Recent advances in carbon-based sulfur host materials for lithium-sulfur batteries
Abstract
Lithium-sulfur batteries (LSBs) have been brought into focus as the development direction of the next-generation power battery system due to their high energy density, eco-friendliness, and low cost, which has a broad application prospect in the field of energy storage. However, some problems are still unresolved in the sulfur cathode, e.g., poor electric conductivity, serious volume expansion of sulfur, shuttle effect caused by easy dissolution of lithium polysulfides in the electrolyte, and slow redox reaction kinetics of sulfur species. These issues lead to poor cycle stability and rate performance, making it hard to meet the requirement for LSBs in practical applications. Since the inherent nature of sulfur is the root cause of the above problems, reasonable design of functional sulfur hosts will be an effective way to break through the current bottlenecks of LSBs. The review covers the latest research progress on carbon-based sulfur host materials of LSBs, including structural design and functional optimization strategies, aiming to prepare multifunctional sulfur host materials by integrating physical confinement, chemical adsorption, and catalytic effect towards lithium polysulfides. The obstacles and future prospects of carbon-based sulfur hosts have also been brought forward, which provides in-depth guidance for practical application of LSBs.
Keywords
INTRODUCTION
The development of efficient, safe, and environmentally friendly energy storage systems is an enabler to improve the energy structure and promote low-carbon development and can effectively solve the contradiction between the continuity of energy demand and the discontinuity of renewable energy supply[1,2]. Among various energy storage systems, lithium-ion batteries (LIBs) consisting of LiCoO2 cathodes and graphite anodes were successfully commercialized in 1991[3]. Due to their high discharge specific capacity, lightweight, long life, and high safety, LIBs have been widely used in energy applications, such as portable electronic devices, electric vehicles (EVs), and hybrid EVs (HEVs)[4,5]. However, with the further growth of energy demand, LIBs based on graphite anodes (theoretical specific capacity of
Among the current energy storage systems, lithium-sulfur batteries (LSBs) consisting of sulfur cathodes and lithium metal anodes are attracting attention and developing very rapidly[9,10]. Unlike the intercalation and de-intercalation mechanism of Li+ in conventional LIBs, the energy conversion mechanism of LSBs is a reaction of S8 + 16Li → 8Li2S. Due to high theoretical energy density (2,600 Wh kg-1) and specific capacity of sulfur cathodes (1,675 mAh g-1), LSBs are considered as one of the directions for next-generation power battery systems[11,12]. Additionally, low toxicity and abundant resources of sulfur are very attractive in terms of mass production and sustainable development. If LSBs can be successfully commercialized, a battery pack with an actual energy density of 400-600 Wh kg-1 can significantly improve the cruising ability and effectively alleviate the problem of “mileage anxiety” of EVs[13,14].
In this review, as shown in Figure 1, a comprehensive overview of the latest research progress on carbon-based sulfur host materials of LSBs is provided, aiming to prepare multifunctional sulfur host materials by integrating physical confinement, chemical adsorption, and catalytic effect towards lithium polysulfides (LiPSs). The review mainly includes four aspects: (1) The latest development status and the reaction mechanism of LSBs; (2) Remaining problems of sulfur cathodes hindering the development of LSBs; (3) The research progress of carbon-based sulfur host materials in improving the electrochemical performance of LSBs; and (4) The future development direction of carbon-based sulfur host materials for LSBs. This review aims to inspire future research and development of innovative sulfur host materials by providing clear and concise information to ultimately achieve efficient sulfur cathodes.
Mechanism and challenges of LSBs
The configuration and operating mechanism of LSBs
LSBs are a class of secondary batteries that use the breaking and generation of sulfur-sulfur bonds to achieve the mutual conversion of chemical and electrical energy by applying sulfur or sulfur-containing compounds as the cathode and lithium or lithium-containing materials as the anode[15]. The structure of LSBs is schematically shown in Figure 2A, which mainly consists of four parts: a sulfur cathode, separators, electrolytes, and lithium metal anodes[16]. Among them, the sulfur cathode is usually composed of elemental sulfur (S8), conductive carbon, and binders. For the typical “solid-liquid-solid” reaction mechanism of LSBs, ether-based electrolytes are usually used because the carbonate-based electrolyte will nucleophilically react with LiPS[17,18]. The formation of LiPS can be avoided by changing the “solid-liquid-solid” to a “solid-solid” reaction mechanism, thus making carbonate electrolytes possible[19,20].
Figure 2. (A) Schematic diagram and (B) charge-discharge curves of LSBs[16]. Copyright Elsevier 2020.
A typical charge/discharge curve of an LSB in an ether electrolyte is shown in Figure 2B. During the discharge process, two voltage plateaus are typically observed, 2.1-2.4 and 1.6-2.1 V, respectively. In general, the first plateau is 2.1-2.4 V, corresponding to the conversion of S8 to soluble Li2Sx (6 ≤ x ≤ 8), which provides a specific capacity of 419 mA h g-1, which accounts for 25% of theoretical capacity. The second plateau occurs at 1.6~2.1 V, mainly corresponding to the transformation of Li2Sx to short-chain Li2S4 and insoluble Li2S2/Li2S, providing a specific capacity of about 1,256 mA h g-1, which accounts for 75% of theoretical capacity[21]. During the charging process, insoluble Li2S/Li2S2 is transformed into LiPSs and eventually converted to elemental sulfur[22]. Recently, Zhou et al. observed directly the transformation of LiPSs on the electrode surface at the atomic scale by using in situ liquid-cell electrochemical transmission electron microscopy[23]. The introduction of surficial active centers of metal nanoclusters induces LiPSs aggregation and charge storage, resulting in a transient transition from LiPSs enriched phase to non-equilibrium Li2S nanocrystals. This reaction pathway reveals a new transformation mechanism, which is different from the traditional electrochemical reaction in which LiPSs are gradually converted to Li2S2 and Li2S, which depends on the basic understanding of LSBs[23].
Main problems of sulfur cathodes for LSBs
The insulation and volume expansion of sulfur
Due to the different densities of sulfur and Li2S (2.07 and 1.66 g cm-3, respectively), the conversion from S8 to Li2S leads to a huge volume expansion of sulfur cathodes (~80%), which will inevitably pulverize the electrode after long cycles. In addition, electrically insulating sulfur has a low conductivity of about
Shuttle effect
LiPS is an intermediate product formed by the reduction reaction of sulfur and has a high solubility in ether electrolytes[26]. It can act as an activator that can promote sulfur redox kinetics and contribute to forming a uniform dispersion of sulfur in the cathode to contact ions and electrons[27]. However, the good mobility of LiPS makes it irreversibly dissolve into the electrolyte and diffuse to the negative electrode due to the concentration gradient, which can be gradually reduced to Li2S, resulting in a loss of active material. Dissolved LiPS can be further reoxidized to sulfur after the charging process. This free migration of LiPS back and forth in the electrolyte between the cathode and anode is called “shuttle effect”[28]. The shuttle effect causes the loss of sulfur easily, which mainly accounts for a capacity drop of LSBs. During the charging process, some migrated Li2S will shuttle back to the cathode and redeposited unevenly in the sulfur region, which seriously affects the performance of LSBs[29].
Sluggish kinetics of sulfur conversion
Solid S8 is converted to soluble long-chain LiPS and subsequently to solid Li2S at the end of the discharge process. Then, unconverted Li2S in the anode results in a lower discharge plateau and a higher overpotential, increasing the impedance of electron and ion transport[30]. After cycles, the reduction of sulfur species decreases the effective capacity and energy density of LSBs. Therefore, sulfur conversion kinetics will affect the overall performance of LSBs[31].
Advancements in carbon-based sulfur host materials for LSBs
Currently, reasonable design of sulfur hosts has been a primary breakthrough to solve the abovementioned problems. Porous carbon with high electronic conductivity, good mechanical stability, and an adjustable pore structure is an ideal host for loading sulfur[32]. Its porous structure can make sulfur dispersed uniformly, alleviate volume expansion of sulfur, and limit LiPS dissolution through physical confinement. Compared with physical confinement, chemisorption can trap LiPS more efficiently and improve the cycling stability of sulfur cathodes. Furthermore, catalysts can be introduced into sulfur hosts, which can regulate the deposition of Li2S and accelerate LiPS redox kinetics as well, thus improving the electrochemical performance of LSBs[33]. Therefore, a design of multifunctional sulfur hosts to enhance the adsorption and sulfur redox kinetics by the triple synergistic effects of physical confinement, chemical adsorption, and catalytic conversion is expected to be an efficient strategy to break through existing bottlenecks of sulfur cathodes in LSBs[34].
Due to the poor conductivity of sulfur, it is difficult to directly obtain electrons from the external circuit, so sulfur needs to be combined with conductive materials to improve the conductivity of sulfur cathodes[35]. Porous carbon spheres with mesoporous/microporous structures are widely used as sulfur hosts due to their protective structural integrity. Thereinto, the porous carbon shell can prevent the outward diffusion of LiPS through the physical barrier, while the larger space inside can improve the sulfur loading and relieve the volume expansion of sulfur. In addition, multistage pores are also conducive to accelerating ion transport and limiting LiPS dissolution[36]. However, due to the weak physical force between the non-polar surface of carbon and polar LiPS, the shuttle effect is hard to inhibit. Therefore, by modifying non-polar carbon, S8 and Li2S can be evenly distributed on the surface of carbon through chemisorption and catalysis to ensure strong electric contact and relieve shuttle effect of LiPS[37]. Heteroatom-doping carbon materials, e.g., B, N, O, and S, can adsorb and enrich LiPS at the electrochemical interface through chemical bonding, enhancing the reaction kinetics[38]. Some transition metal compounds, including transition metal sulfides, nitride, and oxides, have strong interaction with LiPS, contributing to catalyzing the conversion of LiPS into Li2S, which reduces LiPS accumulation in the electrolyte and alleviates shuttle effect[39,40]. When designing a functional sulfur host, multiple functions, such as physical confinement, chemisorption, and catalytic conversion, should be taken into consideration comprehensively to enhance the performance of LSBs[41].
Physically confined materials
Porous carbon has high electronic conductivity, good mechanical stability, and an adjustable pore structure, so it is an ideal host for sulfur loading. The stability of the sulfur cathode is improved mainly through physical confinement. In recent years, researchers have devoted themselves to developing nano-carbon materials with different morphologies and structures as sulfur hosts[42]. According to pore size (d), carbon materials can be divided into three types: (1) microporous carbon (d < 2 nm); (2) mesoporous carbon
Summary of electrochemical performance of physically confined materials for LSBs
Sulfur hosts | SBET (m2 g-1) | Vtotal (cm3 g-1) | Sulfur content (wt.%) | Sulfur loading (mg cm-2) | Initial capacity (mAh g-1) | Current density (C) | Cycles | Last capacity | Rate capacity | Refs. |
CMK-3 | 1,976 | 2.1 | 70 | / | 1,000 | 0.1 | 20 | 800 | / | [44] |
MCC-8 | 761 | 1.33 | 71 | 1.5 | 1,143 | 0.5 | 200 | 869 | 943 (2 C) | [47] |
C60-S | / | / | 75 | 4.0 | 1,121 | 0.2 | 350 | 691 | 530 (2 C) | [49] |
NF@VG | 760 | / | 70.1 | 7.0 | 738 | 1.0 | 600 | 609 | 783 (3 C) | [51] |
/ | / | 57.1 | / | 920 | 0.5 | 500 | ~600 | 634 (5 C) | [56] | |
HCF/CNT | / | / | / | 7.3 | ~1,100 | 0.2 | 60 | 945 | 876 (0.5 C) | [57] |
WLC-CNTs | 130.4 | / | 79 | 17.3 | 862 | 0.2 | 300 | 547 | 840 (0.5 C) | [58] |
According to their dimensions, carbon materials can be divided into zero-dimensional (0D), one-dimensional (1D), two-dimensional (2D), and three-dimensional (3D) structures. In the context of dimensionality, 0D usually refers to materials with all sizes in the nanoscale range, such as carbon nanospheres, quantum dots, fullerenes (C60), etc., which belong to the 0D structure. Hollow carbon spheres (HCSs) are regarded as ideal hosts for loading sulfur due to their unique structural characteristics of the large inner cavity and abundant outer porous shell. The carbon cavity inside can efficiently load sulfur to achieve high sulfur content, and the carbon shell can improve the conductivity of sulfur cathodes and prevent LiPS dissolution[45]. However, the size of sulfur particles in the single cavity is relatively large, and the sulfur in the central part cannot be well-exploited because gaining and losing electrons far from the carbon wall is difficult[46]. Meanwhile, the volume expansion of large sulfur particles will cause a breakage of HCS in the charging and discharging process, resulting in capacity decline and cycle deterioration. As shown in Figure 3A, Zhang et al. developed a surface energy-driven self-assembly method for the preparation of multi-cavity carbon nanospheres (MCC)[47]. The secondary structural units were spontaneously assembled to form multi-cavity aggregates with low surface energy. The internal large cavity of MCC is divided into many small cavities connected by porous carbon walls. The volume, connection, number, and size of the cavity can be adjustable. Thereinto, the size of the cavity can be further increased by employing “nanospace confined pyrolysis”. When the MCC serves as the sulfur host, the sulfur content is up to 71 wt.%. A small cavity can reduce the size of sulfur particles, thus relieving volume expansion of sulfur cathodes. The interconnected carbon network improves sulfur utilization, which is conducive to the adsorption of LiPS produced in the charging-discharging process, thus improving the cyclic stability of MCC@S cathodes. The prepared MCC@S electrode can maintain a capacity of 869 mA h g-1 after 200 cycles at 0.5 C and reach a capacity of 943 mA h g-1 at 2 C. The cycle performance was significantly improved compared to the single cavity carbon sphere (with a low capacity of 636 mA h g-1 after 100 cycles at 0.5 C). The results show that MCC has high efficiency in sulfur fixation and utilization. Liu et al. prepared ZCO-QDs@HCS-S cathodes by in situ growing ZnCo2O4 quantum dots (ZCO-QDs) on HCS[48]. Thereinto, mesoporous HCS can improve the overall conductivity and sulfur loading of the cathode and facilitate the penetration of electrolytes and Li+ diffusion. The sulfur electrode has excellent cycle stability, with a capacity decay rate of 0.057% after 1,000 cycles at 3 C. Xiang et al. reported a novel C60-S supramolecular complex as the cathode for LSBs, in which 100% of C60 molecules provide active sites for the LiPS adsorption and promote LiPS conversion owing to the eutectic structure[49]. In addition, the lithiated C60 helps to accelerate the Li+ transport in the sulfur cathode and improve the electrochemical performance in the lean electrolyte. When the electrolyte/sulfur (E/S) ratio is 5 μL mg-1 and sulfur loading is 4 mg cm-2, the specific capacity of C60-S electrodes is 606 mA h g-1 (2.4 mA h cm-2) after 100 cycles at 0.2 C.
Carbon nanotubes (CNTs) and carbon nanofibers (CNFs), as 1D nanomaterials, have a high length-diameter ratio and good electrical conductivity, which are conducive to the construction of the conductive network in the electrode and the improvement of electronic conductivity of sulfur cathodes. Meanwhile, CNTs and CNFs are often taken as sulfur host materials due to their high mechanical strength, reducing the volume expansion of sulfur effectively. Fu et al. studied the electrochemical reaction of Li and S in narrow-diameter single-walled CNTs (SWNTs)[50]. The results show that the electrochemical performance can be significantly adjusted by changing the diameter of SWNTs and the size of solvated Li+. The average diameter of HiPco-SWNTs is 1.0 nm prepared by the high-pressure carbon monoxide method, which is smaller than the size of solvated Li+. Therefore, the Li reacts with S limited in HiPco-SWNTs through solid phase reaction, thus improving the electrochemical reaction activity. Lin et al. prepared a nitrogen-doped porous carbon fiber/vertical graphene (NF@VG) composite as a sulfur host[51]. The porous structure and nitrogen doping effectively enhance the adsorption effect for LiPS through physical limitation and chemisorption, while the VG network greatly promotes electron transfer and speeds up the LiPS conversion kinetics. Under the conditions of high sulfur loading (13 mg cm-2), high sulfur content (81.6 wt.%), and low E/S
When 3D porous carbon served as a sulfur host, the performance of sulfur cathodes can be enhanced owing to the advantages of mesoporous and microporous structures[54,55]. Therefore, a self-standing 3D hierarchical graphene host modified with N-doped nanoarrays (3DRGO/NC-BL) was constructed [Figure 3B][56]. Due to high conductivity, strong affinity, and optimized Li+ transport path, 3DRGO/NC-BL can effectively promote the uniform Li+ deposition and sulfur conversion. In particular, the extra graphene layer can effectively inhibit shuttle effect of sulfur cathodes. Therefore, the S cathode shows a high specific capacity and ultrahigh capacity retention. More importantly, an integrated LSB exhibits long-cycle stability and good flexibility. When the sulfur loading is high, shuttle effect is more serious and S8/Li2S deposition will not be uniform, which aggravates the capacity attenuation of the battery.
How to develop high sulfur loading and areal capacity is of great significance for the construction of high energy density LSBs. He et al. prepared a self-standing carbon film (HCF/CNT) in which CNTs and N, O co-doped hollow carbon fibers (HCF) are “welded” with each other by a chemical vapor deposition method [Figure 3C][57]. The HCF/CNT-S film shows a high areal capacity and cycling stability. When the sulfur loading is 10.2 mg·cm-2, the areal specific capacity is 8.9 mAh cm-2 after 60 cycles at 0.2 C. The HCF/CNT film shows that the contact resistance of CNTs and HCF is reduced by welding together through the carbon layer, which can significantly improve the conductivity of the carbon film and sulfur utilization. Meanwhile, the cavity of HCF can encapsulate a large amount of sulfur and realize high sulfur loading. N and O doping can enhance LiPS adsorption and improve the cyclic stability of the sulfur cathode. In addition, CNTs and HCF are interwoven into large pores, which can provide a channel for rapid electrolyte transfer. This study puts forward an original idea on construction of high-areal-capacity sulfur hosts. The traditional electrode with high areal specific capacity is prepared by casting slurry on the current collector. However, due to the large shrinkage of slurry in the drying process, the active electrode material will fracture and crack, resulting in poor mechanical stability. The binder is an electrical insulation that blocks the path of electrons and reduces the conductivity of the electrode, especially for thick electrodes.
Additionally, the increase in electrode thickness leads to the deteriorative charge transfer kinetics. As shown in Figure 3D, a wood-like carbon with a forest of CNTs (S@WLC-CNTs) is prepared by an ice template method and catalytic tip growth method as the skeleton in the sulfur cathode, which has high electrical conductivity and strong wood structural characteristics[58]. This unique S@WLC-CNTs electrode has microchannels of low curvature that reduce charge diffusion paths of ions and electrons, allow the electrolyte to move freely within the electrode, and mitigate volume changes of sulfur cathodes. In addition, electron-rich CNT forest can effectively capture soluble LiPS through non-polar interactions and catalyze the redox kinetics of LiPS conversion in the electrode.
Chemisorption and catalytic conversion materials
Since the sulfur cathode of LSBs is difficult to meet the demand of practical application, the primary breakthrough to solve the above problems is to design the sulfur host materials reasonably[59]. Compared to physical limiting effect, chemisorption can anchor LiPS more effectively and enhance cycle stability of sulfur cathodes. Then, a variety of catalytic catalysts have been introduced into sulfur hosts, which can regulate
Metal-free materials
(1) Heteroatom-doping carbon
According to surface chemistry, carbon materials can be divided into heteroatom-doping and functional groups. For non-polar carbon, heteroatom-doping can be a straightforward method to achieve surface functionalization of carbon materials. Heteroatom-doped porous carbon has a high specific surface area, which makes the sulfur disperse evenly[60]. The porous structure restricts the LiPS dissolution by physical confinement[61]. The heteroatoms can act as anchoring sites to chemically adsorb LiPS, which is conducive to the preparation of high-performance sulfur cathode materials[62]. Graphene oxide (GO) can be considered as an oxygen-doped graphene due to a large number of oxygen-containing groups, e.g., epoxide and -OH groups. This oxygen-doping graphene shows high affinity with LiPS, thus effectively inhibiting LiPS diffusion[63]. However, excessive oxygen functional groups can impair the high conductivity of carbon materials. GO is prone to partial reduction at high temperatures to form reduced GO (rGO), which has high conductivity and residual oxygen groups, offering sites for LiPS adsorption[64]. An all-graphene electrode formed by a graphene current collector with high conductivity and partial GO was proposed, showing good cyclic stability even under high sulfur loading, where GO as a polar component to inhibit LiPS dissolution[65].
Nitrogen-doping graphene can be conductive skeletons and LiPS adsorbents as well. Song et al. synthesized graphene sheets with large SBET and abundant N-containing groups (NG)[66]. Transmission electron microscope (TEM) images of NG are shown in Figure 4A. This NG-S electrode provided a high areal specific capacity of 5 mA h cm-2 when the sulfur loading reached 5 mg cm-2, demonstrating good cyclic stability. Nitrogen-doping carbon nanospheres (NCSs) as sulfur hosts have been synthesized with a sulfur loading of 5 mg cm-2[67]. In addition, NCSs have polar sites, which can form Li-N bonds with LiPS and combine with the micropore restriction effect to further enhance the adsorption towards LiPS, thus achieving good cyclic stability. The electrochemical performance of some chemisorption and catalytic conversion materials as sulfur hosts for LSBs has been summarized in Table 2.
Summary of electrochemical performance of chemisorption and catalytic conversion materials for LSBs
Sulfur hosts | Sulfur content (wt.%) | Sulfur loading (mg cm-2) | Initial capacity (mAh g-1) | Current density (C) | Cycles | Last capacity | Rate capacity | Refs. |
NG | 90 | 1.5 | 1,082 | 0.5 | 200 | 833 | 950 (1 C) | [66] |
UHCS | / | / | 930 | 0.5 | 200 | 675 | 630 (2 C) | [70] |
CC/poly(Li2S6-r-DIB) | 43 | / | 1,200 | 2 A g-1 | 120 | 934 | 727 (2 A g-1) | [82] |
CNG | 50 | / | 1,280 | / | 280 | 700 | / | [89] |
CoSe2/Co3O4@NC-CNT | ~ 75 | 2.0 | 780 | 1.0 | 500 | 602 | 688 (5 C) | [102] |
CC@Co0.85Se/NC | / | 1.0 | 953 | 1.0 | 400 | 799 | / | [107] |
Co9S8/C | 77 | 1.5 | 1,020 | 0.5 | 200 | 790 | 810 (3 C) | [108] |
MoS2-X/rGO | 75 | 1.5 | ~1,251 | 0.5 | 600 | 628.2 | 690 (3 C) | [110] |
MoP/GO | 73 | 1.0 | ~739 | 1.0 | 11,566 | 597 | 656 (2 C) | [115] |
TiO2-TiN | 80 | 1.2 | ~790 | 1.0 | 2,000 | 704 | 682 (2 C) | [125] |
La2O3-MXene@CNF | 75.24 | 1.0 | 992.6 | 0.2 | 400 | 846 | 747.7 (3 C) | [128] |
To learn the interaction between LiPS and heteroatomic doping sites, 7Li nuclear magnetic resonance (NMR) spectra have been conducted, combined with Density Functional Theory (DFT) calculations and experimental data[68]. It was found that pyridine N composed of lone pair electrons has a strong interaction with Li+.
Xu et al. used P2O5 as a sacrificial template to synthesize phosphorus and oxygen-doping porous carbon sheets[69]. Phosphorus-doping porous carbon has been prepared by heating phosphorous compounds reduced by carbon at high temperatures. The X-ray photoelectron spectroscopy (XPS) spectra of P-doping carbon changed before and after the Li2S6 adsorption, indicating the strong binding effect with LiPS. Therefore, P-doping carbon as a sulfur host delivers relatively stable cycling performance with an average capacity decay of 0.025% after 1,000 cycles at 1 C.
Besides the introduction of external heteroatomic precursors, heteroatom-doping carbon materials can also be prepared by using a precursor composed of heteroatomic groups. Metal-organic frameworks (MOF) containing organic ligands and rich functional groups can serve as precursors for the synthesis of heteroatom-doping carbon skeletons. That helps the carbon skeleton achieve heteroatomic self-doping through an easy step. As shown in Figure 4B, Hao et al. prepared ultra-hydrophilic carbon nanosheets (UHCS) by a thermal stripping MOF precursor (4,4′-bipyridine copper)[70]. The MOF is a 2D layered structure that interacts with other molecules through π-π bonds. Several merits of carbon nanosheets prepared by using the exfoliated MOF are listed: (1) UHCS are composed of centrally distributed micropores (~0.7 nm); (2) There are abundant functional groups in a MOF precursor. After carbonization at 500 and 900 °C, the heteroatom-doping content of UHCS is as high as 30 and 24 at.%, respectively. Therefore, the surface of carbon nanosheets is polar and highly hydrophilic. As a sulfur host, it provides abundant polar sites for LiPS adsorption so that the sulfur cathode has a stable cycling performance. To solve the poor conversion kinetics of sulfur species and Li+ transport, Liu designed a bridging structure of the MOF-carbon interface to achieve catalytic acceleration of Li+ desolvation and sulfur conversion processes[71]. At the interface conferring ion-electron double conductance, the active C-N bridging bonds in the MOF@CC structure serve as the adsorption and catalytic sites, which reduces the binding effect between solvent molecules and Li+ and enables the highly active Li+ that are free from the solvent shell to conduct to the site more quickly, and the electron exchange reaction occurs when they contact with LiPS. Based on the designed MOF@CC separator, the LSB delivers excellent reversibility (0.5 C, 1,063 mA h g-1) and rate performance (5 C, 765 mA h g-1).
(2) Polymers modified carbon
Functional groups that provide additional lone pair electrons can coordinate with Li+, resulting in interacting strongly with LiPS[72]. Therefore, the combination of carbon-based materials and functional groups can exert their superiorities to enhance cycle performance of the sulfur electrode[73,74]. Polymers, such as polyvinylpyrrolidone (PVP)[75], polyimide (PI)[76], poly (3,4-vinyldioxythiophene) (PEDOT)[77] and polyaniline (PAN)[78], some of which have good electronic conductivity and positively charged amino groups, can attract negatively charged polysulfide anions through static electricity[79]. Therefore, it can be directly applied or modified as a sulfur host to improve the cyclic stability of the sulfur electrode. The PAN-coated S-C cathode (a sulfur content of 73 wt.%) was prepared [Figure 5A][80]. After cycling 2,500 times at 5 C, the capacity decay is as low as 0.01%, which shows good cycle stability. PAN is commonly taken as a coating for sulfur due to the abundance of nitrogen-containing groups on the skeleton. To improve the chemisorption effect, Chen et al. grafted phytic acid (PA) containing P onto the
Figure 5. (A) Schematic of synthetic procedure for PAN-assisted S/C nanospheres[80]. Copyright American Chemical Society 2015. (B) Schematic illustrating the stabilization and enrichment of quinonoid imines by PA[81]. Copyright John Wiley and Sons 2017. (C) Synthetic schematics of the Li-rich sulfur-incorporated polymeric cathodes, (D) digital photographs, (E) IR absorption characterization of the poly(Li2S6-r-DIB) copolymers, (F) digital images of poly(Li2S6-r-10 wt.% DIB) before and after tilting the vial at room temperature and 60 °C, and (G) digital images of poly(Li2S6-r-10 wt.% DIB) transparent films cast in a glass dish[82]. Copyright American Chemical Society 2021.
It is essential to construct a hierarchical channel structure with high pore volume to store sulfur and introduce strong polar segments and catalytic sites to promote the catalytic conversion of LiPS. A copolymer poly (Li2S6-r-DIB) was prepared by covalent polymerization of Li2S6 to 1,3-diisopropenylbenzene (DIB), as shown in Figure 5C-G[82]. The LiPS dissolution was effectively inhibited by reinforcing the chemical restriction of Li2S6 by a conjugated polymer framework. During the discharging-charging process, π electron in poly (Li2S6-r-DIB) promotes rapid electron migration, and the extra Li+ compensates for Li loss and promotes the redox kinetics of Li+, which improves the sulfur utilization and Coulombic efficiency of the battery. In addition, by using the thermoplastic properties, poly (Li2S6-r-DIB) is directly coated on the flexible conductive carbon cloth to prepare a flexible sulfur electrode. The gap space of the carbon cloth is conducive to buffering the volume expansion of sulfur to obtain stable long-term cycling performance. On this basis, the flexible LSB was assembled by matching the modified carbon cloth/Si/Li flexible anode, which showed stable electrochemical performance under different folding conditions.
A semi-fixed molecular catalyst was designed, in which porphyrins were covalently grafted on conductive and flexible polymer chains as the active sites so that they had both homogeneous and heterogeneous catalytic functions[83]. Semi-fixed molecular catalysts play a key part in accelerating electrochemical reaction and regulating deposition behavior of reactants in heterogeneous reactions. Meanwhile, the actual energy density of the 1.5 Ah soft pack battery can reach 343 Wh kg-1.
(3) Other metal-free materials
According to literature reports, many non-metallic materials (silicene, phosphorene, 2D borides, nitrogen carbide, boron nitride[84], and other low-dimensional materials) have catalytic activity mediated by p orbitals[85], which can be used as electrocatalysts to promote the conversion of soluble LiPS to insoluble
Metal-based materials
(1) Metal
Compared with non-metallic materials, metal-based materials have higher electronic conductivity. They not only have a stronger chemisorption effect on LiPS but also serve as catalysts to accelerate redox reaction of LiPS[90,91]. A unique multifunctional double-layer carbon structure with micron-scale physical limitation is proposed; that is, metal Co and CNTs constitute a fluffy porous inner carbon skeleton, which can accelerate electron transport and provide sufficient space for LiPS conversion[92]. The outer carbon is composed of a micron-thick graphene nanoflower layer (GF), which efficiently limits the diffusion of soluble LiPS, playing an important role in physically inhibiting the shuttle effect. Meanwhile, metal Co serves as a chemical adsorption site and an electrocatalyst, which can chemically trap LiPS and accelerate LiPS transformation[93]. After 200 cycles at 2 C, the capacity of Co/CNT@GF-S electrodes remains at
(2) Metal oxides
Due to the presence of oxygen ions, metal oxides have strong polar surfaces[94]. Therefore, owing to abundant polar active sites, metal oxides have strong chemisorption on LiPS, which greatly promotes the sulfur redox reaction[95]. Different morphologies of titanium dioxide (TiO2), such as nanospheres, nanotubes, and nanosheets, can prevent the diffusion of LiPS through chemisorption. Early, TiO2 shells were taken to suppress the volume expansion of sulfur[96]. A TiO2 layer is a coating on the sulfur core to form a yolk-shell structure so that it can maintain a complete shell structure during the process inhibiting the volume expansion of sulfur. In the sulfur electrode, the combination of carbon and TiO2 contributes to accelerating charge transfer kinetics. Moreover, a TiO2 atomic layer was deposited on the NG-S electrode to prepare a TiO2/NG-S electrode[97]. TiO2 has strong chemical adsorption towards LiPS, which slows down the shuttle effect of LiPS and significantly improves the performance of LSBs. The initial specific capacity of the TiO2/NG-S electrode reached about 1,069 mA h g-1 at 1 C and maintained 919 mA h g-1 after 500 cycles. Fang et al. prepared TiO2 sandwiched between HCSs (C@TiO2@C) as a sulfur host[98]. The TiO2 layer anchors the LiPS through chemisorption, while the double-layer carbon shell provides electron transport paths and acts as a physical blocking in space to limit LiPS dissolution. Additionally, a hollow structure mitigates the volume expansion of sulfur during the lithiation process. When the sulfur content is
MnO2 has strong adsorption towards LiPS, but the conductivity of the S-MnO2 electrode is low.
Co3O4 nanoparticles could improve the affinity towards LiPS[100].
It is still a challenge to design electrodes with high conductivity, high capacity, and strong affinity for LiPSs to meet the requirements of LSBs with high S content and long-term cycle stability. Three-phase interface heterostructures
Figure 7. (A) Schematic diagram illustration of the synthesis procedure of the CoSe2/Co3O4@NC-CNT heterostructure[102]. Copyright John Wiley and Sons 2022. (B) Schematic illustration of the fabrication process for CC@Co0.85Se/NC, and (C-E) SEM images of CC@Co0.85Se/NC[107]. Copyright American Chemical Society 2021.
(3) Metal sulfides/selenides
For metal sulfides, metal ions and S22- give metal atoms high valence electron density, thus generating binding sites for non-intrinsic adsorbents[103]. In addition, the soft base S22- is highly polarizable, thus giving the metal sulfides high conductivity. Therefore, the metal sulfides can reduce the decomposition barrier and enhance the redox reaction kinetics of LiPS by chemisorption[104]. By observing the color changes of Li2S6 and 1,2-dimethoxyethane (DME) solution and the DFT calculation, it can be verified that the adsorption ability for Li2S6 by metal sulfides is strongly linked to the kinds of metal ions. To study the adsorption behavior of Li2S6 on surfaces of metal sulfides, Ni3S2, SnS2, FeS, CoS2, VS2, and TiS2 were added to Li2S6/DME solutions[105]. It was found that the color of solution became lighter or transparent, indicating that metal sulfides had an excellent ability to adsorb LiPS. The color change is correlated to the binding energy of metal sulfides and Li2S6, where VS2 has the strongest interaction with Li2S6 (1.04 eV). The specific discharge capacity of VS2/S cathodes at 2 C is 1,093 mA h g-1, and the capacity decay is 0.07%, which is better than that of other metal sulfides.
Cobalt-based sulfides/selenides are commonly used as sulfur hosts due to their excellent room temperature conductivity (1.36 × 105 S m-1 for Co9S8, 6.7 × 105 S m-1 for CoS2, and 3.3 × 105 S m-1 for Co3S4)[106]. As shown in Figure 7B-E, a MOF-derived bi-functional Co0.85Se nanoparticle embedded in 2D N-doped carbon nanosheets (Co0.85Se/NC) was developed as an efficient sulfur host for LSBs[107]. The interleaved NC grown on carbon fibers can provide accommodation space and reactive sites for LiPSs, which play a role in physical limitation and alleviating volume expansion. Polar Co0.85Se nanoparticles modified by NC can form strong chemical bonds with LiPSs and accelerate LiPS conversion kinetics. The specific discharge capacity of the Co0.85Se/NC-S electrode reaches a capacity of 1,361, 1,001, and 810 mA h g-1 at 0.1, 1, and 3 C, respectively. After 400 cycles at 1 C, the capacity of Co0.85Se/NC-S electrodes reaches 799 mA h g-1, and the attenuation is ~0.04% per cycle.
Chen et al. synthesized Co9S8 nanoparticles embedded in hollow nano polyhedral carbon (Co9S8/C) by a template and vulcanization method[108], as shown in Figure 8A. Co9S8/C composites have many advantages: (1) Large internal cavity can contain high content of sulfur, easing the volume expansion of sulfur; (2) The embedded Co9S8 nanoparticles can effectively adsorb LiPS and inhibit the shuttle effect; and (3) a 3D conducting network provides a fast electron transfer path. DFT calculation showed that the adsorption energy of Co9S8 nanoparticles for different sulfur species ranged from 2.97 to 6.08 eV, indicating that Co9S8 nanoparticles had strong chemisorption capacity. The chemical interaction between sulfur and Co9S8 is mainly due to the formation of Co-S and Li-S bonds. The specific capacity of the Co9S8/C-S electrode was 560 mA h g-1 after 1,000 cycles at 2 C.
Figure 8. (A) Schematic illustration of the synthesis process and cycle performance of Co9S8/C-S nanopolyhedra[108]. Copyright Elsevier 2017. (B) Schematic of the synthesis of the MoS2-x/rGO composite; TEM images of (C) GO and (D and E) MoS2 nanoflakes[110] Copyright RSC Publishing 2017. (F) Schematic structure and long-term cycle performance of CNT-MoP/GO-S[116]. Copyright Springer Nature 2017.
Molybdenum disulfide (MoS2) exhibits a 2D layered structure with good electrocatalytic activity[109]. As shown in Figure 8B-E, Lin et al. prepared sulfur-rich defective MoS2 nanosheets modified with rGO (MoS2-x/rGO) as a sulfur host[110]. Due to abundant sulfur defects, MoS2-x/rGO shows a strong affinity towards LiPS and promotes LiPS redox reaction, thus enhancing sulfur utilization. Similarly, You et al. prepared a 3D sulfur host (MoS2/rGO) by combining MoS2 nanosheets with rGO foam[111]. Hu et al. prepared HCS with nanobubbles by using Ni-MOF as a precursor[112]. A layer of MoS2 nanosheets was then coated on the surface of HCS by a solvothermal method. The results show that the specific capacity of the S/MoS2@HCS electrode is 1,048 mAh g-1 at 0.2 C. Good electrochemical performance of S/MoS2@HCS electrodes is mainly owing to: (1) Hollow nanobubbles provide enough space for sulfur loading and reduce the volume expansion of sulfur; (2) High conductivity ensures fast electron transmission and improves the sulfur utilization; (3) MoS2 nanosheets coated on the surface of HCS effectively limited LiPS diffusion through chemisorption; and (4) MoS2 nanosheets, as an electrocatalyst, promote the reversible conversion of LiPS to Li2S.
(4) Metal phosphides
For metal phosphides, because P atoms can obtain electrons from metal atoms and serve as Lewis bases to capture positively charged species, metal phosphides show chemical adsorption towards LiPS and catalytic activity to accelerate LiPS redox reaction[113,114]. Transition metal phosphides possess metallic properties when the atomic ratio of metal to P is appropriate. Therefore, metal phosphides are often taken as LiPS adsorbents and catalysts due to their superior electronic conductivity and strong polarity[115]. MoP nanoparticles decorated on CNTs (CNT-MoP) were taken as a sulfur host[116], as shown in Figure 8F. MoP has a strong chemisorption effect on LiPS, which improves the cycling stability of CNT-MoP-S electrodes. In addition, experimental results showed that the CNT-MoP electrode exhibited higher redox current density and lower charge transfer resistance, indicating that MoP nanoparticles can accelerate the redox kinetics of LiPS conversion. Ye et al. prepared CoP nanoparticles embedded in hollow polyhedron/CNT (CoP@HPCN-MWCNT) as sulfur hosts with the following advantages: (1) The hollow polyhedron structure can alleviate the volume expansion of sulfur; (2) Uniformly dispersed CoP nanoparticles can effectively catalyze the LiPS conversion; and (3) Conductive polyhedral carbon shell can promote Li+ and electron transport[117]. When the sulfur loading is 3.7 mg cm-2, the CoP@HCPN-MWCNT/S electrode delivers a capacity of 729 mA h g-1 after 200 cycles. Huang et al. synthesized iron phosphide (FeP) nanocrystals as sulfur hosts[118]. It was found that FeP nanocrystals not only show chemisorption towards LiPS but also can accelerate redox reaction and reduce nucleation barrier of Li2S. The FeP/rGO/CNTs electrode shows higher redox charge transfer ability, indicating stronger catalytic activity. In brief, FeP nanocrystals as sulfur hosts have two advantages: (1) FeP nanocrystals have chemisorption for LiPS, effectively reducing the shuttle effect of LiPS; (2) FeP nanocrystals act as catalysts in sulfur redox reaction, promoting LiPS conversion. Therefore, the FeP/rGO/CNT-S electrode has good cyclic stability, and the capacity decay is 0.04% after 400 cycles at 1 C.
Other catalytic materials
(1) Single-atom transition metal catalysts
Single-atom (SA) transition metal catalysts have attracted great attention due to their unique quantum size and high-frequency element utilization[119]. In the sulfur cathode, the SA catalyst combines strong chemisorption for LiPS, rapid electron transfer, and improved electrolyte permeability, which promotes LiPS conversion and Li2S deposition and improves sulfur utilization[120,121]. Du et al. studied the electrochemical performance of Co-SAs embedded in N-doped graphene skeleton (Co-N/G) as a sulfur host[122]. When the sulfur loading is 6.0 mg cm-2, the initial specific discharge capacity of the Co-N/G-S electrode is 1,210 mA h g-1 at 0.2 C, and the capacity decay is 0.029% after 100 cycles. As Fermi level density increases, Co-N/G exhibits stronger metallic properties, resulting in higher electrical conductivity of
(2) Heterostructures catalysts
The heterogeneous structure combines the advantages of various electrocatalysts to improve the efficiency of catalysts. TiO2 has strong adsorption towards LiPS, while its low electron conductivity is not conducive to speeding up LiPS conversion. As a result, LiPS adsorbed on the TiO2 surface requires a longer diffusion process to reach the conductive substrate and then be reduced, which may limit the overall reaction rate. TiN can promote nucleation and growth of Li2S, while it shows weak adsorption capacity for LiPS. As shown in Figure 9A, TiN and TiO2 were combined to form a heterogeneous structure, and LiPS adsorbed on the surface of TiO2 can be diffused to the surface of TiN to be reduced, thus accelerating the kinetics of LiPS redox reaction[125]. The heterogeneous structure of the TiO2-TiN electrocatalyst is shown in Figure 9B, which co-exists in the form of large particles. The interface between them not only serves as a highly active site to adsorb LiPS but also provides a fast electron transfer path for LiPS conversion.
Figure 9. (A) Schematic of LiPS conversion processes, (B) TEM image of TiO2-TiN structure, and (C) potentiostatic discharge curves at 2.05 V on different surfaces[125]. Copyright RSC Publishing 2017. (D) Schematic illustration for the preparation process, SEM images of the electrospun La2O3-MXene nanofibers after (E) spinning and (F) carbonization, and (G) TEM images of La2O3-MXene@CNF[128]. Copyright John Wiley and Sons 2023.
As shown in Figure 9C, heterogeneous catalysts (CP-2TiN:8TiO2 and CP-7TiN:3TiO2) have a higher capacity of Li2S deposition than single catalysts (TiN or TiO2) when Li2S deposition experiment is conducted. This suggests that electrocatalysts with heterogeneous structures can promote the conversion of LiPS to Li2S. Similarly, VO2 shows weak adsorption capacity for LiPS, which can be combined with VN to form a heterostructure (VO2-VN) to optimize the performance of electrocatalysts[126]. To optimize the energy band of transition metal oxides, Wang et al. designed a microcatalytic reactor with Co3O4/ZnO heterojunction embedded in polyhedral carbon nanocages (CZO/HNC) to serve as a bi-functional host for S and Li electrodes[127]. The internal electric field between ZnO and Co3O4 can promote interfacial charge transfer and reaction kinetics, and CZO/HNC provides sufficient active sites for the adsorption towards LiPSs on the cathode and uniform nucleation of Li+ on the anode. Therefore, the LSBs exhibit a long lifetime of more than 1,000 cycles, and the capacity decay is as low as 0.05%.
A high-efficiency catalytic material (La2O3-MXene@CNF) prepared by a simple electrospinning and carbonization process as a multifunctional sulfur host for LSBs was proposed [Figure 9D-G][128]. The multi-dimensional characterizations combined with theoretical calculation show that the unique La2O3@MXene heterostructure has many advantages in enhancing adsorption ability and accelerating sulfur redox kinetics. It is indicated that the strong adsorption capacity provided by the La2O3 structure can trap dissolved LiPSs for subsequent catalytic conversion, while the insoluble thiosulfate intermediates produced by the hydroxyl terminal group on the surface of MXene greatly promote the conversion of LiPSs to Li2S. Therefore, La2O3-MXene@CNF/S electrodes exhibit excellent electrochemical performance in LSBs.
SUMMARY AND OUTLOOK
In view of the excellent electrical conductivity, stability and structural versatility, the review places emphasis on recent progress of carbon-based sulfur hosts for LSBs. Aimed at the problems such as poor conductivity, large volume change, shuttle effect, etc., it is an ideal choice to design carbon-based sulfur hosts with high conductivity, high chemical absorption and high catalytic effect, which needs optimization of the structure and chemical composition of sulfur hosts. The introduction of carbon materials not only improves the conductivity of sulfur hosts, thus improving the sulfur utilization, but also alleviates the LiPS dissolution in the electrolyte, significantly improving the electrochemical performance of LSBs. To prepare the ideal carbon-based sulfur hosts, attention should be paid to considering advantages of structural design and chemical modification. When designing a functional sulfur host, multiple functions, such as physical confinement, chemisorption, and catalytic conversion, should be taken into consideration comprehensively to promote the development of high-specific energy, high-power, and fast-charging LSBs. However, there are still some problems that need to be solved. As one of next-generation directions of power systems, LSBs have several issues worthy of further research, as follows.
(1) The development of carbon materials with highly active catalysts possessing multitudinous advantages, e.g., porous structure, large surface area, self-standing, polarity, and catalytic activity, is relatively independent and complex currently. However, their integrated development is still lacking. Therefore, controlling the relationship between the above three functions of carbon and performance of LSBs is a major focus in the future development. Feasible strategies, such as N, S/O or Co/Fe-N co-doping, integrating heterointerface catalysts, and SA catalysts into porous carbon, can be taken into consideration.
(2) Although catalytic sulfur hosts have made a great breakthrough in facilitating the conversion between sulfur and Li2S/Li2S2, the collaborative relationship between chemical break of the catalyst-induced S-S/Li-S bonds and sustainable active sites and barrier-free mass/electron transfer remains unclear. In addition, the relationship between the catalytic activity of catalysts and adsorption capacity, which is related to the sustainability of catalysis, is still not widely understood. These challenges require quantitative analysis and sound theoretical guidance based on in-situ characterization techniques, such as in-situ Raman, X-ray diffraction (XRD), TEM, X-ray near edge spectroscopy (XANES), etc., and detailed theoretical calculations. More in-depth characterization of the triple functions of carbon can provide insight into its active sites, mechanisms and processes so as to guide practical applications.
(3) Compared with CNTs, graphene, and their derivatives, CNFs exhibit higher mechanical flexibility and stability in electrodes. However, low specific surface area and inherent surface inertness of CNFs lead to their low use weight and high interface curvature in LSB electrodes. The synergistic structure of CNFs and carbon nanomaterials is an effective strategy to provide mechanical stability while improving the sulfur loading and inhibiting the shuttle effect. Therefore, developing carbon-based sulfur hosts with ultra-flexibility, high performance, and breathability, which can be applied in wearable devices, laid the groundwork for further development of flexible LSBs.
DECLARATIONS
Authors’ contributions
Conceptualization, investigation, and writing-original draft: Yu XF
Editing: Shao DY
Writing-review & editing, supervision, and funding acquisition: Xu J, Cao J
Availability of data and materials
Not applicable.
Financial support and sponsorship
This work was supported by the Scientific Research Start-Up Funds of Changzhou University (ZMF23020028).
Conflicts of interest
All authors declared that there are no conflicts of interest.
Ethical approval and consent to participate
Not applicable.
Consent for publication
Not applicable.
Copyright
© The Author(s) 2024.
REFERENCES
3. Mizushima K, Jones PC, Wiseman PJ, Goodenough JB. LixCoO2 (0 < x < -1): a new cathode material for batteries of high energy density. Mater Res Bull 1980;15:783-9.
4. Yao YX, Yan C, Zhang Q. Emerging interfacial chemistry of graphite anodes in lithium-ion batteries. Chem Commun 2020;56:14570-84.
5. Wang L, Liu G, Xu R, et al. Enabling an intrinsically safe and high-energy-density 4.5 V-class lithium-ion battery with synergistically incorporated fast ion conductors. Adv Energy Mater 2023;13:2203999.
6. Kundu D, Vajargah SH, Wan L, Adams B, Prendergast D, Nazar LF. Aqueous vs. nonaqueous Zn-ion batteries: consequences of the desolvation penalty at the interface. Energy Environ Sci 2018;11:881-92.
7. Zhao LF, Hu Z, Lai WH, et al. Hard carbon anodes: fundamental understanding and commercial perspectives for Na-ion batteries beyond Li-ion and K-ion counterparts. Adv Energy Mater 2021;11:2002704.
8. Bonnick P, Muldoon J. The Dr Jekyll and Mr Hyde of lithium sulfur batteries. Energy Environ Sci 2020;13:4808-33.
9. Zhao Y, Ye Y, Wu F, Li Y, Li L, Chen R. Anode interface engineering and architecture design for high-performance lithium-sulfur batteries. Adv Mater 2019;31:e1806532.
10. Ji H, Wang Z, Sun Y, et al. Weakening Li+ De-solvation barrier for cryogenic Li-S pouch cells. Adv Mater 2023;35:e2208590.
11. Manthiram A, Fu Y, Su YS. Challenges and prospects of lithium-sulfur batteries. ACC Chem Res 2013;46:1125-34.
12. Bandyopadhyay S, Nandan B. A review on design of cathode, anode and solid electrolyte for true all-solid-state lithium sulfur batteries. Mater Today Energy 2023;31:101201.
13. Zhao M, Li BQ, Zhang XQ, Huang JQ, Zhang Q. A perspective toward practical lithium-sulfur batteries. ACS Cent Sci 2020;6:1095-104.
14. Bi CX, Zhao M, Hou LP, et al. Anode material options toward 500 Wh kg-1 lithium-sulfur batteries. Adv Sci 2022;9:e2103910.
15. Gong Y, Li J, Yang K, et al. Towards practical application of Li-S battery with high sulfur loading and lean electrolyte: will carbon-based hosts win this race? Nanomicro Lett 2023;15:150.
16. Zhang T, Zhang L, Zhao L, Huang X, Hou Y. Catalytic effects in the cathode of Li-S batteries: accelerating polysulfides redox conversion. EnergyChem 2020;2:100036.
17. Yang JL, Cai DQ, Hao XG, et al. Rich heterointerfaces enabling rapid polysulfides conversion and regulated Li2S deposition for high-performance lithium-sulfur batteries. ACS Nano 2021;15:11491-500.
18. Xiang Y, Lu L, Kottapalli AGP, Pei Y. Status and perspectives of hierarchical porous carbon materials in terms of high-performance lithium-sulfur batteries. Carbon Energy 2022;4:346-98.
19. He Y, Zou P, Bak S, et al. Dual passivation of cathode and anode through electrode-electrolyte interface engineering enables long-lifespan Li metal-SPAN batteries. ACS Energy Lett 2022;7:2866-75.
20. Xing C, Chen H, Qian S, et al. Regulating liquid and solid-state electrolytes for solid-phase conversion in Li-S batteries. Chem 2022;8:1201-30.
21. Chen X, Hou T, Persson KA, Zhang Q. Combining theory and experiment in lithium-sulfur batteries: current progress and future perspectives. Mater Today 2019;22:142-58.
22. Sun X, Qiu Y, Jiang B, et al. Isolated Fe-Co heteronuclear diatomic sites as efficient bifunctional catalysts for high-performance lithium-sulfur batteries. Nat Commun 2023;14:291.
23. Zhou S, Shi J, Liu S, et al. Visualizing interfacial collective reaction behaviour of Li-S batteries. Nature 2023;621:75-81.
24. Zhou L, Danilov DL, Qiao F, et al. Sulfur reduction reaction in lithium-sulfur batteries: mechanisms, catalysts, and characterization. Adv Energy Mater 2022;12:2202094.
25. Chen Y, Wang T, Tian H, Su D, Zhang Q, Wang G. Advances in lithium-sulfur batteries: from academic research to commercial viability. Adv Mater 2021;33:e2003666.
26. Song Y, Cai W, Kong L, Cai J, Zhang Q, Sun J. Rationalizing electrocatalysis of Li-S chemistry by mediator design: progress and prospects. Adv Energy Mater 2020;10:1901075.
27. Li C, Xi Z, Guo D, Chen X, Yin L. Chemical immobilization effect on lithium polysulfides for lithium-sulfur batteries. Small 2018;14:1701986.
28. Tang T, Hou Y. Multifunctionality of carbon-based frameworks in lithium sulfur batteries. Electrochem Energy Rev 2018;1:403-32.
29. Li Z, Zhou Y, Wang Y, Lu Y. Solvent-mediated Li2S electrodeposition: a critical manipulator in lithium-sulfur batteries. Adv Energy Mater 2019;9:1802207.
30. Yuan H, Peng H, Li B, et al. Conductive and catalytic triple-phase interfaces enabling uniform nucleation in high-rate lithium-sulfur batteries. Adv Energy Mater 2019;9:1802768.
31. Hou R, Zhang S, Zhang Y, et al. A “three-region” configuration for enhanced electrochemical kinetics and high-areal capacity lithium-sulfur batteries. Adv Funct Mater 2022;32:2200302.
32. Zhang X, He B, Li W, Lu A. Hollow carbon nanofibers with dynamic adjustable pore sizes and closed ends as hosts for high-rate lithium-sulfur battery cathodes. Nano Res 2018;11:1238-46.
33. Ma F, Srinivas K, Zhang X, et al. Mo2N quantum dots decorated N-doped graphene nanosheets as dual-functional interlayer for dendrite-free and shuttle-free lithium-sulfur batteries. Adv Funct Mater 2022;32:2206113.
34. He B, Liu D, Cheng Z, et al. Enabling selenium-rich SexSy cathodes to work in carbonate-based electrolytes. Adv Energy Mater 2022;12:2102832.
35. Deng W, Phung J, Li G, Wang X. Realizing high-performance lithium-sulfur batteries via rational design and engineering strategies. Nano Energy 2021;82:105761.
36. Yu Z, Liu M, Guo D, et al. Radially inwardly aligned hierarchical porous carbon for ultra-long-life lithium-sulfur batteries. Angew Chem Int Ed 2020;59:6406-11.
37. Djuandhi L, Mittal U, Sharma N, Andersen HL. The role of carbon-based cathode components in Li-S batteries. J Electrochem Soc 2023;170:010522.
38. Wang R, Yang J, Chen X, et al. Highly dispersed cobalt clusters in nitrogen-doped porous carbon enable multiple effects for high-performance Li-S battery. Adv Energy Mater 2020;10:1903550.
39. Peng H, Zhang Y, Chen Y, et al. Reducing polarization of lithium-sulfur batteries via ZnS/reduced graphene oxide accelerated lithium polysulfide conversion. Mater Today Energy 2020;18:100519.
40. Li G, Qiu W, Gao W, et al. Finely-dispersed Ni2Co nanoalloys on flower-like graphene microassembly empowering a Bi-service matrix for superior lithium-sulfur electrochemistry. Adv Funct Mater 2022;32:2202853.
41. Zhang W, Xu B, Zhang L, et al. Co4N-decorated 3D wood-derived carbon host enables enhanced cathodic electrocatalysis and homogeneous lithium deposition for lithium-sulfur full cells. Small 2022;18:e2105664.
42. Xu Z, Kim J, Kang K. Carbon nanomaterials for advanced lithium sulfur batteries. Nano Today 2018;19:84-107.
43. Zhang LL, Zhao XS. Carbon-based materials as supercapacitor electrodes. Chem Soc Rev 2009;38:2520-31.
44. Ji X, Lee KT, Nazar LF. A highly ordered nanostructured carbon-sulphur cathode for lithium-sulphur batteries. Nat Mater 2009;8:500-6.
45. Yu X, Li W, Hu Y, Ye C, Lu A. Sculpturing solid polymer spheres into internal gridded hollow carbon spheres under controlled pyrolysis micro-environment. Nano Res 2021;14:1565-73.
46. Zhang Y, Li W, He B, Yu X, Hou L, Lu A. Utilizing the alterable solubility of chitosan in aqueous solution to synthesize nanosized sulfur for high performance Li-S batteries. Chin J Chem 2019;37:775-80.
47. Zhang L, He B, Li W, Lu A. Surface free energy-induced assembly to the synthesis of grid-like multicavity carbon spheres with high level in-cavity encapsulation for lithium-sulfur cathode. Adv Energy Mater 2017;7:1701518.
48. Liu Y, Ma Z, Yang G, et al. Multifunctional ZnCo2O4 quantum dots encapsulated in carbon carrier for anchoring/catalyzing polysulfides and self-repairing lithium metal anode in lithium-sulfur batteries. Adv Funct Mater 2022;32:2109462.
49. Xiang J, Shen W, Guo Z, et al. A supramolecular complex of C60-S with high-density active sites as a cathode for lithium-sulfur batteries. Angew Chem Int Ed 2021;60:14313-8.
50. Fu C, Oviedo MB, Zhu Y, et al. Confined lithium-sulfur reactions in narrow-diameter carbon nanotubes reveal enhanced electrochemical reactivity. ACS Nano 2018;12:9775-84.
51. Lin J, Mo Y, Li S, Yu J. Nitrogen-doped porous carbon fiber/vertical graphene as an efficient polysulfide conversion catalyst for high-performance lithium-sulfur batteries. J Mater Chem A 2022;10:690-8.
52. Peng L, Wei Z, Wan C, et al. A fundamental look at electrocatalytic sulfur reduction reaction. Nat Catal 2020;3:762-70.
53. Zhou G, Li L, Wang DW, et al. A flexible sulfur-graphene-polypropylene separator integrated electrode for advanced Li-S batteries. Adv Mater 2015;27:641-7.
54. Han Z, Zhao S, Xiao J, et al. Engineering d-p orbital hybridization in single-atom metal-embedded three-dimensional electrodes for Li-S batteries. Adv Mater 2021;33:e2105947.
55. Qi C, Li Z, Wang G, et al. Microregion welding strategy prevents the formation of inactive sulfur species for high-performance Li-S battery. Adv Energy Mater 2021;11:2102024.
56. Lu R, Cheng M, Mao L, et al. Nitrogen-doped nanoarray-modified 3D hierarchical graphene as a cofunction host for high-performance flexible Li-S battery. EcoMat 2020;2:e12010.
57. He B, Li W, Chen Z, et al. Multilevel structured carbon film as cathode host for Li-S batteries with superhigh-areal-capacity. Nano Res 2021;14:1273-9.
58. Wang N, Zhang X, Ju Z, et al. Thickness-independent scalable high-performance Li-S batteries with high areal sulfur loading via electron-enriched carbon framework. Nat Commun 2021;12:4519.
59. Qin J, Wang R, Xiao P, Wang D. Engineering cooperative catalysis in Li-S batteries. Adv Energy Mater 2023;13:2300611.
60. Yu X, Li W, He B, et al. Porous carbon/borocarbonitride hybrid with enhanced tap density as a polar host for ultralong life lithium-sulfur batteries. Chem Eng J 2022;430:132987.
61. Wang J, Han W. A review of heteroatom doped materials for advanced lithium-sulfur batteries. Adv Funct Mater 2022;32:2107166.
62. Pan J, Sun Y, Wu Y, et al. Yolk-double shells hierarchical N-doped carbon nanosphere as an electrochemical nanoreactor for high performance lithium-sulfur batteries. Carbon 2022;198:80-90.
63. Ji L, Rao M, Zheng H, et al. Graphene oxide as a sulfur immobilizer in high performance lithium/sulfur cells. J Am Chem Soc 2011;133:18522-5.
64. Zheng Y, Jiao Y, Ge L, Jaroniec M, Qiao SZ. Two-step boron and nitrogen doping in graphene for enhanced synergistic catalysis. Angew Chem Int Ed 2013;52:3110-6.
65. Fang R, Zhao S, Pei S, et al. Toward more reliable lithium-sulfur batteries: an all-graphene cathode structure. ACS Nano 2016;10:8676-82.
66. Song J, Yu Z, Gordin ML, Wang D. Advanced sulfur cathode enabled by highly crumpled nitrogen-doped graphene sheets for high-energy-density lithium-sulfur batteries. Nano Lett 2016;16:864-70.
67. Hu C, Kirk C, Cai Q, et al. A high-volumetric-capacity cathode based on interconnected close-packed N-doped porous carbon nanospheres for long-life lithium-sulfur batteries. Adv Energy Mater 2017;7:1701082.
68. Hou TZ, Xu WT, Chen X, Peng HJ, Huang JQ, Zhang Q. Lithium bond chemistry in lithium-sulfur batteries. Angew Chem Int Ed 2017;56:8178-82.
69. Xu J, Su D, Zhang W, Bao W, Wang G. A nitrogen-sulfur co-doped porous graphene matrix as a sulfur immobilizer for high performance lithium-sulfur batteries. J Mater Chem A 2016;4:17381-93.
70. Hao GP, Tang C, Zhang E, et al. Thermal exfoliation of layered metal-organic frameworks into ultrahydrophilic graphene stacks and their applications in Li-S batteries. Adv Mater 2017;29:1702829.
71. Li L, Tu H, Wang J, et al. Electrocatalytic MOF-carbon bridged network accelerates Li+-solvents desolvation for high Li+ diffusion toward rapid sulfur redox kinetics. Adv Funct Mater 2023;33:2212499.
72. Seh ZW, Zhang Q, Li W, Zheng G, Yao H, Cui Y. Stable cycling of lithium sulfide cathodes through strong affinity with a bifunctional binder. Chem Sci 2013;4:3673-7.
73. Zhou W, Xiao X, Cai M, Yang L. Polydopamine-coated, nitrogen-doped, hollow carbon-sulfur double-layered core-shell structure for improving lithium-sulfur batteries. Nano Lett 2014;14:5250-6.
74. Qiao X, Wang C, Zang J, et al. Conductive inks composed of multicomponent carbon nanomaterials and hydrophilic polymer binders for high-energy-density lithium-sulfur batteries. Energy Stor Mater 2022;49:236-45.
75. Kong W, Sun L, Wu Y, et al. Binder-free polymer encapsulated sulfur-carbon nanotube composite cathodes for high performance lithium batteries. Carbon 2016;96:1053-9.
76. Gu PY, Zhao Y, Xie J, et al. Improving the performance of lithium-sulfur batteries by employing polyimide particles as hosting matrixes. ACS Appl Mater Interfaces 2016;8:7464-70.
77. Su D, Cortie M, Fan H, Wang G. Prussian blue nanocubes with an open framework structure coated with PEDOT as high-capacity cathodes for lithium-sulfur batteries. Adv Mater 2017;29:1700587.
78. Ding Z, Zhao D, Yao R, Li C, Cheng X, Hu T. Polyaniline@spherical ordered mesoporous carbon/sulfur nanocomposites for high-performance lithium-sulfur batteries. Int J Hydrogen Energy 2018;43:10502-10.
79. Zhang K, Li X, Yang Y, et al. High loading sulfur cathodes by reactive-type polymer tubes for high-performance lithium-sulfur batteries. Adv Funct Mater 2023;33:2212759.
80. Hu H, Cheng H, Liu Z, Li G, Zhu Q, Yu Y. In situ polymerized PAN-assisted S/C nanosphere with enhanced high-power performance as cathode for lithium/sulfur batteries. Nano Lett 2015;15:5116-23.
81. Chen CY, Peng HJ, Hou TZ, et al. A quinonoid-imine-enriched nanostructured polymer mediator for lithium-sulfur batteries. Adv Mater 2017;29:1606802.
82. Dong F, Peng C, Xu H, et al. Lithiated sulfur-incorporated, polymeric cathode for durable lithium-sulfur batteries with promoted redox kinetics. ACS Nano 2021;15:20287-99.
83. Zhao CX, Li XY, Zhao M, et al. Semi-immobilized molecular electrocatalysts for high-performance lithium-sulfur batteries. J Am Chem Soc 2021;143:19865-72.
84. He B, Li W, Zhang Y, et al. Paragenesis BN/CNTs hybrid as a monoclinic sulfur host for high rate and ultra-long life lithium-sulfur battery. J Mater Chem A 2018;6:24194-200.
85. Zhou S, Pei W, Zhao Y, Yang X, Liu N, Zhao J. Low-dimensional non-metal catalysts: principles for regulating p-orbital-dominated reactivity. NPJ Comput Mater 2021;7:186.
86. Lee BJ, Zhao C, Yu JH, et al. Development of high-energy non-aqueous lithium-sulfur batteries via redox-active interlayer strategy. Nat Commun 2022;13:4629.
87. Li L, Yu Y, Ye GJ, et al. Black phosphorus field-effect transistors. Nat Nanotechnol 2014;9:372-7.
88. Li L, Chen L, Mukherjee S, et al. Phosphorene as a polysulfide immobilizer and catalyst in high-performance lithium-sulfur batteries. Adv Mater 2017;29:1602734.
89. Liang J, Yin L, Tang X, et al. Kinetically enhanced electrochemical redox of polysulfides on polymeric carbon nitrides for improved lithium-sulfur batteries. ACS Appl Mater Interfaces 2016;8:25193-201.
90. Wen Y, Shen Z, Hui J, Zhang H, Zhu Q. Co/CoSe junctions enable efficient and durable electrocatalytic conversion of polysulfides for high-performance Li-S batteries. Adv Energy Mater 2023;13:2204345.
91. Li J, Shi K, Pan J, et al. Designing electrochemical nanoreactors to accelerate Li2S1/2 three-dimensional growth process and generating more Li2S for advanced Li-S batteries. Renewables 2023;1:341-52.
92. Xie Y, Ao J, Zhang L, et al. Multi-functional bilayer carbon structures with micrometer-level physical encapsulation as a flexible cathode host for high-performance lithium-sulfur batteries. Chem Eng J 2023;451:139017.
93. Liu Q, Wu Y, Li D, et al. Dilute alloying to implant activation centers in nitride electrocatalysts for lithium-sulfur batteries. Adv Mater 2023;35:e2209233.
94. Lu Y, Qin J, Shen T, et al. Hypercrosslinked polymerization enabled N-doped carbon confined Fe2O3 facilitating Li polysulfides interface conversion for Li-S batteries. Adv Energy Mater 2021;11:2101780.
95. Yu X, Chen W, Cai J, Lu X, Sun Z. Oxygen vacancy-rich MnO nanoflakes/N-doped carbon nanotubes modified separator enabling chemisorption and catalytic conversion of polysulfides for Li-S batteries. J Colloid Interface Sci 2022;610:407-17.
96. Wei Seh Z, Li W, Cha JJ, et al. Sulphur-TiO2 yolk-shell nanoarchitecture with internal void space for long-cycle lithium-sulphur batteries. Nat Commun 2013;4:1331.
97. Yu M, Ma J, Song H, et al. Atomic layer deposited TiO2 on a nitrogen-doped graphene/sulfur electrode for high performance lithium-sulfur batteries. Energy Environ Sci 2016;9:1495-503.
98. Fang M, Chen Z, Liu Y, et al. Design and synthesis of novel sandwich-type C@TiO2@C hollow microspheres as efficient sulfur hosts for advanced lithium-sulfur batteries. J Mater Chem A 2018;6:1630-8.
99. Rehman S, Tang T, Ali Z, Huang X, Hou Y. Integrated design of MnO2@Carbon hollow nanoboxes to synergistically encapsulate polysulfides for empowering lithium sulfur batteries. Small 2017;13:1700087.
100. Xu J, Zhang W, Chen Y, Fan H, Su D, Wang G. MOF-derived porous N-Co3O4@N-C nanododecahedra wrapped with reduced graphene oxide as a high capacity cathode for lithium-sulfur batteries. J Mater Chem A 2018;6:2797-807.
101. Zhou L, Li H, Wu X, et al. Double-shelled Co3O4/C nanocages enabling polysulfides adsorption for high-performance lithium-sulfur batteries. ACS Appl Energy Mater 2019;2:8153-62.
102. Chu R, Nguyen TT, Bai Y, Kim NH, Lee JH. Uniformly controlled treble boundary using enriched adsorption sites and accelerated catalyst cathode for robust lithium-sulfur batteries. Adv Energy Mater 2022;12:2102805.
103. Hua W, Shang T, Li H, et al. Optimizing the p charge of S in p-block metal sulfides for sulfur reduction electrocatalysis. Nat Catal 2023;6:174-84.
104. Dai X, Lv G, Wu Z, et al. Flexible hierarchical Co-doped NiS2@CNF-CNT electron deficient interlayer with grass-roots structure for Li-S batteries. Adv Energy Mater 2023;13:2300452.
105. Zhou G, Tian H, Jin Y, et al. Catalytic oxidation of Li2S on the surface of metal sulfides for Li-S batteries. Proc Natl Acad Sci USA 2017;114:840-5.
106. He J, Bhargav A, Manthiram A. High-performance anode-free Li-S batteries with an integrated Li2S-electrocatalyst cathode. ACS Energy Lett 2022;7:583-90.
107. Xie Y, Cao J, Wang X, et al. MOF-derived bifunctional Co0.85Se nanoparticles embedded in N-doped carbon nanosheet arrays as efficient sulfur hosts for lithium-sulfur batteries. Nano Lett 2021;21:8579-86.
108. Chen T, Ma L, Cheng B, et al. Metallic and polar Co9S8 inlaid carbon hollow nanopolyhedra as efficient polysulfide mediator for lithium-sulfur batteries. Nano Energy 2017;38:239-48.
109. Shi M, Liu Z, Zhang S, et al. A mott-schottky heterogeneous layer for Li-S batteries: enabling both high stability and commercial-sulfur utilization. Adv Energy Mater 2022;12:2103657.
110. Lin H, Yang L, Jiang X, et al. Electrocatalysis of polysulfide conversion by sulfur-deficient MoS2 nanoflakes for lithium-sulfur batteries. Energy Environ Sci 2017;10:1476-86.
111. You Y, Ye Y, Wei M, et al. Three-dimensional MoS2/rGO foams as efficient sulfur hosts for high-performance lithium-sulfur batteries. Chem Eng J 2019;355:671-8.
112. Hu L, Dai C, Lim JM, et al. A highly efficient double-hierarchical sulfur host for advanced lithium-sulfur batteries. Chem Sci 2018;9:666-75.
113. Feng Y, Zu L, Yang S, et al. Ultrahigh-content Co-P cluster as a dual-atom-site electrocatalyst for accelerating polysulfides conversion in Li-S batteries. Adv Funct Mater 2022;32:2207579.
114. Hu Z, Geng C, Wang L, Lv W, Yang Q. Revisiting the roles of carbon in the catalysis of lithium-sulfur batteries. Adv Energy Sustain Res 2024;5:2300148.
115. Yu X, Tian D, Li W, et al. One-pot synthesis of highly conductive nickel-rich phosphide/CNTs hybrid as a polar sulfur host for high-rate and long-cycle Li-S battery. Nano Res 2019;12:1193-7.
116. Mi Y, Liu W, Li X, Zhuang J, Zhou H, Wang H. High-performance Li-S battery cathode with catalyst-like carbon nanotube-MoP promoting polysulfide redox. Nano Res 2017;10:3698-705.
117. Ye Z, Jiang Y, Qian J, et al. Exceptional adsorption and catalysis effects of hollow polyhedra/carbon nanotube confined CoP nanoparticles superstructures for enhanced lithium-sulfur batteries. Nano Energy 2019;64:103965.
118. Huang S, Lim YV, Zhang X, et al. Regulating the polysulfide redox conversion by iron phosphide nanocrystals for high-rate and ultrastable lithium-sulfur battery. Nano Energy 2018;51:340-8.
119. Li S, Lin J, Chang B, et al. Implanting single-atom N2-Fe-B2 catalytic sites in carbon hosts to stabilize high-loading and lean-electrolyte lithium-sulfur batteries. Energy Storage Mater 2023;55:94-104.
120. Li Z, Li B, Yu C, Wang H, Li Q. Recent progress of hollow carbon nanocages: general design fundamentals and diversified electrochemical applications. Adv Sci 2023;10:e2206605.
121. Zhou J, Tang W, Shu C, et al. Well-defined metal-N4 sites coordinated defective carbon as efficient electrocatalysts for high performance lithium-sulfur batteries. Mater Today Energy 2022;30:101151.
122. Du Z, Chen X, Hu W, et al. Cobalt in nitrogen-doped graphene as single-atom catalyst for high-sulfur content lithium-sulfur batteries. J Am Chem Soc 2019;141:3977-85.
123. Yang JL, Yang P, Cai DQ, Wang Z, Fan HJ. Atomically dispersed Fe-N4 and Ni-N4 independent sites enable bidirectional sulfur redox electrocatalysis. Nano Lett 2023;23:4000-7.
124. Yu X, He B, Li W, Wu T, Chen X, Lu A. Multi-cavity carbon nanofiber film decorated with Co-Nx doped CNTs for lithium-sulfur batteries with high-areal-capacity. J Mater Chem A 2022;10:12168-76.
125. Zhou T, Lv W, Li J, et al. Twinborn TiO2 -TiN heterostructures enabling smooth trapping-diffusion-conversion of polysulfides towards ultralong life lithium-sulfur batteries. Energy Environ Sci 2017;10:1694-703.
126. Song Y, Zhao W, Kong L, et al. Synchronous immobilization and conversion of polysulfides on a VO2-VN binary host targeting high sulfur load Li-S batteries. Energy Environ Sci 2018;11:2620-30.
127. Wang B, Ren Y, Zhu Y, et al. Construction of Co3O4/ZnO heterojunctions in hollow N-doped carbon nanocages as microreactors for lithium-sulfur full batteries. Adv Sci 2023;10:e2300860.
Cite This Article
Export citation file: BibTeX | EndNote | RIS
OAE Style
Yu XF, Shao DY, Xu J, Cao J. Recent advances in carbon-based sulfur host materials for lithium-sulfur batteries. Microstructures 2024;4:2024030. http://dx.doi.org/10.20517/microstructures.2023.82
AMA Style
Yu XF, Shao DY, Xu J, Cao J. Recent advances in carbon-based sulfur host materials for lithium-sulfur batteries. Microstructures. 2024; 4(3): 2024030. http://dx.doi.org/10.20517/microstructures.2023.82
Chicago/Turabian Style
Xiao-Fei Yu, Dao-Yu Shao, Juan Xu, Jianyu Cao. 2024. "Recent advances in carbon-based sulfur host materials for lithium-sulfur batteries" Microstructures. 4, no.3: 2024030. http://dx.doi.org/10.20517/microstructures.2023.82
ACS Style
Yu, X.F.; Shao D.Y.; Xu J.; Cao J. Recent advances in carbon-based sulfur host materials for lithium-sulfur batteries. Microstructures. 2024, 4, 2024030. http://dx.doi.org/10.20517/microstructures.2023.82
About This Article
Special Issue
Copyright
Data & Comments
Data
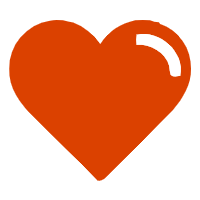
Comments
Comments must be written in English. Spam, offensive content, impersonation, and private information will not be permitted. If any comment is reported and identified as inappropriate content by OAE staff, the comment will be removed without notice. If you have any queries or need any help, please contact us at support@oaepublish.com.