for potassium-ion batteries with long cycling lifespan and superior rate capability. ACS Appl Mater Interfaces 2023;15:57165-73.
46. Mu J, Gao X, Liu Z, et al. Boosting nitrogen electrocatalytic fixation by three-dimensional TiO2-N nanowire arrays. J Energy Chem 2022;75:293-300.
47. Wang D, Liu Z, Gao X, Gu Q, Zhao L, Luo W. Massive anionic fluorine substitution two-dimensional δ-MnO2 nanosheets for high-performance aqueous zinc-ion battery. J Energy Stor 2023;72:108740.
48. Li J, Mu J, Liu Z, et al. Boosting potassium-based dual ion battery with high energy density and long lifespan by red phosphorous. J Power Sources 2023;571:233054.
49. Han MH, Gonzalo E, Singh G, Rojo T. A comprehensive review of sodium layered oxides: powerful cathodes for Na-ion batteries. Energy Environ Sci 2015;8:81-102.
50. Lin C, Liu H, Kang J, et al. In-situ X-ray studies of high-entropy layered oxide cathode for sodium-ion batteries. Energy Stor Mater 2022;51:159-71.
51. Zhang G, Li J, Fan Y, et al. Suppressed P2-P2′ phase transition of Fe/Mn-based layered oxide cathode for high-performance sodium-ion batteries. Energy Stor Mater 2022;51:559-67.
52. Yu Y, Ning D, Li Q, et al. Revealing the anionic redox chemistry in O3-type layered oxide cathode for sodium-ion batteries. Energy Stor Mater 2021;38:130-40.
53. Zhong L, Qiu X, Yang S, Sun S, Chen L, Zhang W. Supermolecule-regulated synthesis strategy of general biomass-derived highly nitrogen-doped carbons toward potassium-ion hybrid capacitors with enhanced performances. Energy Stor Mater 2023;61:102887.
54. Shi C, Wang L, Chen X, et al. Challenges of layer-structured cathodes for sodium-ion batteries. Nanoscale Horiz 2022;7:338-51.
55. Katcho NA, Carrasco J, Saurel D, et al. Origins of bistability and Na ion mobility difference in P2- and O3-Na2/3Fe2/3Mn1/3O2 cathode polymorphs. Adv Energy Mater 2017;7:1601477.
56. Lee E, Lu J, Ren Y, et al. Layered P2/O3 intergrowth cathode: toward high power Na-ion batteries. Adv Energy Mater 2014;4:1400458.
57. Zhao W, Tsuchiya Y, Yabuuchi N. Influence of synthesis conditions on electrochemical properties of P2-type Na2/3Fe2/3Mn1/3O2 for rechargeable Na batteries. Small Methods 2019;3:1800032.
58. Cao Y, Zhang Q, Wei Y, et al. A water stable, near-zero-strain O3-layered titanium-based anode for long cycle sodium-ion battery. Adv Funct Mater 2020;30:1907023.
59. Yang L, del Amo JML, Shadike Z, et al. A Co- and Ni-Free P2/O3 biphasic lithium stabilized layered oxide for sodium-ion batteries and its cycling behavior. Adv Funct Mater 2020;30:2003364.
60. Zhao C, Avdeev M, Chen L, Hu YS. An O3-type oxide with low sodium content as the phase-transition-free anode for sodium-ion batteries. Angew Chem Int Ed 2018;57:7056-60.
61. Zhu YF, Xiao Y, Hua WB, et al. Manipulating layered P2@P3 integrated spinel structure evolution for high-performance sodium-ion batteries. Angew Chem Int Ed 2020;59:9299-304.
62. Liu Z, Zhou C, Liu J, Yang L, Liu J, Zhu M. Phase tuning of P2/O3-type layered oxide cathode for sodium ion batteries via a simple Li/F co-doping route. Chem Eng J 2022;431:134273.
63. Maughan PA, Naden AB, Irvine JTS, Armstrong AR. Manipulating O3/P2 phase ratio in bi-phasic sodium layered oxides via ionic radius control. Commun Mater 2023;4:6.
64. Li R, Liu Y, Wang Z, Li J. A P2/O3 biphasic cathode material with highly reversibility synthesized by Sn-substitution for Na-ion batteries. Electrochim Acta 2019;318:14-22.
65. Huang J, Li W, Ye D, Xu L, Wu W, Wu X. Designing ultrastable P2/O3-type layered oxides for sodium ion batteries by regulating Na distribution and oxygen redox chemistry. J Energy Chem 2024;94:466-76.
66. Zhang L, Guan C, Zheng J, et al. Rational design of intergrowth P2/O3 biphasic layered structure with reversible anionic redox chemistry and structural evolution for Na-ions batteries. Sci Bull 2023;68:180-91.
67. Sharma N, Bahri OKA, Han MH, Gonzalo E, Pramudita JC, Rojo T. Comparison of the structural evolution of the O3 and P2 phases of Na2/3Fe2/3Mn1/3O2 during electrochemical cycling. Electrochim Acta 2016;203:189-97.
68. Xu G, Amine R, Xu Y, et al. Insights into the structural effects of layered cathode materials for high voltage sodium-ion batteries. Energy Environ Sci 2017;10:1677-93.
69. Zhou D, Huang W, Lv X, Zhao F. A novel P2/O3 biphase Na0.67Fe0.425Mn0.425Mg0.15O2 as cathode for high-performance sodium-ion batteries. J Power Sources 2019;421:147-55.
70. Zhao J, Zhang X, Wang J, Yang X, Deng J, Wang Y. P2-type Na0.59Co0.20Mn0.77Mo0.03O2 cathode with excellent cycle stability for sodium-ion batteries. J Solid State Electrochem 2020;24:1349-61.
71. Bianchini M, Wang J, Clément RJ, et al. The interplay between thermodynamics and kinetics in the solid-state synthesis of layered oxides. Nat Mater 2020;19:1088-95.
72. Wang JE, Kim H, Jung YH, Kim DK, Kim DJ. Designing high energy sodium-ion battery cathodes by utilizing P2/O3 biphasic structure and lithium honeycomb ordering. Small 2021;17:e2100146.
73. Guo S, Liu P, Yu H, et al. A layered P2- and O3-type composite as a high-energy cathode for rechargeable sodium-ion batteries. Angew Chem Int Ed 2015;54:5894-9.
74. Qi X, Liu L, Song N, et al. Design and comparative study of O3/P2 hybrid structures for room temperature sodium-ion batteries. ACS Appl Mater Interfaces 2017;9:40215-23.
75. Zhou D, Zeng C, Ling D, et al. Sustainable alternative cathodes of sodium-ion batteries using hybrid P2/O3 phase
76. Zhang P, Zhang G, Liu Y, et al. Constructing P2/O3 biphasic structure of Fe/Mn-based layered oxide cathode for high-performance sodium-ion batteries. J Colloid Interface Sci 2024;654:1405-16.
77. Wang K, Wu Z, Melinte G, et al. Preparation of intergrown P/O-type biphasic layered oxides as high-performance cathodes for sodium ion batteries. J Mater Chem A 2021;9:13151-60.
78. Hong J, Xiao S, Deng L, Lan T, He G. Li-free P2/O3 biphasic Na0.73Ni0.4Mn0.4Ti0.2O2 as a cathode material for sodium-ion batteries. Ionics 2020;26:3911-7.
79. Xu W, Gao X, Zhou Y, Zou G, Hou H, Ji X. Sodium de-insertion processes in single Na TMO2 particles studied by an electrochemical collision method: O3 phases versus P2 phases. Electrochem Commun 2021;125:107000.
80. Zhou P, Che Z, Liu J, et al. High-entropy P2/O3 biphasic cathode materials for wide-temperature rechargeable sodium-ion batteries. Energy Stor Mater 2023;57:618-27.
81. Chen C, Huang W, Li Y, et al. P2/O3 biphasic Fe/Mn-based layered oxide cathode with ultrahigh capacity and great cyclability for sodium ion batteries. Nano Energy 2021;90:106504.
82. Huang Q, Liu J, Xu S, et al. Roles of coherent interfaces on electrochemical performance of sodium layered oxide cathodes. Chem Mater 2018;30:4728-37.
83. Yu L, Cheng Z, Xu K, et al. Interlocking biphasic chemistry for high-voltage P2/O3 sodium layered oxide cathode. Energy Stor Mater 2022;50:730-9.
84. Hu B, Geng F, Zhao C, et al. Deciphering the origin of high electrochemical performance in a novel Ti-substituted P2/O3 biphasic cathode for sodium-ion batteries. ACS Appl Mater Interfaces 2020;12:41485-94.
85. Gao G, Tie D, Ma H, et al. Interface-rich mixed P2 + T phase NaxCo0.1Mn0.9O2 (0.44 ≤ x ≤ 0.7) toward fast and high capacity sodium storage. J Mater Chem A 2018;6:6675-84.
86. Zhang Z, Liu Y, Liu Z, et al. Dual-strategy of Cu-doping and O3 biphasic structure enables Fe/Mn-based layered oxide for high-performance sodium-ion batteries cathode. J Power Sources 2023;567:232930.
87. Feng J, Fang D, Yang Z, et al. A novel P2/O3 composite cathode toward synergistic electrochemical optimization for sodium ion batteries. J Power Sources 2023;553:232292.
88. Wang P, You Y, Yin Y, et al. Suppressing the P2-O2 Phase Transition of Na0.67Mn0.67Ni0.33O2 by magnesium substitution for improved sodium-ion batteries. Angew Chem Int Ed 2016;128:7571-5.
89. Wang QC, Meng JK, Yue XY, et al. Tuning P2-structured cathode material by Na-site Mg substitution for Na-ion batteries. J Am Chem Soc 2019;141:840-8.
90. Tao S, Zhou W, Wu D, et al. Insights into the Ti4+ doping in P2-type Na0.67Ni0.33Mn0.52Ti0.15O2 for enhanced performance of sodium-ion batteries. J Mater Sci Technol 2021;74:230-6.
91. Wang JZ, Teng YX, Su GQ, Bao S, Lu JL. A dual-modification strategy for P2-type layered oxide via bulk Mg/Ti co-substitution and MgO surface coating for sodium ion batteries. J Colloid Interface Sci 2022;608:3013-21.
92. Kaliyappan K, Liu J, Lushington A, Li R, Sun X. Highly stable Na2/3(Mn0.54Ni0.13Co0.13)O2 cathode modified by atomic layer deposition for sodium-ion batteries. ChemSusChem 2015;8:2537-43.
93. Liu Y, Fang X, Zhang A, et al. Layered P2-Na2/3[Ni1/3Mn2/3]O2 as high-voltage cathode for sodium-ion batteries: the capacity decay mechanism and Al2O3 surface modification. Nano Energy 2016;27:27-34.
94. Li N, Ren J, Dang R, et al. Suppressing phase transition and improving electrochemical performances of O3-NaNi1/3Mn1/3Fe1/3O2 through ionic conductive Na2SiO3 coating. J Power Sources 2019;429:38-45.
95. Xue L, Bao S, Yan L, Zhang Y, Lu J, Yin Y. MgO-coated layered cathode oxide with enhanced stability for sodium-ion batteries. Front Energy Res 2022;10:847818.
96. Hwang J, Myung S, Choi JU, Yoon CS, Yashiro H, Sun Y. Resolving the degradation pathways of the O3-type layered oxide cathode surface through the nano-scale aluminum oxide coating for high-energy density sodium-ion batteries. J Mater Chem A 2017;5:23671-80.
97. Yang J, Lim J, Park M, et al. Thermally activated P2-O3 mixed layered cathodes toward synergistic electrochemical enhancement for Na ion batteries. Adv Energy Mater 2021;11:2102444.
98. Zuo W, Qiu J, Liu X, et al. Highly-stable P2-Na0.67MnO2 electrode enabled by lattice tailoring and surface engineering. Energy Stor Mater 2020;26:503-12.
99. Xiao Y, Wang HR, Hu HY, et al. Formulating high-rate and long-cycle heterostructured layered oxide cathodes by local chemistry and orbital hybridization modulation for sodium-ion batteries. Adv Mater 2022;34:e2202695.
Cite This Article
Export citation file: BibTeX | EndNote | RIS
OAE Style
Liu Z, Song Y, Fu S, An P, Dong M, Wang S, Lai Q, Gao XW, Luo WB. Multiphase manganese-based layered oxide for sodium-ion batteries: structural change and phase transition. Microstructures 2024;4:2024036. http://dx.doi.org/10.20517/microstructures.2024.01
AMA Style
Liu Z, Song Y, Fu S, An P, Dong M, Wang S, Lai Q, Gao XW, Luo WB. Multiphase manganese-based layered oxide for sodium-ion batteries: structural change and phase transition. Microstructures. 2024; 4(3): 2024036. http://dx.doi.org/10.20517/microstructures.2024.01
Chicago/Turabian Style
Zhaomeng Liu, Yingying Song, Shizheng Fu, Pengyan An, Mohan Dong, Shuran Wang, Qingsong Lai, Xuan-Wen Gao, Wen-Bin Luo. 2024. "Multiphase manganese-based layered oxide for sodium-ion batteries: structural change and phase transition" Microstructures. 4, no.3: 2024036. http://dx.doi.org/10.20517/microstructures.2024.01
ACS Style
Liu, Z.; Song Y.; Fu S.; An P.; Dong M.; Wang S.; Lai Q.; Gao X.W.; Luo W.B. Multiphase manganese-based layered oxide for sodium-ion batteries: structural change and phase transition. Microstructures. 2024, 4, 2024036. http://dx.doi.org/10.20517/microstructures.2024.01
About This Article
Special Issue
Copyright
Data & Comments
Data
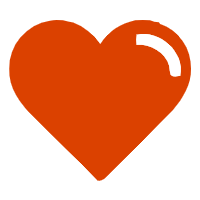
Comments
Comments must be written in English. Spam, offensive content, impersonation, and private information will not be permitted. If any comment is reported and identified as inappropriate content by OAE staff, the comment will be removed without notice. If you have any queries or need any help, please contact us at support@oaepublish.com.