Integrating Cu/CuxO ternary nanocomposites with multi-walled carbon nanotubes enabling a high-performance nonenzymatic amperometric glucose sensor
Abstract
We developed a new nonenzymatic amperometric glucose sensor by integrating a ternary nanocomposite,
Keywords
INTRODUCTION
Diabetes is a metabolic disorder syndrome featuring high blood glucose levels[1], making it one of the three biggest killers of human health worldwide, following cardiovascular disease and cancer. It is projected to affect 693 million people (aged 18-99) by 2045, a 54% increase compared to 2017[2]. As a result, developing rapid, accurate and convenient methods for detecting glucose levels[3,4] in human blood or serum is crucial for diagnosing and managing diabetes within clinical practice. In 1962, Clark and Updike elaborated the first enzyme electrodes and current-based enzyme sensors[5-7]. Even though as many as 85% of commercial glucose sensors are enzyme-based electrochemical biosensors[8], there has been an instability of this type of enzyme-based biosensors[9-11]. This instability is primarily due to the sensitivity of glucose oxidase to environmental conditions (e.g., temperature[12], humidity[13], and pH[14]). It is challenging to establish a reliable method toward glucose by enzyme-based electrochemical biosensors.
Additionally, the applications of enzyme-based biosensors are largely restricted due to issues such as complicated immobilization processes, sophisticated operating conditions, poor reproducibility, and prohibitive costs[3,15-17]. To surmount the aforementioned weaknesses, scholars proposed a nonenzymatic sensor based on the direct electrochemical reaction toward glucose, which has been rapidly developed. Previous studies have shown that this nonenzymatic sensor relies on the electrical signal produced during the glucose oxidation on the surface of the electrode. The electrocatalytic activity of electrode materials is the key aspect influencing glucose sensing sensitivity and selectivity[8].
To date, noble metals and their oxides (e.g., Pt[18], Pd[19], Au, Ag, and various alloy particles[20,21]) have been utilized in the construction of nonenzymatic biosensors for detecting glucose. While they possess superior electrocatalytic properties for glucose oxidation, they are susceptible to contamination through chemisorption of intermediates or chloride adsorption[22]. The stability and sensitivity of the sensors are reduced when they are modified with noble metals or their oxides, limiting their widespread use in glucose detection. Transition metals and their oxides, such as Ni[23], Cu[21], MnO2[24], CuO[25], Cu2O[26], Co3O4[27], and ZnO[28], have demonstrated increasing application potential on account of resourceful, biocompatible, relatively inexpensive, and excellent glucose sensing capability. Among these, copper and its oxides exhibit attractive application prospects due to their low cost and outstanding catalytic performance for various reactions. For quite some time, they have been capable of catalyzing the electrochemical oxidation of glucose. For example, Cu[21],
Furthermore, alloys, nanocomposite materials consisting of bimetallic alloy and their oxides, metal/metal oxide, and 1D or 2D carbon nanomaterials tend to manifest synergistic effects from the constituent metals[30]. The large surface area of nanocomposite facilitates the exposure of numerous electrochemically active sites for the glucose reactions[31]. Fan et al. demonstrated that a bimetallic alloy nanoparticles-modified 3D graphene nanocomplex, Au-Ag/3D graphene, exhibited excellent electrocatalytic properties towards glucose[32]. Studies on the nanocomposite of copper oxide anchored polyaniline to modify the electrode by Sudharsan et al. have shown superior electrochemical determination of chlorpyrifos[33]. In contrast, multi-walled carbon nanotubes (MWCNTs) are gaining recognition as promising electrode materials due to their hollow tubular nanostructure, high surface area, remarkable electrical conductivity, exceptional stability, and promising potential for glucose detection[34]. To enhance the electrical conductivity of copper oxides and improve the stability and dispersion of copper and its oxides, researchers have deposited them on various substrates[21,26] using MWCNTs as a representative example. Most studies have focused on two-phase composites of metals and their oxides[20]. Building on the above research, integrating triple materials could be a compelling option for nonenzymatic glucose detection. The different properties of the various materials and sufficient exposure of active metal species on MWCNT surfaces will have multiple promotions on electrochemical activity.
Herein, Cu/CuxO ternary nanocomposites have been successfully modified onto the surface of MWCNTs by the electroless plating and further heat treatment to obtain Cu/CuxO@MWCNTs nanocomposites. These acquired nanocomposites demonstrate superior nonenzymatic electrocatalytic activity towards glucose. By taking advantage of their high-performance catalytic activity for glucose oxidation, we developed an electrochemical biosensor for glucose based on Cu/CuxO@MWCNTs nanocomposites-modified electrodes and investigated their electrocatalytic application. A schematic diagram illustrating the electrochemical sensing using Cu/CuxO@MWCNTs ternary nanocomposites-based sensors is presented in Scheme 1.
MATERIALS AND METHODS
Chemicals and materials
CuSO4·5H2O was acquired from Yaohua Chemical Reagent Co., Ltd., Tianjin, China. NaOH was gained from the Mainland Chemical Reagent Factory (Tianjin, China). Glucose was obtained from Ruijinte Chemical Co., Ltd. (Tianjin, China). The serum of healthy volunteers was sourced from a hospital in Nanjing. All aqueous solutions were prepared and diluted with deionized water from Hitech-Kflow (Shanghai Hitech Instruments Co. Ltd., China).
Pretreatment of initial MWCNTs and synthesis of the Cu/CuxO@MWCNTs nanocomposites
The initial MWCNTs were purchased from Shenzhen Nanotech Port Co., Ltd., Shenzhen, China. The purification of MWCNTs was approached as reported in our previous study[35]. In brief, prior to use, the initial MWCNTs were dispersed in a 3 M solution of nitric acid and treated with the help of ultrasonication for 4 h. Subsequently, the solution was cleaned with deionized water and filtered using a vacuum pump; the MWCNTs slurry collected by filtration was then dried.
The synthesis of Cu/CuxO@MWCNTs ternary nanocomposites was carried out as follows: sensitization → activation → reduction treatment → plating → post-plating. In order to acquire the homogeneity and integrity of the MWCNTs, the entire process was operated under ultrasonic environment. The formulations and operating conditions regarding to the Cu/CuxO@MWCNTs ternary nanocomposites preparation are displayed in Table 1.
The formulations and operating conditions of synthesizing Cu/CuxO@MWCNTs
Procedure | Chemicals and operating conditions | Parameters |
Sensitization | SnCl2 | 5 g |
T | 25 °C | |
Time | 2-2.5 mins | |
Activation | PdCl2 | 0.1 g/L |
T | 25 °C | |
Time | 2-2.5 mins | |
Reduction | NaH2PO2 | 15 g/L |
Plating | NaOH | 7 g/L |
NaKC4H4O6·4H2O | 25 g/L | |
CuSO4·5H2O | 5 g/L | |
Methanal | 12 mL | |
T | 30 °C | |
Time | 2-2.5 min | |
pH | 13-14 | |
Post-plating | Oxidation of Cu | 220 °C, 4 h |
Characterization
Analyses employing a transmission electron microscope (TEM) and energy dispersive X-ray spectroscopy (EDS) were conducted using the Talos F200X G2 TEM (Thermo Scientific, USA). X-ray diffractometer (XRD) examination was performed by an X’Pert Pro (PANalytical B.V., Netherlands) using Cu Ka radiation. X-ray photoelectron spectroscopy (XPS) was analyzed using a Thermofisher Escalab Xi+ (Thermofisher, USA) by Al Kα radiation.
Preparation of the glucose electrochemical sensor and electrochemical measurements
The glassy carbon electrodes (GC, diameters of 3 mm) were used as a substrate for the working electrode fabrication. They were mechanically polished using emery paper, followed by polishing of 0.3 and 0.05 μm alumina powder with a polishing cloth, and were alternately ultrasonic bath cleaning for 3 min with pure ethanol and deionized water. Subsequently, these electrodes were modified by dropping 6 μL of the
Additionally, a CHI 660E electrochemical analyzer (Chenhua, Shanghai) was collected to carry out electrochemical experimentations in a two-compartment electrochemical cell at room temperature. In this work, the GC, platinum, and Ag/AgCl electrodes (KCl-saturated) were utilized as the substrates for the fabricated working, counter, and reference electrodes, respectively. For electrochemical impedance spectroscopy (EIS) measurements, the ac voltage was set as 5 mV at an open circuit potential of 0.23 V. The amperometric i-t tests were studied by adding various glucose concentrations consecutively at 0.45 V.
RESULTS AND DISCUSSION
Characterization of as-synthesized samples
After purification, we observed the morphology of the raw MWCNTs with TEM and characterized their functional groups using Raman spectra. Considering that the surface of MWNT contains residual metal catalytic particles originating from the production process, we subject MWCNTs to acid purification prior to preparing the composites, as previously reported[35]. The TEM images and Raman spectra of raw and purified MWCNTs composites are investigated [Figure 1A-C]. Comparing the TEM images exhibited in Figure 1A and B, MWCNTs, before and after purification, exhibit a hollow tubular structure. Additionally, the wall dissolution breakage or damage phenomenon is not detected in MWCNTs following purification. Furthermore, the purification treatment significantly improved the agglomerated degree of initial MWCNTs. In Figure 1A, impurities exist in initial MWCNTs (catalyst metal nanoparticles and amorphous carbons[36]). The catalyst metal nanoparticles and amorphous carbon impurities are observed to decrease significantly in Figure 1B. This decrease is mainly attributed to the removal of metal catalyst impurities present in the initial MWCNTs, as they are transformed into metal oxides or hydroxides during the acid purification and thus dissolved in the solution. Meanwhile, since the structural stability of MWCNTs is much greater than that of carbon impurities, the carbon impurities are easier to remove by oxidation in acid solution[36-38]. Acid purification not only removes impurities but also introduces oxygen-containing functional groups [Supplementary Figure 1] into the surface defects and tube ends of MWCNTs[36]. The impact of these functional groups on MWCNT dispersion in the plating solution and the performance of the modified electrode is significant. Therefore, an acid purification treatment of purchased MWCNTs is both essential and efficient.
Figure 1. (A and B) TEM images of the initial MWCNTs and the purified MWCNTs composites. (C) Raman spectra of the raw MWCNTs and the purified MWCNTs composites at λ = 532 nm. (D and E) TEM images of the Cu/CuxO@MWCNTs nanocomposites. (F) HRTEM images of the Cu/CuxO@MWCNTs nanocomposites and the corresponding SAED pattern (insert). (G) TEM-EDX of C, Cu, O, Pd, P, Cl, and Sn elements for the Cu/CuxO@MWCNTs nanocomposites. (H) EDX spectra of the Cu/CuxO@MWCNTs nanocomposites.
Figure 1C records the Raman spectra for MWCNTs. As investigated in Figure 1C, the D-band located near 1,342 cm-1 is mainly derived from the presence of structural defects or disordered structural positions in the MWCNTs associated with the amorphous or disordered carbon. The G-band located near 1,573 cm-1 for initial MWCNTs and 1,580 cm-1for purified MWCNTs originating from the doubly condensed in-plane optical vibrations of sp2 hybridized carbon[39], which is consistent with former studies (G-band at
The purified MWCNTs were used to prepare Cu/CuxO/MWCNTs ternary nanocomposites, and the morphology and composition characterization was shown in Figure 1D-H. As depicted in Figure 1D and E, the surface of the MWCNTs owns a number of evenly distributed nanoparticles, which increases the specific surface area of the MWCNTs. The increased surface area of the Cu/CuxO@MWCNTs may enhance the direct electron transfer between the Cu/CuxO@MWCNTs and the GC electrode compared with the direct electron transfer between MWCNTs and the bare GC electrode. From the high-resolution transmission electron microscopy (HRTEM) images [Figure 1F], the lattice spacings of these tubular structures are analyzed as 0.339 nm, which is close to the lattice spacing (0.34 nm) of crystal plane (111) of MWCNTs. Herein, the marked lattice spacings of 0.208, 0.246, and 0.212 nm ascribed to the (111) crystal plane of Cu, the (111) crystal plane of Cu2O[15,31], and the (200) crystal plane of CuO, respectively. The analysis demonstrates that the as-prepared MWCNTs-based composites are ternary nanocomposites, consisting of Cu, Cu2O, and CuO phases. In addition, the corresponding selected area electron diffraction (SAED) pattern (insert for Figure 1F) investigated that the composites exhibit superior polycrystallinity, which is consistent with HRTEM analysis. Figure 1G illustrates the scanning electron microscopy-energy-dispersive X-ray spectrometry (TEM-EDX) elemental mapping of the selected area and the distribution of each component. The analysis reveals that the selected area has a uniform distribution of the elements C, Cu, and O, with no elemental segregation or aggregation. Almost no residue of the impurities, such as Pd, P, Cl, and Sn, may be retained during the electroless plating process. From Figure 1H, the quantitative analysis of the EDS results presents mainly C, O, and Cu elements, confirming the successful construct of the Cu and CuxO onto MWCNTs. Above all, the phase composition of the Cu/CuxO@MWCNTs nanocomposites is fully identified as MWCNTs, Cu, and CuxO. The XRD pattern of the synthesized Cu/CuxO@MWCNTs
Figure 2. (A) XRD patterns of the Cu/CuxO@MWCNTs nanocomposites. The XPS survey scan (B) and the Cu 2p spectrum (C) of the Cu/CuxO@MWCNTs nanocomposites.
As recorded in Figure 2B and C, the exact composition and valence state of the Cu/CuxO@MWCNTs nanocomposites are further identified through XPS. The high-resolution XPS spectrum of Cu 2p clearly shows Cu 2p1/2, 2p3/2 and satellite peaks. After fitting, the Cu 2p1/2 peak with 952.5 and 954.6 eV corresponds to Cu+[41] and Cu2+[42], respectively. The Cu 2p3/2 peak with 932.6 and 934.6 eV corresponds to Cu0[43] and
Electrochemical behavior and performance of the electrode
Before developing an electrochemical method for nonenzymatic glucose sensing, it is required to electro-activate the Cu/CuxO@MWCNTs. We process the electrochemical activation by conducting 40-cycle successive cyclic voltammograms (CVs) at a 50 mV·s-1 scan rate within potential ranging from -0.90 to
Figure 3. (A) The typical cyclic voltammograms of the Cu/CuxO@MWCNTs-modified GC electrode, 0.1 M NaOH, a scanning rate of
Peak 1
Peak 2
Peak 3
Peak 4
Peak 5
Peak 6
Peak 7
The above analysis redox results further confirm the successful synthesis of Cu/CuxO@MWCNTs nanocomposites. After accomplishing the electrochemical activation, the constructed
Herein, to further study this excellent electron transferring capability, cyclic voltammetry is conducted to explore the electrocatalytic activity of the modified electrode towards glucose. As shown in Figure 3D, in the presence of 1.0 mM glucose, glucose oxidation on the GC electrode seems to be very sluggish oxidation with a tailed oxidation, indicating that the glucose electrooxidation on the GC electrode has very low electrochemical kinetics. The oxidation potential of glucose on the MWCNTs-base electrode appears at
To obtain the kinetics of the electrochemical reaction of glucose at the Cu/CuxO@MWCNTs-modified GC electrode, the CVs of the Cu/CuxO@MWCNTs-modified GC electrodes are further investigated for the influence of scan rate (v) on the peak currents (Ip) in Figure 4A. As the scan rates increase, the glucose oxidation peak current at 0.37 V of the Cu/CuxO@MWCNTs-modified GC electrodes also rises. As shown in Figure 4B, the Ip is linearly related to v, indicating that the electrochemical process of glucose at
Amperometric response of the electrode
We have systemically examined and demonstrated the effective electrocatalytic activity of the
Figure 5. (A) Amperometric curve of the response of Cu/CuxO@MWCNTs-modified GC electrode to glucose measured at 0.45 V. The insert is the i-t curve of the response of the electrode to glucose with consecutive addition of glucose ranging from 0.005 to 0.5 μM. (B) Corresponding calibration plots of Ivs. C, n = 3.
Table 2 compares the results obtained from this work with those of previously reported nonenzymatic glucose sensors. The result reveals that the sensitivity, LOD, and linear detection range are better than those of most reported sensors.
Comparison of the electrochemical performances between Cu/CuxO@MWCNTs-based and other nonenzymatic glucose sensors
Electrodes | Sensitivity μA·mM-1·cm-2 | Linear range (μM) | Detection limit (μM) | Ref. |
CuO@MCM-41/Ni foama | 0.01723 | 83-1,500 | 0.016 | [51] |
NiO/C@rGO/GCEb | - | 1-1,115 | 0.658 | [52] |
Ni/NiO/NC/GCEc | 76.03 | 0.6-8,600 | 0.2 | [53] |
ZnO TPs/MXene/GOxd | 29 | 50-700 | 17 | [54] |
Co-PM-NDGPC/SPEe | 427.85 | 30-1,071 | 7.8 | [55] |
Pt-MWCNT/SFf | 8.09 | 100-3,750 | 0.1 | [56] |
GCE/CNT/MoS2/NiNPsg | - | 50-650 | 0.197 | [57] |
Cu-BC1/PGEh | 6214.4 | 1,000-5,000 | 0.8 | [58] |
MoS2@CuCo2O4/GCEi | - | 0.5-393 | 0.5 | [59] |
Cu/CuxO@MWCNTs/GC | 257.14 | 0.005-6,000 | 0.003 | This work |
Selectivity, repeatability, and reproducibility
High selectivity, repeatability, and reproducibility are crucial requirements for practical glucose detection. In blood, glucose usually coexists with small amounts of organic molecules [i.e., dopamine (DA), ascorbic acid (AA), uric acid (UA), 5-Hydroxytryptamine (5-HT) and 3,4-Dihydroxyphenylacetic acid (DOPAC)]. Whereas these organic substances also generate corresponding current signals during the actual detection process, which can influence the glucose detection results. What is more, copper-containing materials-based electrodes are susceptible to chlorine ion poisoning (Cl-). Therefore, it is required that the prepared electrode materials must have great anti-interference ability. In order to investigate the interference rejection capability of the Cu/CuxO@MWCNTs-modified electrodes, 10 μM DA, AA, UA, 5-HT, DOPAC,
Application analysis in serum samples
To assess its analytical utility, the constructed electrochemical sensor is employed for the glucose determination in real samples. Before detection, all of the samples are diluted with 0.1 M NaOH by 50-fold, which can reduce the matrix effect of the serum samples. To evaluate the correctness of the results, a certain amount of glucose is spiked to the sample and the electrochemical results are compared with those of a commercial blood glucose analyzer, yielding consistent results. The results obtained from three samples are studied [Table 3]. The recovery rates of the spiked samples are measured in the range of 100.54% to 102.4% with an RSD of 5.60 ± 1.55 for glucose. This indicates that the developed sensor is both accurate and precise.
Determination results of glucose in human serum samples (n = 3)
Sample | Concentration of glucose (mM) | Added (mM) | C (mM) | Recovery (%) | Mean RSD (mM) |
1 | 5.0 | 0.5 | 5.55 | 100.5 | |
2 | 5.0 | 0.5 | 5.55 | 102.4 | 5.60 ± 1.55 |
3 | 5.1 | 0.5 | 5.70 | 101.8 |
CONCLUSIONS
The Cu/CuxO@MWCNTs ternary nanocomposites were used as an efficient electrocatalyst to construct an electrochemical nonenzymatic sensor toward glucose. The Cu/CuxO@MWCNTs ternary nanocomposites were prepared through the electroless plating process and heat treatment. The Cu/CuxO@MWCNTs-modified GC electrode, based on Cu/CuxO@MWCNTs ternary nanocomposites, exhibited increased current response to glucose, demonstrating rapid response, excellent linearity, and superior sensitivity, repeatability and reproducibility. The nonenzymatic electrochemical sensor demonstrated high selectivity and sensitivity for glucose detection in human serum samples.
DECLARATIONS
Authors’ contributions
Conceptualization, investigation, methodology, data curation, formal analysis, writing - original draft:
Investigation, validation, supervision: Zhang Y
Writing - original draft: Zhang Z
Validation, supervision: Chen Y
Writing - review & editing, Formal analysis: Huang X
Methodology, formal analysis: Mou H, Deng Z
Conceptualization, supervision, writing - review & editing: Li Z
Investigation, methodology, writing - review & editing, supervision: Xu X
Formal analysis, supervision, project administration, writing - review & editing, resources: Zheng W
Availability of data and materials
Not applicable.
Financial support and sponsorship
None.
Conflicts of interest
All authors declared that there are no conflicts of interest.
Ethical approval and consent to participate
Not applicable.
Consent for publication
Not applicable.
Copyright
© The Author(s) 2024.
Supplementary Materials
REFERENCES
1. Golsanamlou Z, Mahmoudpour M, Soleymani J, Jouyban A. Applications of advanced materials for non-enzymatic glucose monitoring: from invasive to the wearable device. Crit Rev Anal Chem 2023;53:1116-31.
2. Cho NH, Shaw JE, Karuranga S, et al. IDF diabetes Atlas: global estimates of diabetes prevalence for 2017 and projections for 2045. Diabetes Res Clin Pract 2018;138:271-81.
3. Shen L, Liang Z, Chen Z, et al. Reusable electrochemical non-enzymatic glucose sensors based on Au-inlaid nanocages. Nano Res 2022;15:6490-9.
4. Bollella P. Enzyme-based amperometric biosensors: 60 years later … Quo Vadis? Anal Chim Acta 2022;1234:340517.
5. Clark LC Jr, Lyons C. Electrode systems for continuous monitoring in cardiovascular surgery. Ann N Y Acad Sci 1962;102:29-45.
7. Karyakin AA. Glucose biosensors for clinical and personal use. Electrochem Commun 2021;125:106973.
8. Vashist SK. Non-invasive glucose monitoring technology in diabetes management: a review. Anal Chim Acta 2012;750:16-27.
9. Sun Y, Shu T, Ma J, et al. Rational design of ZIF-8 for constructing luminescent biosensors with glucose oxidase and AIE-type gold nanoclusters. Anal Chem 2022;94:3408-17.
10. Kucherenko IS, Soldatkin OO, Kucherenko DY, Soldatkina OV, Dzyadevych SV. Advances in nanomaterial application in enzyme-based electrochemical biosensors: a review. Nanoscale Adv 2019;1:4560-77.
11. Du Y, Zhang X, Liu P, Yu DG, Ge R. Electrospun nanofiber-based glucose sensors for glucose detection. Front Chem 2022;10:944428.
12. Morshed J, Nakagawa R, Hossain MM, Nishina Y, Tsujimura S. Disposable electrochemical glucose sensor based on water-soluble quinone-based mediators with flavin adenine dinucleotide-dependent glucose dehydrogenase. Biosens Bioelectron 2021;189:113357.
13. Tutel Y, Koylan S, Tunca S, Unalan HE. Nanometer-thick Mn:NiO and Co:NiO films for high performance nonenzymatic biosensors. ACS Appl Nano Mater 2021;4:13871-83.
14. Chao D, Chen J, Dong Q, Wu W, Qi D, Dong S. Ultrastable and ultrasensitive pH-switchable carbon dots with high quantum yield for water quality identification, glucose detection, and two starch-based solid-state fluorescence materials. Nano Res 2020;13:3012-8.
15. Feng T, Yu C, Manaye Kabtamu D, Bu L, Li F, Wang Y. Cu2O nanowires with exposed {111} facet for nonenzymatic detection of glucose in complex biological fluids. Chem Eng J 2022;429:132267.
16. Zhou Y, Hu Q, Yu F, et al. A metal-organic framework based on a nickel bis(dithiolene) connector: synthesis, crystal structure, and application as an electrochemical glucose sensor. J Am Chem Soc 2020;142:20313-7.
17. Cohen R, Cohen Y, Mukha D, Yehezkeli O. Oxygen insensitive amperometric glucose biosensor based on FAD dependent glucose dehydrogenase co-entrapped with DCPIP or DCNQ in a polydopamine layer. Electrochim Acta 2021;367:137477.
18. Dong L, Ren S, Zhang X, Yang Y, Wu Q, Lei T. In-situ synthesis of Pt nanoparticles/reduced graphene oxide/cellulose nanohybrid for nonenzymatic glucose sensing. Carbohydr Polym 2023;303:120463.
19. Ghanam A, Haddour N, Mohammadi H, Amine A, Sabac A, Buret F. A membrane-less glucose/O2 non-enzymatic fuel cell based on bimetallic Pd-Au nanostructure anode and air-breathing cathode: towards micro-power applications at neutral pH. Biosens Bioelectron 2022;210:114335.
20. Wang Y, Kong J, Xue R, et al. Highly stable, stretchable, and transparent electrodes based on dual-headed Ag@Au core-sheath nanomatchsticks for non-enzymatic glucose biosensor. Nano Res 2023;16:1558-67.
21. Edet HO, Louis H, Godwin UC, Adalikwu SA, Agwamba EC, Adeyinka AS. Single-metal (Cu, Ag, Au) encapsulated gallium nitride nanotube (GaNNT) as glucose nonenzymatic nanosensors for monitoring diabetes: perspective from DFT, visual study, and MD simulation. J Mol Liq 2023;384:122209.
22. Dai Z, Yang A, Bao X, Yang R. Facile non-enzymatic electrochemical sensing for glucose based on Cu2O-BSA nanoparticles modified GCE. Sensors 2019;19:2824.
23. Chen J, Liu G, Xiao Q, et al. Glucose biosensors based on pinecone-shaped Au and Ni nanoparticle composite microelectrodes. ACS Appl Nano Mater 2022;5:13319-31.
24. Meng A, Hong X, Zhang Y, et al. A free-standing flexible sensor MnO2-Co/rGO-CNT for effective electrochemical hydrogen peroxide sensing and real-time cancer biomarker assaying. Ceram Int 2023;49:2440-50.
25. Hussain MH, Fook LP, Sanira Putri MK, Tan HL, Abu Bakar NF, Radacsi N. Advances on ultra-sensitive electrospun nanostructured electrochemical and colorimetric sensors for diabetes mellitus detection. Nano Mater Sci 2021;3:321-43.
26. Alanazi N, Selvi Gopal T, Muthuramamoorthy M, et al. Cu2O/MXene/rGO ternary nanocomposites as sensing electrodes for nonenzymatic glucose sensors. ACS Appl Nano Mater 2023;6:12271-81.
27. Yang M, Jeong JM, Lee KG, Kim DH, Lee SJ, Choi BG. Hierarchical porous microspheres of the Co3O4@graphene with enhanced electrocatalytic performance for electrochemical biosensors. Biosens Bioelectron 2017;89:612-9.
28. Chavez-Urbiola IR, Reséndiz-Jaramillo AY, Willars-Rodriguez FJ, et al. Glucose biosensor based on a flexible Au/ZnO film to enhance the glucose oxidase catalytic response. J Electroanal Chem 2022;926:116941.
29. Zhang X, Wang L, Ji R, Yu L, Wang G. Nonenzymatic glucose sensor based on Cu-Cu2S nanocomposite electrode. Electrochem Commun 2012;24:53-6.
30. Yang Y, Li P, Zheng X, et al. Anion-exchange membrane water electrolyzers and fuel cells. Chem Soc Rev 2022;51:9620-93.
31. Yu Z, Wu H, Xu Z, Yang Z, Lv J, Kong C. Wearable noninvasive glucose sensor based on CuxO NFs/Cu NPs Nanocomposites. Sensors 2023;23:695.
32. Fan L, Wu R, Patel V, Huang JJ, Selvaganapathy PR. Solid-state, reagent-free and one-step laser-induced synthesis of graphene-supported metal nanocomposites from metal leaves and application to glucose sensing. Anal Chim Acta 2023;1264:341248.
33. Sudharsan S, Rajaram R, Kumar S, Swaminathan P, Ramanujam K, Neelakantan L. Copper oxide anchored polyaniline modified glassy carbon electrode: a new sensor platform for the amperometric determination of chlorpyrifos. Electrochim Acta 2023;471:143305.
34. Zhu T, Zhang Y, Luo L, Zhao X. Facile fabrication of NiO-decorated double-layer single-walled carbon nanotube buckypaper for glucose detection. ACS Appl Mater Interfaces 2019;11:10856-61.
35. Deng Z, Zhao L, Mu H, et al. High selective property of gelatin/MWCNTs functionalized carbon fiber microelectrode: toward real-time monitoring of ascorbate. J Electroanal Chem 2022;914:116315.
36. Zhang XX, Deng CF, Xu R, Wang DZ. Oxidation resistance of multi-walled carbon nanotubes purified with sulfuric and nitric acids. J Mater Sci 2007;42:8377-80.
37. Hsu HL, Jehng JM, Sung Y, Wang LC, Yang SR. The synthesis, characterization of oxidized multi-walled carbon nanotubes, and application to surface acoustic wave quartz crystal gas sensor. Mater Chem Phys 2008;109:148-55.
38. Liu CK, Wu JM, Shih HC. Application of plasma modified multi-wall carbon nanotubes to ethanol vapor detection. Sens Actuat B Chem 2010;150:641-8.
39. Hu X, Zuo S, Yang M. High-yield preparation of ultrahigh-hydrophilicity MWCNTs by highly controllable oxidation with concentrated HNO3/HClO4 mixture. Appl Surf Sci 2023;626:157200.
40. Liu X, Wang C, Huang Z, Zhu P. Electrospun multiwall nanotubes-loaded polyethylene glycol/polyacrylonitrile composite form-stable nanofibers for thermal energy storage. J Energy Stor 2023;66:107458.
41. Jolley JG, Geesey GG, Hankins MR, Wright RB, Wichlacz PL. Auger electron and X-ray photoelectron spectroscopic study of the biocorrosion of copper by alginic acid polysaccharide. Appl Surf Sci 1989;37:469-80.
42. Jiang J, Liu XX, Han J, Hu K, Chen JS. Self-supported sheets-on-wire CuO@Ni(OH)2/Zn(OH)2 nanoarrays for high-performance flexible quasi-solid-state supercapacitor. Processes 2021;9:680.
43. Santra AK, Rao CNR. Surface alloy formation in Pd/Ag, Cu/Au and Ni/Au bimetallic overlayers. Appl Surf Sci 1995;84:347-50.
44. Parmigiani F, Sangaletti L. The Cu2p X-ray photoelectron core-lines in copper oxide based high temperature superconductors. J Electron Spectrosc Relat Phenom 1994;66:223-39.
45. Liu S, Hui KS, Hui KN. Flower-like copper cobaltite nanosheets on graphite paper as high-performance supercapacitor electrodes and enzymeless glucose sensors. ACS Appl Mater Interfaces 2016;8:3258-67.
46. Le WZ, Liu YQ. Preparation of nano-copper oxide modified glassy carbon electrode by a novel film plating/potential cycling method and its characterization. Sens Actuat B Chem 2009;141:147-53.
47. Paixão TRLC, Corbo D, Bertotti M. Amperometric determination of ethanol in beverages at copper electrodes in alkaline medium. Anal Chim Acta 2002;472:123-31.
48. Hou L, Zhao H, Bi S, Xu Y, Lu Y. Ultrasensitive and highly selective sandpaper-supported copper framework for non-enzymatic glucose sensor. Electrochim Acta 2017;248:281-91.
49. Marioli JM, Kuwana T. Electrochemical characterization of carbohydrate oxidation at copper electrodes. Electrochim Acta 1992;37:1187-97.
50. Zhang Y, Su L, Manuzzi D, et al. Ultrasensitive and selective non-enzymatic glucose detection using copper nanowires. Biosens Bioelectron 2012;31:426-32.
51. Zohaa, Arif D, Hassan M, et al. An electrochemical sensor based on copper oxide nanoparticles loaded on a mesoporous MCM-41 for non-enzymatic detection of glucose. Ceram Int 2024;50:12614-20.
52. Imanzadeh H, Amiri M, Nozari-Asbemarz M. A novel NiO/C@rGO nanocomposite derived from Ni(gallate): a non-enzymatic electrochemical glucose sensor. Microchem J 2024;199:110106.
53. Liang H, Luo Y, Xiao Y, Chen R, Wang L, Song Y. Ni/NiO/carbon derived from covalent organic frameworks for enzymatic-free electrochemical glucose sensor. Ceram Int 2024;50:977-84.
54. Myndrul V, Coy E, Babayevska N, et al. MXene nanoflakes decorating ZnO tetrapods for enhanced performance of skin-attachable stretchable enzymatic electrochemical glucose sensor. Biosens Bioelectron 2022;207:114141.
55. Elancheziyan M, Prakasham K, Eswaran M, et al. Eco-friendly fabrication of nonenzymatic electrochemical sensor based on cobalt/polymelamine/nitrogen-doped graphitic-porous carbon nanohybrid material for glucose monitoring in human blood. Environ Res 2023;223:115403.
56. Kuang D, Yu W, Liu J, Yin Y, Wang C. Flexible MWCNT/silk fibroin film decorated with Pt NPs for electrochemical glucose sensors. Microchem J 2023;191:108760.
57. Fall B, Sall DD, Hémadi M, et al. Highly efficient non-enzymatic electrochemical glucose sensor based on carbon nanotubes functionalized by molybdenum disulfide and decorated with nickel nanoparticles (GCE/CNT/MoS2/NiNPs). Sens Actuat Rep 2023;5:100136.
58. Larasati LD, Ateş A, Oskay KO. Direct co-deposition of binder-free Cu-biochar-based nonenzymatic disposable sensing element for electrochemical glucose detection. Surf Interfaces 2023;42:103355.
Cite This Article
Export citation file: BibTeX | EndNote | RIS
OAE Style
Xi W, Zhang Y, Zhang Z, Chen Y, Huang X, Mou H, Deng Z, Li Z, Xu X, Zheng W. Integrating Cu/CuxO ternary nanocomposites with multi-walled carbon nanotubes enabling a high-performance nonenzymatic amperometric glucose sensor. Microstructures 2024;4:2024028. http://dx.doi.org/10.20517/microstructures.2023.79
AMA Style
Xi W, Zhang Y, Zhang Z, Chen Y, Huang X, Mou H, Deng Z, Li Z, Xu X, Zheng W. Integrating Cu/CuxO ternary nanocomposites with multi-walled carbon nanotubes enabling a high-performance nonenzymatic amperometric glucose sensor. Microstructures. 2024; 4(3): 2024028. http://dx.doi.org/10.20517/microstructures.2023.79
Chicago/Turabian Style
Weiyan Xi, Yupeng Zhang, Zhijia Zhang, Yu Chen, Xuanyuan Huang, Hongwei Mou, Zhaoxue Deng, Zhen Li, Xiaoxue Xu, Wei Zheng. 2024. "Integrating Cu/CuxO ternary nanocomposites with multi-walled carbon nanotubes enabling a high-performance nonenzymatic amperometric glucose sensor" Microstructures. 4, no.3: 2024028. http://dx.doi.org/10.20517/microstructures.2023.79
ACS Style
Xi, W.; Zhang Y.; Zhang Z.; Chen Y.; Huang X.; Mou H.; Deng Z.; Li Z.; Xu X.; Zheng W. Integrating Cu/CuxO ternary nanocomposites with multi-walled carbon nanotubes enabling a high-performance nonenzymatic amperometric glucose sensor. Microstructures. 2024, 4, 2024028. http://dx.doi.org/10.20517/microstructures.2023.79
About This Article
Copyright
Data & Comments
Data
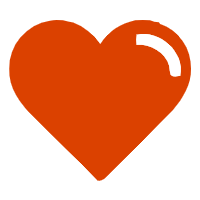
Comments
Comments must be written in English. Spam, offensive content, impersonation, and private information will not be permitted. If any comment is reported and identified as inappropriate content by OAE staff, the comment will be removed without notice. If you have any queries or need any help, please contact us at support@oaepublish.com.