Superior single-atom and single-cluster catalysts towards electrocatalytic nitrogen reduction reactions: a theoretical perspective
Abstract
The traditional Haber-Bosch process for ammonia synthesis is both energy-intensive and capital-demanding. Electrocatalytic nitrogen reduction reaction (NRR) has emerged as a promising, sustainable alternative, with recent advantages highlighting its potential. Single-atom catalysts (SACs) and single-cluster catalysts (SCCs) are promising catalysts for NRR due to their atomically dispersed active sites, maximized atom utilization, and distinctive coordination and electronic structures, all of which facilitate mechanism insights at the atomic level. Benefiting from efficient atom utilization, for example, the ammonia yield rate on Au1/C3N4 is roughly 22.5 times as high as that of supported Au nanoparticles, fully demonstrating the significant advantages of SACs over nanoparticles. In this review, we focus on the theoretical progress in SACs and SCCs for electrocatalyzing NRR, including nitrogenase-like bio-inspired catalysts and other metal-based catalysts. We further examine key adsorption energy and electronic descriptors that enhance our understanding of catalytic performance. Finally, we discuss the remaining challenges and future directions for advancing SACs and SCCs in electrocatalytic NRR applications.
Keywords
INTRODUCTION
Though atomic dispersion of transition metal (TM) had been recognized for its excellent catalytic activity[1-3], the term “single-atom catalysts (SACs)” was formally coined by Zhang et al. in 2011[4]. SACs exhibit superior catalytic performance across a wide range of reactions compared with traditional catalysts, mainly due to their unsaturated coordination and unique electronic structures, which enhance both catalytic activity and atom economy[5]. The presence of single active sites in SACs also facilitates the investigation of structure-activity relationships at the molecular and atomic levels, bridging the gap between homogeneous and heterogeneous catalysis. Consequently, within just a few years of their introduction, SACs have rapidly emerged as a research frontier in catalysis and successfully applied to various energy-related reactions[6-10].
The seminal article by Zhang et al. has now been cited over 5,500 times, underscoring the impact and growing attention that SACs have received within the catalysis community, as illustrated in Figure 1.
Figure 1. (A) Publications and (B) citations based on the seminal article by Zhang et al. on SACs, retrieved from the Web of Science website on October 30, 2024. SACs: Single-atom catalysts.
Exciting progress has been made in high-performance electrocatalytic SACs. However, breaking the linear scaling relationship between the adsorption energy of reaction intermediates remains challenging due to the single-atom active sites, which fundamentally limits further improvement in catalytic efficiency. Compared to SACs, single-cluster catalysts (SCCs) offer multiple active centers and more flexible adsorption configurations, allowing intermediates to adsorb at different active centers. This flexibility is expected to overcome the limitations imposed by the linear scaling relationship[11,12].
Moreover, the synergistic interaction between multiple metal atoms in SCCs can modulate the electronic structure, providing enhanced catalytic activity from different perspectives. Last but not least, the well-defined structure of SCCs facilitates the study of structure-performance relationships and catalytic mechanisms. In recent years, researchers have explored SCCs derived from SACs, mainly focusing on simple diatomic and triatomic catalysts (DACs and TACs)[13-15].
Nitrogen, the most abundant gas in Earth’s atmosphere (more than 78%), plays a crucial role in various industrial processes[16]. The industrial production of ammonia (NH3) via the Haber Bosch process, however, is energy-intensive and contributes significantly to environmental pollution[17]. Thus, the exploration of sustainable and green ammonia synthesis methods has been a research hotspot in recent years.
Significant efforts have been dedicated to developing high-performance electrocatalysts for the nitrogen reduction reaction (NRR)[18]. With regard to NRR, recently emerged SACs and SCCs have shown promise, demonstrating remarkable performance under mild conditions[19-21]. Theoretical calculations, which serve as a guiding tool for designing novel and efficient electrocatalysts, are both time- and cost-effective, greatly facilitating the screening of numerous potential catalysts without experimental effort.
This review summarizes recent theoretical and computational efforts in SACs and SCCs toward electrocatalytic ammonia synthesis. First, we introduce the typical reaction mechanisms. Next, we elaborate on the latest research progress of SACs towards NRR, classified by the elements of the active components, and present recent research on SCCs for NRR. Then, we discuss activity descriptors in detail. Finally, we highlight some existing challenges and outline future directions to achieve further breakthroughs in NRR and other multi-electron reactions, focusing on enhancing catalytic performance and selectivity.
BRIEF INTRODUCTION TO THE NRR REACTION MECHANISM
The reaction mechanisms for NRR vary depending on the type and structure of the catalysts. Conventional mechanisms include dissociative (DM) and associative mechanisms (AM). In the DM, the N≡N triple bond is completely broken at the active site before hydrogenation occurs, similar to the traditional Haber-Bosch process. AMs can be further categorized into consecutive, distal, alternating, enzymatic, and continuous pathways, depending on the mode of N2 adsorption and hydrogenation [Figure 2A].
Figure 2. (A) Schematic illustration of five possible mechanisms (consecutive, enzymatic, alternative, distal, and mixed) for NRR. Reprinted with permission from Ref.[22]. Copyright © 2021, American Chemical Society; (B) MvK mechanism for NRR. Reprinted with permission from Ref.[23]. Copyright © 2019 WILEY-VCH Verlag GmbH & Co. KGaA, Weinheim; (C) Enzyme-distal mechanism for NRR. Reprinted with permission from Ref.[24]. Copyright © 2021 Dalian Institute of Chemical Physics, the Chinese Academy of Sciences; (D) N2 dissociation mechanism under confined dual sites (pink and blue spheres denote metal and nitrogen atoms, respectively; IS, TS, and FS denote initial, transition, and final states, respectively). Reprinted with permission from Ref.[25]. Copyright © 2024 National Academy of Sciences; (E) Reaction mechanism for NRR on Rh surface. Reprinted with permission from Ref.[26]. Copyright © 2020 Wiley-VCH Verlag GmbH & Co. KGaA, Weinheim; (F) Schematic of surface-hydrogenation mechanism for NRR on noble-metal-based catalysts. Reprinted with permission from Ref.[27]. Copyright © 2019 American Chemical Society. NRR: Nitrogen reduction reaction; MvK: Mars-van-Krevelen; IS: initial state; TS: transition state; FS: final state.
For the N2 end-on adsorption configuration, the distal mechanism involves hydrogenating the nitrogen atom furthest from the active center to form NH3, while the alternating mechanism involves hydrogenating both nitrogen atoms in succession. In the enzymatic and consecutive mechanisms, the hydrogenation of side-on adsorbed N2 is similar to the alternating and distal mechanisms, respectively, except that N2 is adsorbed in a side-on configuration. Additionally, the Mars-van-Krevelen (MvK) mechanism, proposed by Skúlason and Abghoui[28-30], involves the reduction of a surface N atom to NH3, followed by a reformation of the nitrogen-vacancy, particularly in TM nitrides (TMNs) [Figure 2B].
Qu et al. discovered a novel enzyme-distal mechanism in which the potential-determining step is the transition from *NH2 to NH3 [Figure 2C]. This process occurs because both the lone pairs of electrons and the unbonded sp3 electrons in N atoms in SAC@MXene systems can be coordinated with single metal atomic sites. During the final hydrogenation step, the SAC-N interaction is disrupted, and a new N–H σ bond is formed, resulting in an uphill change in energy. By weakening the SAC–N bond, the adsorption of
More recently, Wang et al. designed a localized dual-active site catalyst that successfully broke the linear relationship of traditional systems[25]. They proposed a new mechanism involving the co-activation of N2 at both ends. Following this mechanism, two weakly adsorbed N atoms are effectively activated and the N≡N triple bond is cleaved, with each N atom binding to separate weakly adsorbed metal centers, thus facilitating subsequent hydrogenation steps [Figure 2D].
Notably, Wang et al. introduced another novel mechanism, where two *N2H2 species desorbed from a Rh surface and subsequently decomposed into NH3 and N2 [Figure 2E][26]. In addition to the DMs or AMs described above, they proposed a surface-hydrogenation mechanism where N2 is activated and reduced to
SACS TOWARDS NRR
Mo-based SACs
In nature, nitrogenase enzymes convert N2 to NH3 under mild conditions (< 40 °C, atmospheric pressure)[31]. Unfortunately, biological nitrogenases are significantly influenced by environmental factors, leading to instability in nitrogen fixation and limiting their large-scale applications. The active center of nitrogenase systems comprises clusters of different metal atoms, such as Fe-Mo, V-Fe, and Fe-Fe nitrogenases. Naturally, bio-inspired catalyst structures containing Mo, Fe, or V atoms deposited on two-dimensional (2D) nanomaterials have been extensively explored for their catalytic performance under mild conditions.
Recent reports on Mo/Fe/V-based SACs towards NRR, calculated using first-principles methods, are summarized in Table 1. Among these systems, the Fe-Mo nitrogenase systems have received the most attention, leading to the design of various Mo-containing catalysts for NRR. Thus, in this subsection, we focus on Mo-based SACs, highlighting representative catalysts.
Summary of the Mo/Fe/V-based SACs towards NRR investigated by DFT calculations
Systems | Limiting potential/V | Reaction mechanism | Year of publication |
Mo-BN | -0.35 | Enzymatic | 2017[32] |
Mo@C2N | -0.17 | Distal | 2018[33] |
Mo-MoS2 | -0.53 | Distal/alternating | 2018[34] |
Mo@GDY | -0.33 | Distal | 2020[35] |
Mo-Pp | -0.58 | Distal/alternating | 2020[36] |
Mo@BM-β12 | -0.26 | Enzymatic | 2021[37] |
Mo@BM-α | -0.32 | Enzymatic | 2022[38] |
Mo1-N1C2 | -0.40 | Enzymatic | 2018[39] |
Mo1/N3-G | -0.50 | Distal-to-alternating hybrid | 2019[40] |
MoN3@555-777 graphene | -0.57 -0.57 | Distal Enzymatic | 2020[41] |
Mo/g-CN | -0.39 | Distal | 2020[42] |
Mo-MoSSe with S vacancy | -0.49 | Alternating | 2019[43] |
Mo-based MOFs | -0.34 | Distal/alternating | 2019[44] |
Mo@BCN | -0.59 | Enzymatic | 2019[45] |
Mo/G with Se doping | -0.41 | Enzymatic | 2019[46] |
Mo1N3-BP | -0.18 | Distal | 2019[47] |
Mo/CeO2-S | -0.52 | Distal/alternating | 2019[48] |
Mo-PTA | -0.42 | Distal | 2019[49] |
Mo1-PMA | -0.35 | Enzymatic | 2022[50] |
FeB6 (β) | -0.68 | Distal | 2019[51] |
V@BN | -0.41 | Enzymatic | 2020[52] |
V1@Ti2CO2 | -0.20 | Mixed | 2023[53] |
V@g-C3N4 | -0.55 | Distal | 2023[54] |
Fe-C2N | -0.7 | Distal/alternating | 2020[55] |
Ti@N4 V@N4 | -0.69 -0.87 | Distal Distal | 2018[56] |
V/β12-BM | -0.28 | Enzymatic | 2019[57] |
V@GDY | -0.67 | Distal/alternating | 2020[58] |
V/Ars | -0.26 | Enzymatic | 2020[59] |
Zhao et al. systematically investigated the potential of a series of single TM atoms (Sc ~ Zn, Nb, Mo, Rh, Ru, Pd, and Ag) anchored on the BN monolayers with a boron monovacancy (TM-BN) and on C2N monolayer (TM@C2N) as NRR catalysts by density functional theory (DFT) calculations. The results showed that Mo-BN [Figure 3A] and Mo@C2N are promising NRR catalysts with low UL of -0.35 and -0.17 V, respectively[32,33]. In addition, Zhao et al. systematically studied the activity of single atoms of Sc, Ti, V, Cr, Mn, Fe, Co, Ni, Cu, Mo, Rh, and Ru embedded on MoS2 nanosheets with S-vacancy defects (TM/MoS2) as NRR catalysts. It is indicated that Mo/MoS2 nanosheet exhibits the highest NRR activity due to the high stability of *N2H intermediates[34]. These findings suggest that the choice of substrate material significantly influences catalytic activity due to differences in the coordination environment of the Mo center. Specifically, the intrinsic nitrogen reduction catalytic activity and selectivity of SACs are strongly influenced by the surrounding environment of the active metal atoms and the geometry of the support, just as they are in the oxygen reduction reaction (ORR)[60].
Figure 3. Representative optimized structures of Mo-embedded SACs. (A) Mo-BN, reprinted with permission from Ref.[32]. Copyright © 2017 American Chemical Society; (B) Mo@GDY, reprinted with permission from Ref.[35]. Copyright © 2019 Elsevier B.V.; (C) Mo-Pp, reprinted with permission from Ref.[36]. Copyright © 2020 American Chemical Society; (D) Mo@BM-β12, reprinted with permission from Ref.[37]. Copyright © 2021 American Chemical Society; (E) Mo@BM-α, reprinted with permission from Ref.[38]. Copyright © 2022 Elsevier B.V. SACs: Single-atom catalysts; Mo@GDY: graphdiyne monolayer.
Mo-based SACs supported by graphene-like 2D materials are expected to combine the advantages of Mo atoms (the active center of natural nitrogenase) and 2D substrates (acting as electron bridges and reservoirs), showing great potential for NRR applications. Zhai et al. reported that Mo embedded in a graphdiyne (GDY) monolayer (Mo@GDY) [Figure 3B] stood out among a series of TM-embedded GDY monolayer (TM@GDY, TM = Sc, Cr, Mn, Fe, Co, Ni, Cu, Zn, Mo, Ru, Rh, Pd, and Ag), exhibiting high stability, excellent conductivity, and superior NRR activity through the distal mechanism. This behavior was attributed to the strong interactions between hydrogen and the neighboring nitrogen atoms (H-N2) during the first hydrogenation step[35].
Based on the experimental work by Osuka et al. on the tetrameric porphyrin sheet and linear conjugated Zn-Pp tapes[61,62], Liu et al. investigated extended 2D TM porphyrin sheets (TM-Pp, TM = Sc, Ti, V, Cr, Mn, Fe, Co, Ni, Cu, Zn, Nb, Mo, Ru) for NRR[36]. Their study indicated that Mo-Pp [Figure 3C] is a promising NRR electrocatalyst under ambient conditions due to its high thermodynamic stability and low UL.
The combination of edge fluoridation with Mo dopants endowed Mo single atoms anchored on zigzag graphene nanoribbons with H/F terminations (Mo-g/H and Mo-g/F) with excellent NRR properties. Electronic analysis showed that edge termination modulates the binding strength of Mo anchoring, alternating its affinity for NRR intermediates and boosting NRR selectivity[63].
Xu et al. systematically studied the NRR activity of metal-atom-embedded M@BM-β12 (including 3d/4d TMs and main group metals) SACs, and their results showed that Mo@BM-β12 [Figure 3D] and Mn@BM-β12 demonstrate promising properties with UL of -0.26 and -0.32 V, respectively, and could effectively suppress the competitive hydrogen evolution reaction (HER)[37].
Similarly, Li et al. studied the donor-acceptor interaction between TM atoms and N2, showing that during N2 activation, the antibonding orbital of N2 accumulates significant negative charge transferred from the TM atom, thus facilitating the activation of N2. Among the 20 TMs (3d/4d TMs) on an α-borobenzene monolayer (BM-α), V@BM-α and Mo@BM-α [Figure 3E] showed the best activity, with UL of -0.22 and
Single TM atoms coordinated by N atoms (TM-Nx) are also attractive for NRR. Wang et al. reported that the experimentally realized single Mo atoms supported on N-doped carbon (Mo1-N1C2, a Mo atom coordinated by one N atom and two C atoms) exhibited excellent NRR activity via the enzymatic mechanism[39]. Yang et al. reported that a single Mo atom supported on nitrogen-doped graphene (Mo1/Gr1-N3G) has high activity and selectivity with low UL (-0.50/-0.75 V) and high selectivity (40%/100%) via a new distal-to-alternating hybrid mechanism involving two spectator N2 molecules[40].
Liu et al. demonstrated that defective graphene substrates could act as electron reservoirs during the NRR process[41]. In a series of single TM atom anchored divacancy 555-777 graphene systems (containing three pentagons and three heptagons constructed by removing two neighboring C atoms), MoN3@555-777 graphene sheets showed good catalytic activity with a low UL of -0.57 V.
Niu et al. explored a series of TM atoms (from Sc to Au) supported on graphitic carbon nitride (g-CN) SACs for NRR[42]. Due to high activity (with low UL of -0.42, -0.39, -0.35, -0.29, and -0.39 V, respectively), high selectivity (100%, 100%, 100%, 94%, and 69%, respectively), and good kinetic stability, Nb, Mo, Ta, W, and Re/g-CN were identified as efficient NRR electrocatalysts.
In summary, leveraging the advantages of Mo atom active centers inspired by natural nitrogenase and 2D substrate materials holds great promise for achieving the catalytic conversion of N2 to NH3 under mild conditions. This can be realized by precisely tuning the coordination environment of Mo atoms. Furthermore, a detailed and accurate characterization of the electronic structure of SACs is essential for gaining a deeper understanding of their NRR activity[64].
Non-Mo-based SACs
Fe-based SACs
Apart from Mo, Fe has garnered significant attention as an essential metal in nitrogenase enzymes responsible for biological nitrogen fixation[65]. For example, Fe-based catalysts have been widely used in the industrial Haber-Bosch process for NH3 synthesis[66]. Li et al. demonstrated that a highly spin-polarized FeN3 active center on graphene with a local magnetic moment, which enhances N2 adsorption and activation, is a promising NRR catalyst at room temperature[67]. Li et al. revealed that Fe(V)-PTC exhibited excellent NRR activity among a series of TM-PTC (TM = Fe, Sc, Ti, V, Cr, Mn, Co, Ni, Cu) SACs, attributed to the donation/back-donation mechanism[68]. Sahoo et al. investigated the NRR mechanism for single Au and Fe atoms supported on C2N monolayers. Their findings indicated that Fe-C2N was a better catalyst than Au-C2N, with a UL of -0.7 V, owing to its stronger N2 adsorption energy[55].
V-based SACs
Vanadium nitrogenase is another biological nitrogenase system in nature[69]. Therefore, researchers have extensively studied the NRR performance of V-based SACs.
Among others, Choi et al. examined the NRR performance of single atoms anchored on defective graphene derivatives by DFT computations[56]. Ti@N4 and V@N4 were identified as efficient catalysts with low UL and high selectivity due to strong back-bonding interactions between the hybridized d-orbital metal atoms and the π* orbital of *N2. Zhu et al. found that V/β12-BM demonstrated good energy efficiency for NRR due to the acceptor-donor interaction between the V atom and N2[57].
Zhen et al. showed that TM@GDY nanomaterials (TM = Ti, V, Fe, Co, Zn, Rh, Hf) are promising SACs for NRR, surpassing Ru(0001) stepped surfaces in performance. In particular, V@GDY exhibited the lowest UL of -0.67 V[58]. Most recently, Xu et al. screened V-, Fe-, Co-, and Ru-doped arsenene nanosheets, identifying them as efficient, low-cost NRR catalysts. In particular, during the NRR process, the arsenene nanosheet acts as a medium for accepting and donating electrons, while VAs3 serves as a charging transmitter between NxHy species and the nanosheet, resulting in high catalytic efficiency for V-doped arsenide (V/Ars) with a low UL of -0.26 V[59].
The study of bio-inspired catalysts with Mo-, Fe-, and V-like nitrogenase active centers for ammonia synthesis at room temperature and atmospheric pressure holds great potential but presents significant challenges. Much work remains to be done, particularly concerning the underlying mechanisms.
Nobel metal-based SACs
Noble metal-based catalysts, such as Ru and Pt, have been extensively studied for NRR due to their strong electron-donating properties, which arise from their unfilled d orbitals.
Liu et al. proposed that single Ru atoms anchored in hexagonal pores of boron monolayers (Ru/B α-sheet and Ru/B β12-sheet) could serve as promising monoatomic NRR catalysts, with reaction energy barriers less than half that of flat Ru(0001) catalysts[70]. Yin et al. found that Pt/g-C3N4 exhibited outstanding NRR catalytic activity at room temperature, with a low UL of -0.24 V, attributed to the synergistic interaction between Pt atoms and the g-C3N4 substrate, which optimized the energetics of *N2H and *NH2 intermediates[71].
SACs with other TMs
While noble metal catalysts have shown promise for NRR, their high cost limits their large-scale application. TMs, such as W, Co, Nb, and Ni, are also being considered as active centers for NRR.
Chen et al. evaluated the catalytic performance of a series of single metal atoms loaded on g-C3N4[72]. The NRR catalytic activity of five TMs (Ti, Co, Mo, W, and Pt) supported on g-C3N4 monolayers exceeded that of Ru(0001) stepped surfaces. W@ g-C3N4, in particular, showed the highest catalytic activity with a UL of
Wang et al. studied the NRR behavior of SACs formed by a series of single TM atoms (from Sc to Zn) anchored on g-C9N10. They found that Mn/g-C9N10 exhibited excellent NRR activity with a low UL of
The Ni@Ti2NO2 MXene structure, which satisfies orbital symmetry matching, could achieve an acceptor-donor interaction, allowing N2 to be transformed via a novel “enzyme-distal” mechanism due to the synergistic interaction between Ti and Ni atoms[24].
Additionally, Nb and W embedded in defective boron phosphide (BP) monolayers with boron monovacancies (Nb/BP and W/BP) were documented as promising NRR electrocatalysts with low UL (-0.25 and -0.19 V), attributed to the favorable matching between the d-orbitals of Nb or W atoms and the p-orbitals of the N2 molecule above the Fermi level (EF)[75].
Gao et al. reported a theory-guided design of Nb catalysts supported on anatase TiO2(110) for NRR. Theoretical calculations showed that dispersing Nb atoms on anatase TiO2(110) significantly increased electron density at the EF, enhancing conductivity and facilitating the proton-coupled electron transfer process, thus leading to excellent NRR activity and selectivity for Nb-TiO2(110)[76].
Importance of coordination environment
Non-noble metal catalysts are advantageous due to their low cost and abundant availability; however, they often have weaker electron-donating abilities compared to noble metals. To enhance electron donation, heteroatoms are often introduced to regulate the density of d-orbital states[77]. At present, it remains a significant challenge to select matched heteroatoms to regulate the structure of non-noble metal catalysts accurately.
Zhao et al. recently proposed that introducing boron dopants in Fe-N4/G could effectively regulate the interaction between active centers and *N2H species. Specifically, Fe coordinated by two boron and two nitrogen atoms exhibited outstanding NRR activity[78]. Wang et al. studied TM atoms anchored on N/O-codoped graphene (TM-OxNy@Gra, x + y = 4) for NRR, demonstrating that catalytic performance can be modulated through coordination engineering[79]. Tang et al. identified two excellent NRR catalysts
These results indicate that, in addition to TM active centers, the coordination environment plays a crucial role in catalytic activity. Synergistic effects can modify electronic structures, thereby creating a favorable environment for nitrogen fixation, promoting chemisorption of N2 molecules, and activating the inert N≡N triple bond. Furthermore, the prediction of the catalytic activity of each SAC should be complemented by parallel investigations into its stability[81].
To facilitate comparison of catalytic performance, the reaction mechanisms and UL of various catalysts are summarized in Table 2.
Summary of the metal atoms other than Mo/Fe/V-based SACs towards NRR
System | Limiting potential/V | Reaction mechanism | Year of publication |
Ni@Ti2NO2 | -0.13 | Enzymatic-distal | 2021[24] |
Mn@BM-β12 Ta@BM-β12 Cr@BM-β12 | -0.32 -0.38 -0.48 | Distal Enzymatic Enzymatic | 2021[37] |
V@BM-α | -0.22 | Enzymatic | 2022[38] |
Gr1/N3-G | -0.75 | Distal-to-alternating hybrid | 2019[40] |
Nb/g-CN Ta/g-CN W/g-CN Re/g-CN | -0.42 -0.35 -0.29 -0.39 | Distal Distal Distal Distal | 2020[42] |
Ru/CeO2-S | -0.35 | Distal | 2019[48] |
Ta@BN | -0.59 | Enzymatic | 2020[52] |
Ti@g-C3N4 | -0.84 | Alternating | 2023[54] |
Ru/B α-sheet Ru/B β12-sheet | -0.58 -0.60 | Distal Distal | 2019[70] |
Pt/g-C3N4 | -0.24 | Alternating | 2019[71] |
W@g-C3N4 | -0.35 | Enzymatic | 2018[72] |
Mn/g-C9N10 | -0.295 | Distal | 2023[73] |
Co/N3-Gr | -0.53 | Enzymatic | 2019[74] |
Nb/BP W/BP | -0.25 -0.19 | Enzymatic Enzymatic | 2021[75] |
Fe–B2N2/G | -0.65 | Distal | 2021[78] |
V-N4@Gra | -0.44 | Consecutive | 2022[79] |
Ta-N4@Gra | -0.49 | Distal | |
V-O1N3@Gra | -0.40 | Consecutive | |
V-O2N2α@Gra | -0.42 | Consecutive | |
V-O2N2β@Gra | -0.44 | Consecutive | |
V-O2N2γ@Gra | -0.38 | Consecutive | |
V-O3N1@Gra | -0.39 | Consecutive | |
Mo-O3N1@Gra | -0.48 | Distal | |
V-O4@Gra | -0.43 | Consecutive | |
Ru-O4@Gra | -0.43 | Distal | |
V-S3@Gr | -0.00 | Mixed | 2023[80] |
V-S2C@Gr | -0.17 | Mixed | |
Ti@CN | -0.38 | Enzymatic | 2021[82] |
Nb@P3-Ars | -0.52 | Distal | 2021[83] |
Ta@g-C7N3 W@g-C7N3 | -0.27 -0.06 | Distal Alternating | 2022[84] |
Mn@GY | -0.36 | Distal | 2021[85] |
U@N1C2 | -0.46 | Enzymatic | 2022[86] |
Consecutive |
SCCS TOWARDS NRR
In recent years, SCCs for NRR have garnered significant research interest. While catalytic processes are often simplified into reactant adsorption and product desorption, a deeper understanding of the catalytic enhancement of SCCs can emerge from analyzing the synergistic interactions within atomic clusters and between clusters and substrates. Moreover, the flexible clusters can adaptively modify their structure during the reaction process, thereby significantly improving their catalytic activity[87]. In this subsection, we will focus on three kinds of SCCs: DACs, TACs, and transition metal-free catalysts (TMFCs).
DACs
Compared to SACs, DACs offer multiple active sites, enabling diverse adsorption modes [Figure 4A][88]. The reactivity and activation mechanisms of SACs and DACs may also be different due to distinct d-orbital occupations - σ-donation/π-backdonation for SACs and π-donation/π-backdonation for DACs
Figure 4. (A) Schematic diagram of adsorption configurations of diatomic molecules at single-atom site and dual-atom site. Reprinted with permission from Ref.[88]. Copyright © 2022 Wiley-VCH GmbH; (B) N2 molecule activation mechanism for the SACs and DACs. Reprinted with permission from Ref.[89]. Copyright © 2020 The Authors. EcoMat published by John Wiley & Sons Australia; (C) Adsorption configuration and corresponding charge density difference of N2 and free energy diagrams of NRR on Mn@C2N and
DACs can modify the adsorption and activation modes of reactants, intermediates, and products through the synergistic interactions between multiple atomic sites, thereby enhancing the reactivity. For example, varying N2 adsorption configurations on Mn@C2N and Mn2@C2N lead to greater charge transfer from the Mn’s d orbitals to N2’s antibonding orbitals in the Mn2@C2N system, resulting in higher NRR activity
Homonuclear metal dimers anchored on 2D substrates have demonstrated superior NRR performance compared to their single-atom counterparts, such as Cr2-N6G and Mn2-N6G[22], Cr2@C2N and V2@C2N[89], Mo2@C2N[91], and binuclear Mo on N-doped nanoporous graphene (Mo2-N-C)[92]. Recently, Zhang et al. revealed that the presence of a second metal atom not only modulates the electronic structure but also actively participates in N2 activation and early hydrogenation through a unique N2 adsorption configuration. This configuration disrupts the undesirable linear scaling relationships of key intermediate adsorption energies on the catalyst surface. The effective cooperation of dimers resulted in excellent NRR performance, as demonstrated by V2@g-C3N4 and Ni2@g-C3N4 [Figure 5A][93,94].
Figure 5. (A) Free energy profiles and corresponding intermediate structures on V2@g-C3N4 andNi2@g-C3N4, Color scheme: H, white; C, gray; N, blue; V, red; Ni, pink. Reprinted with permission from Ref.[93]. Copyright © 2022 Wiley-VCH GmbH; (B) N≡N bond lengths with and without H* generated on the X site and the relationship between
Furthermore, heteronuclear metal dimers in the DACs may exhibit even better performance than homonuclear dimers due to the d-orbital electronic structure modulation enabled by heteronuclear atom synergy[95]. Recent examples include Fe/Mn-N-C[96], Mo-Ru, Mo-Co, Mo-W, Mo-Fe, and Fe-Ru embedded in a nitrogen-doped graphene framework[97], FeMo@NG[98], CoMo@N6[99], FeCo@GDY and NiCo@GD[100]. Yang et al. recently proposed a novel N2 activation strategy, showing that the reactivity of X/Fe-N-C (X = Pd, Ir, Pt) dual-atom catalysts for N2 reduction can be adjusted by local hydrogen radical (H*) on the X site
TACs
Compared to the synthesis of DACs, preparing TACs is more challenging because it is difficult to control the number of atoms during synthesis precisely. Additionally, the high surface energy of low-coordinate atoms in TACs necessitates the use of an appropriate substrate to improve stability. The adsorption configuration of N2 on TACs is also more complex than that on DACs [Figure 6A], and research into TACs is still in its infancy[101]. In the following sections, we will explore some of their applications in NRR.
Figure 6. (A) Adsorption and dissociative adsorption structures of N2 on three-metal clusters. Reprinted with permission from Ref.[101]. Copyright © 2022 American Chemical Society; (B) Homogeneous and heterogeneous Fe3 cluster with N2 adsorption. Reprinted with permission from Ref.[102]. Copyright © 2018, The Author(s).
Liu et al. proposed a Fe3 cluster catalyst anchored on the surface of θ-Al2O3 (010) [Figure 6B] based on first-principles calculations and micro-kinetic analysis. The electron interaction between the Fe clusters and the substrate was very weak, resulting in a very low oxidation state for Fe atoms within the metal-metal bonded Fe3 clusters. Notably, these low oxidation state Fe3 clusters can serve as electron reservoirs, dynamically adjusting charge in the reaction process. In addition, the significant spin polarization of Fe atoms was identified as a critical factor in facilitating N2 activation[102].
Chen et al. investigated the NRR activity of SACs, DACs, and TACs supported on heterogeneous graphylene and graphene, denoted as Mx-GDY/Gra (where M = Mn, Fe, Co, and Ni; x = 1, 2, 3). Their findings showed that TACs exhibited better stability and catalytic performance than SACs and DACs, with Fe3-GDY/Gra with a theoretical mass loading of 35.8 wt% achieving a particularly low UL of -0.26 V. This enhanced activity was attributed to the M3 active site, which not only provided additional electrons for N2 activation but also displayed weaker adsorption of the product, facilitating easier release[103].
The reaction mechanisms and UL of DACs and TACs are summarized in Table 3; however, TACs are not always superior to DACs. Luo et al. demonstrated that Fe2/MoS2 exhibits high catalytic activity for NRR. Their findings suggest that, compared to Fe/MoS2, the presence of adjacent Fe atoms in Fe2/MoS2 enables a side-on adsorption configuration for N2, which is more favorable for effective activation. However, the addition of a third Fe atom (Fe3/MoS2) reduces electron sharing between Fe atoms, thereby inhibiting N2 adsorption[110].
Summary of the DACs and TACs towards NRR
Systems | Limiting potential/V | Reaction mechanism | Year of publication |
Mo2@C2N | -0.35 | Enzymatic | 2018[91] |
V2@g-C3N4 Ni2@g-C3N4 | -0.38 -0.39 | Mixed Distal | 2022[93] |
TiV@BP TiCo@BP VCr@BP VCo@BP VNi@BP | -0.38 -0.31 -0.41 -0.31 -0.38 | Mixed Distal Distal Distal | 2023[95] |
Fe/Mn-N-C | -0.37 | Distal | 2020[96] |
Mo-RuN6-NG Mo-CoN6-NG Mo-WN6-NG Mo-FeN6-NG Fe-RuN6-NG | -0.17 -0.27 -0.28 -0.36 -0.39 | Enzymatic Enzymatic Distal Enzymatic Enzymatic | 2020[97] |
CoMo@N6 | -0.52 | Distal | 2020[99] |
FeCo@GDY NiCo@GDY | -0.44 -0.36 | Distal Distal | 2021[100] |
Fe3-GDY/Gra | -0.26 | Distal | 2020[103] |
Mo2@B2-C2N | -0.25 | Enzymatic | 2024[104] |
Fe3@C2N | -0.57 | Enzymatic | 2020[105] |
Fe3@N4C6 | -0.45 | Enzymatic | 2023[106] |
VNiCu@NV-W2N3 Fe2Co@NV-W2N3 Fe3@NV-W2N3 | -0.05 -0.49 -0.10 | Enzymatic Enzymatic Enzymatic | 2023[107] |
Pt3@C3N3 Ru3@C3N3 | -0.24 -0.35 | Enzymatic | 2023[108] |
Ru3@g-C3N4 Rh3@g-C3N4 | -0.19 -0.02 | Enzymatic Enzymatic | 2024[109] |
In summary, the factors influencing NRR activity and selectivity are complex, and the catalytic performance of multi-atom clusters in NRR depends on the specific atomic configuration. Further research is needed to understand the underlying characteristics.
TMFCs
TMFCs, such as boron-based catalysts, have shown promise for the NRR. Due to the sp3 or sp2 electron configuration, boron atoms can provide empty orbitals that facilitate N2 activation. For example, Anis et al. investigated single, double, and triple boron atoms supported on a GDY monolayer (B@GDY, B2@GDY, and B3@GDY) for NRR, concluding that B3@GDY demonstrates outstanding catalytic performance and effectively suppresses HER [Figure 7A][111]. Similarly, Wang et al. proposed that a g-C3N4 monolayer embedded with double B atoms (B2@C3N4) enhances N2 activation [Figure 7B]. Their findings suggest strong hybridization between N2-2p orbitals and B-sp3 orbitals, which accounts for the high catalytic efficiency of B2@C3N4[112]. In a related study, Rasool et al. explored NRR on single- and double-boron-doped configurations across five different substrates, demonstrating that g-C3N4 is particularly effective[113].
Figure 7. (A) Free energy profiles and the optimized geometries of reaction intermediates for the NRR on B3@GDY. Reprinted with permission from Ref.[111]. Copyright © 2023 Wiley-VCH GmbH; (B) Free energy profiles for the NRR on B2@C3N4. Reprinted with permission from Ref.[112]. Copyright © 2022 by the authors. NRR: Nitrogen reduction reaction; GDY: graphdiyne.
These studies highlight boron-based TMFCs as efficient and selective NRR catalysts, offering a viable alternative to TMs by utilizing unique electronic interactions that enhance N2 activation while suppressing HER. This approach broadens the materials landscape for sustainable, TM-free catalysis. More efforts are encouraged to explore such catalysts, especially with elements beyond boron.
HIGH-THROUGHPUT CALCULATIONS AND EMERGING MACHINE LEARNING TOWARDS NRR
The search for materials with specific properties is challenging, as traditional trial-and-error methods are often inefficient. However, combining high-throughput computing with machine learning (ML) offers a powerful approach for materials prediction and design, addressing long development cycles and high costs, and accelerating the discovery of novel catalysts[114].
High-throughput DFT calculations
TM elements, coordination environments, and substrates significantly influence NRR efficiency, and the numerous possible combinations can greatly benefit from high-throughput DFT calculations to streamline the search for optimal configurations[115].
By exploring extensive combinations, researchers can more effectively identify catalysts with promising properties for NRR applications. Lv et al. developed a “five-step” screening strategy [Figure 8A] using high-throughput DFT calculations to evaluate TM2/CN catalysts for NRR. This approach identified Fe2/g-CN as a highly active catalyst with low energy consumption and high selectivity, concluding that moderate electron donation from TM to N2 is crucial for balancing N2 activation and *NH3 formation steps[116]. Similarly, Sun et al. used high-throughput computations to screen TM1TM2@C9N4 [TM1, TM2 = 3(4) d TM atoms] and identified five effective catalysts (TM1, TM2 = NiRu, FeNi, TiFe, TiNi, NiZr) with strong activity[117]. Sun et al. further applied a “four-step” screening strategy [Figure 8B], predicting that six catalysts, including W@VB-V1-BC3, Re@VB-V1-BC3, Mo@VB-V2-BC3, Ti@VB-V3-BC3, Mo@VB-V3-BC3, and Ta@VC-V1-BC3, exhibited superior NRR activity and selectivity out of 33 candidates[118]. Pei et al. systematically assessed the stability of 3d-5d TM trimers embedded in C3N3 nanosheets (TM3@C3N3) and found that configurations with Re, Ru, or Pt trimers demonstrated excellent catalytic activity for NRR[108].
Figure 8. (A) “Five-step” screening strategy. Reprinted with permission from Ref.[116]. Copyright © 2021 American Chemical Society; (B) “Four-step” screening strategy. Reprinted with permission from Ref.[118]. Copyright © 2023 The Authors. Energy & Environmental Materials published by John Wiley & Sons Australia, Ltd on behalf of Zhengzhou University.
ML
While high-throughput computing provides a structured approach for catalyst design, the massive data generated can obscure key performance factors, making it challenging to pinpoint the critical influences on catalytic performance. Advanced data analysis tools are crucial to fully leverage high-throughput screening for next-generation NRR catalysts. Emerging ML techniques are providing us with such a solution, enabling rapid and efficient data extraction, prediction, and analysis. By accelerating the identification of high-performance materials from thousands of candidates, ML not only enhances our understanding of structure-activity relationships but also sheds light on the fundamental mechanisms of catalysis
Figure 9. (A) The workflow of ML to explore the origins of catalysis. Reprinted with permission from Ref.[118]. Copyright © 2023 The Authors. Energy & Environmental Materials published by John Wiley & Sons Australia, Ltd on behalf of Zhengzhou University; (B) ML screening and descriptor building framework of the work by Zhang et al.; (C) Workflow of the ML screening process as well as the number of selected candidates after each screening step of the work by Zhang et al. Reprinted with permission from Ref.[119]. Copyright © 2021 Zhengzhou University. ML: Machine learning.
In recent years, ML has shown promise in catalyst design, with applications emerging in NRR research. Wang et al. used ML techniques to explore the nitrogen reduction reactivity of novel, graphene-supported SACs, based on their prior high-throughput calculations [Figure 9B]. Their study evaluated 29 TMs and 57 ligand structures, generating 1,626 unexplored catalyst configurations. Using a trained ML model, they predicted four target properties (Ef,
Zhang et al. also applied ML models to evaluate the catalytic activity of SACs by directly predicting reaction Gibbs free energy. Their findings underscored the high predictive accuracy of the gradient boosting regression (GBR) model for both ∆G (*N2 → *NNH) and ∆G (*NH2 → *NH3). Feature importance analysis revealed that the accuracy of the GBR model was due to its effective identification of key characteristics related to the active center and coordination environment, with the covalent radius emerging as a particularly influential descriptor[120].
Despite its potential, ML-based catalyst selection is currently constrained by limited experimental data. Nevertheless, integrating high-throughput screening with ML strategies offers a promising pathway for catalyst discovery, opening new horizons for efficient and targeted materials design.
DESCRIPTOR-BASED SCREENING AND DESIGN OF NRR CATALYSTS
Given the complexity of multiple intermediates in the NRR process, developing simplified parameters for evaluating catalyst activity is beneficial. Compared with heavy DFT computations, descriptors can quickly predict catalyst performance, providing experimental researchers with a practical tool for screening high-performance materials. By identifying trends in electrocatalytic properties and using reactivity descriptors to forecast promising catalysts, we can realize the rational design of catalysts, especially for processes as complicated as NRR.
Adsorption energy descriptors
The adsorption energy reflects the strength of the interaction between the reactant and the catalyst and is a key indicator for selecting high-efficiency catalysts. When the adsorption energy falls within the optimal range, it reduces the reaction energy barrier and, consequently, the UL. Catalysts with moderate adsorption energy generally exhibit lower UL, making adsorption energy a simple and effective descriptor.
Chen et al. demonstrated that the NRR activity of TM/MoS2 catalysts is affected by the d-orbital electron density states at the EF, with N2 adsorption inversely proportional to the UL value[121]. Similarly, Ma et al. investigated the activity of TM (TM = Mn, Fe, Co, Ni)-based SACs/DACs/TACs on GDY, finding an approximate linear relationship between the adsorption energy of *N and UL[122].
Considering that the formation of *N2H is the potential determining step, Ren et al. examined the scaling relation between the adsorption energy of *N2H and UL, concluding that Nb atoms supported on g-C3N4 are particularly promising NRR catalysts due to their low UL and strong N2H* adsorption[123]. Luo et al. further observed that *N2H and *NH2 adsorption energies are proportional to ∆E(*N) on TM-modified Co4 clusters supported on GDY, revealing the scaling relationship among the adsorbed NxHy species. They concluded that tightly bound N atoms hinder NH3 formation, while loosely bound N atoms impede protonation of *N2 to *N2H. The results showed that the NRR activity of TM/g-CN catalyst can be well estimated by the adsorption energy of *N[124].
Collectively, these findings suggest that adsorption energy is a useful descriptor for estimating NRR activity, despite its limitation as a theoretical measure that lacks experimental control.
Electronic descriptors
The degree of electronic coupling between adsorbed intermediates and the catalyst also plays a central role in catalytic activity[116]. The crystal orbital Hamilton population (COHP) method, grounded in DFT calculations, provides a powerful tool for analyzing the electronic structure of chemical bonds within a crystal. Specifically, it characterizes the bonding and antibonding interactions between atoms. Its integrated counterpart, the integrated COHP (ICOHP), aggregates COHP values over a defined energy range, capturing both bonding and antibonding contributions to offer a holistic measure of bond strength. In NRR, COHP and ICOHP are commonly used to assess bonding and antibonding populations and the interactions between the catalyst and NRR intermediates. These tools provide critical insights into the formation and cleavage of chemical bonds during the reaction, deepening our understanding of the origins of NRR activity.
As shown in Figure 10A, interactions between various TM centers and NRR intermediates can be categorized into bonding states below the EF and antibonding states above EF. These unique properties make COHP a powerful and effective electronic descriptor for evaluating catalytic performance.
Figure 10. (A) Schematic diagram illustrating how TM centers interact with NRR intermediates (*N as an example) on TM/g-CN; (B) Linear relationship between ICOHP and the adsorption energy of *N on TM/g-CN. Reprinted with permission from Ref.[42]. Copyright © 2020, American Chemical Society; (C) and (D) Linear relationships between ICOHP and ΔGN2-NNH on TM@N6-G and TM@g-C2N. Reprinted with permission from Ref.[125]. Copyright © 2022, Elsevier B. V. TM: Transition metal; NRR: nitrogen reduction reaction; ICOHP: integrated crystal orbital Hamilton population.
Through an ICOHP analysis, Niu et al. examined the bonding and antibonding states of NxHy intermediates adsorbed on TM/g-CN, finding a strong linear correlation (R2 = 0.84) between ICOHP and *N adsorption energy [Figure 10B][42]. Similarly, Liu et al. applied ICOHP analysis to assess the NRR performance of TM@N6-G and TM@g-C2N, revealing a good linear relationship between ICOHP and ΔGN2-NNH (R2 = 0.88 and 0.99, respectively) [Figure 10C and D][125]. These results indicate that ICOHP is a promising descriptor to describe the NRR activity of SACs and SCCs.
In addition to ICOHP, other electronic properties, particularly spin magnetic moment, have been explored as descriptors for NRR. Wang et al. investigated the catalytic activity of SACs on 2D VSe2, finding that the UL for NRR is linked to the total magnetic moment of TM-VSe2[126]. Zhang et al. further studied dual single-atom sites, discovering that the metal atoms located in adjacent vacancies can regulate the spin magnetic moment of the active Fe atoms despite a large distance, thereby enhancing N2 activation and reduction[127].
Multiple-level descriptors
Beyond adsorption energy and electronic properties, multiple-level descriptors have also been explored to capture more nuanced aspects of catalytic performance. Among others, Niu et al. proposed a descriptor,
These multiple-level descriptors, encompassing both electronic and atomic-level properties, offer promising avenues for application across various catalytic systems. However, a universally applicable descriptor for efficiently estimating NRR activity remains to be developed, indicating an ongoing need for further research.
SUMMARY AND PERSPECTIVE
Theoretical calculations play a crucial role not only in designing new catalysts but also in enhancing our understanding of reaction mechanisms. This insight provides meaningful guidance for creating efficient NRR catalysts capable of producing high-value-added chemicals. In this review, we have summarized recent theoretical advancements in SACs and SCCs for NRR.
Compared to traditional catalysts, SACs and SCCs feature well-defined active centers, making them more conducive to studying reaction mechanisms. Their structured active sites allow theoretical predictions to align closely with experimental findings, deepening our understanding of catalytic processes. SACs, with their unique structural characteristics, offer exceptional NRR catalytic performance, though their low production efficiency presents challenges for large-scale synthesis. SCCs, a recent extension of SACs, provide higher atomic loading and more flexible active sites, where multi-center synergy can optimize the interaction of the reactant and intermediate with the active sites, enhance mechanistic clarity, and ultimately improve catalytic performance. However, note that SCCs are not always superior to SACs for NRR, as exemplified recently by DACs, where the anticipated advantages over SACs are not consistently observed and are highly dependent on the specific properties of the metal[130].
In general, the coordination and electronic structure of the metal center, along with interactions between the support and the metal, are essential in determining the activity and stability of SACs and SCCs. Although SACs and SCCs have shown great promise in NRR applications, their performance is still insufficient to meet the demands of commercial-scale production.
With continuous in-depth study of the mechanism, we strongly believe that achievements in this field will continue to grow and inspire new advancements. Future breakthroughs in NRR catalyst development can focus on several areas, as outlined below.
First, advancing the synthesis of high-loading DACs and TACs is critical and faces significant challenges. Achieving precise atom or cluster dispersion without aggregation becomes increasingly difficult at higher loadings. Furthermore, identifying and optimizing suitable precursors and dispersion vectors requires meticulous effort and innovation. To address these issues, developing more efficient and robust synthesis strategies is imperative. Additionally, in situ characterization techniques are needed to precisely define active centers, enabling atomic-level investigations of structure-activity relationships and catalytic mechanisms. Such studies will provide invaluable insights into the interplay between catalyst structure and performance, guiding the rational design of high-loading SACs and SCCs.
Second, scaling the industrial application of SACs and SCCs presents formidable challenges, including high production costs driven by complex synthesis methods and the reliance on expensive precursors. Additionally, their stability and durability under rigorous industrial operating conditions require significant improvement. Future research will prioritize the development of cost-effective and scalable synthesis techniques alongside exploring novel support materials to bolster stability and longevity. Efforts will also optimize catalyst formulations tailored to specific industrial applications, ensuring both performance and practicality. As these technological advancements converge, SACs and SCCs are poised to transform industrial processes, unlocking new opportunities for efficient and sustainable energy conversion.
Third, it is essential to integrate theoretical calculations with experimental design to accelerate the research cycle. By combining theoretical and experimental efforts, researchers can promote the rational design of electrocatalysts with optimized activity, selectivity, and durability, generating significant economic and social benefits. To bridge the gap between theoretical simulations and experimental outcomes, advanced computational chemistry methods and standardized approaches are also necessary.
Fourth, it is vital to develop generic activity descriptors that reveal the relationship between catalytic performance and material properties. A deeper understanding of these intrinsic relationships will support the advancement of SACs and SCCs, representing a new frontier in materials science. This progress not only offers a pathway to more efficient catalysts for NRR but also has broader implications for other multi-electron reactions.
In conclusion, single-atom and small-cluster catalysts mark a promising advance in the quest for efficient nitrogen reduction catalysts, and theoretical studies, especially high-throughput computations, ML, and descriptor-based screening techniques, are providing us with powerful tools to explore catalytic mechanisms and screen and design high-performance catalysts. While challenges remain - such as large-scale synthesis, commercialization and bridging theory with experiment - the synergy between theory and experiment is transforming catalyst design, and brings us closer to a future where SACs and SCCs drive sustainable NRR catalysis and open doors for other multi-electron reactions, blending curiosity with real-world impact.
DECLARATIONS
Authors’ contributions
Conceived the idea and designed the project: Li, F., Chen, Z.
Performed data analysis and interpretation: Meng, H., Zhao, Y.
Supervised the project: Li, F.
Drafted the manuscript: Meng, H.
Revised and finalized the manuscript: Li, F., Chen, Z.
Availability of data and materials
Not applicable.
Financial support and sponsorship
This work was supported by the National Natural Science Foundation of China (12364038, 22103026), the young science and technology talents cultivation project of Inner Mongolia University (21200-5223708), the Industrial Technology Innovation Projects of Inner Mongolia Academy of Science and Technology of China (2023JSYD01002) and Science and Technology Plan Projects of Inner Mongolia Autonomous Region of China (2023KYPT0012).
Conflicts of interest
Chen, Z., an Associate Editor of Journal of Materials Informatics, and Li, F., the Guest Editor of the special issue, did not participate in any aspect of the editorial process, including reviewer selection, manuscript handling, or decision-making. The other authors declare that they have no conflicts of interest.
Ethical approval and consent to participate
Not applicable.
Consent for publication
Not applicable.
Copyright
© The Author(s) 2025.
REFERENCES
1. Abbet, S.; Sanchez, A.; Heiz, U.; et al. Acetylene cyclotrimerization on supported size-selected Pdn clusters (1 ≤ n ≤ 30): one atom is enough! J. Am. Chem. Soc. 2000, 122, 3453-7.
2. Li, Y.; Zhou, Z.; Yu, G.; Chen, W.; Chen, Z. CO catalytic oxidation on iron-embedded graphene: computational quest for low-cost nanocatalysts. J. Phys. Chem. C. 2010, 114, 6250-4.
3. Lee, D. H.; Lee, W. J.; Lee, W. J.; Kim, S. O.; Kim, Y. H. Theory, synthesis, and oxygen reduction catalysis of Fe-porphyrin-like carbon nanotube. Phys. Rev. Lett. 2011, 106, 175502.
4. Qiao, B.; Wang, A.; Yang, X.; et al. Single-atom catalysis of CO oxidation using Pt1/FeOx. Nat. Chem. 2011, 3, 634-41.
5. Yang, X. F.; Wang, A.; Qiao, B.; Li, J.; Liu, J.; Zhang, T. Single-atom catalysts: a new frontier in heterogeneous. Acc. Chem. Res. 2013, 46, 1740-8.
6. Akri, M.; Zhao, S.; Li, X.; et al. Atomically dispersed nickel as coke-resistant active sites for methane dry reforming. Nat. Commun. 2019, 10, 5181.
7. Han, B.; Guo, Y.; Huang, Y.; et al. Strong metal-support interactions between Pt single atoms and TiO2. Angew. Chem. Int. Ed. Engl. 2020, 59, 11824-9.
8. Jeong, H.; Lee, G.; Kim, B. S.; Bae, J.; Han, J. W.; Lee, H. Fully dispersed Rh ensemble catalyst to enhance low-temperature activity. J. Am. Chem. Soc. 2018, 140, 9558-65.
9. Wang, Y.; Arandiyan, H.; Scott, J.; Aguey-Zinsou, K.; Amal, R. Single atom and nanoclustered Pt catalysts for selective CO2 reduction. ACS. Appl. Energy. Mater. 2018, 1, 6781-9.
10. Bai, S.; Liu, F.; Huang, B.; et al. High-efficiency direct methane conversion to oxygenates on a cerium dioxide nanowires supported rhodium single-atom catalyst. Nat. Commun. 2020, 11, 954.
11. Sun, C. N.; Wang, Z. L.; Lang, X. Y.; Wen, Z.; Jiang, Q. Synergistic effect of active sites of double-atom catalysts for nitrogen reduction reaction. ChemSusChem 2021, 14, 4593-600.
12. Wang, P.; Chang, F.; Gao, W.; et al. Breaking scaling relations to achieve low-temperature ammonia synthesis through LiH-mediated nitrogen transfer and hydrogenation. Nat. Chem. 2017, 9, 64-70.
13. Guo, X.; Gu, J.; Lin, S.; Zhang, S.; Chen, Z.; Huang, S. Tackling the activity and selectivity challenges of electrocatalysts toward the nitrogen reduction reaction via atomically dispersed biatom catalysts. J. Am. Chem. Soc. 2020, 142, 5709-21.
14. Ouyang, Y.; Shi, L.; Bai, X.; Li, Q.; Wang, J. Breaking scaling relations for efficient CO2 electrochemical reduction through dual-atom catalysts. Chem. Sci. 2020, 11, 1807-13.
15. Chen, Z.; Chen, L. X.; Yang, C. C.; Jiang, Q. Atomic (single, double, and triple atoms) catalysis: frontiers, opportunities, and challenges. J. Mater. Chem. A. 2019, 7, 3492-515.
16. Galloway, J. N.; Townsend, A. R.; Erisman, J. W.; et al. Transformation of the nitrogen cycle: recent trends, questions, and potential solutions. Science 2008, 320, 889-92.
17. Foster, S.; Bakovic, S. I. P.; Duda, R. D.; et al. Catalysts for nitrogen reduction to ammonia. Nat. Catal. 2018, 1, 490-500.
18. Fu, X.; Zhang, J.; Kang, Y. Recent advances and challenges of electrochemical ammonia synthesis. Chem. Catal. 2022, 2, 2590-613.
19. Iqbal, M. S.; Yao, Z.; Ruan, Y.; et al. Single-atom catalysts for electrochemical N2 reduction to NH3. Rare. Met. 2023, 42, 1075-97.
20. Shang, H.; Liu, D. Atomic design of carbon-based dual-metal site catalysts for energy applications. Nano. Res. 2023, 16, 6477-506.
21. He, T.; Puente-Santiago, A. R.; Xia, S.; Ahsan, M. A.; Xu, G.; Luque, R. Experimental and theoretical advances on single atom and atomic cluster-decorated low-dimensional platforms towards superior electrocatalysts. Adv. Energy. Mater. 2022, 12, 2200493.
22. Chen, J.; Cao, H.; Chen, J.; et al. Heterogeneous two-atom single-cluster catalysts for the nitrogen electroreduction reaction. J. Phys. Chem. C. 2021, 125, 19821-30.
23. Yan, Z.; Ji, M.; Xia, J.; Zhu, H. Recent advanced materials for electrochemical and photoelectrochemical synthesis of ammonia from dinitrogen: one step closer to a sustainable energy future. Adv. Energy. Mater. 2020, 10, 1902020.
24. Qu, J.; Xiao, J.; Chen, H.; Liu, X.; Wang, T.; Zhang, Q. Orbital symmetry matching: achieving superior nitrogen reduction reaction over single-atom catalysts anchored on Mxene substrates. Chinese. J. Catal. 2021, 42, 288-96.
25. Wang, T.; Abild-Pedersen, F. Achieving industrial ammonia synthesis rates at near-ambient conditions through modified scaling relations on a confined dual site. Proc. Natl. Acad. Sci. U. S. A. 2021, 118, e2106527118.
26. Yao, Y.; Zhu, S.; Wang, H.; Li, H.; Shao, M. A spectroscopic study of electrochemical nitrogen and nitrate reduction on rhodium surfaces. Angew. Chem. Int. Ed. Engl. 2020, 59, 10479-83.
27. Ling, C.; Zhang, Y.; Li, Q.; Bai, X.; Shi, L.; Wang, J. New mechanism for N2 reduction: the essential role of surface hydrogenation. J. Am. Chem. Soc. 2019, 141, 18264-70.
28. Abghoui, Y.; Skúlason, E. Onset potentials for different reaction mechanisms of nitrogen activation to ammonia on transition metal nitride electro-catalysts. Catal. Today. 2017, 286, 69-77.
29. Abghoui, Y.; Skúlason, E. Computational predictions of catalytic activity of zincblende (110) surfaces of metal nitrides for electrochemical ammonia synthesis. J. Phys. Chem. C. 2017, 121, 6141-51.
30. Martín, A. J.; Shinagawa, T.; Pérez-Ramírez, J. Electrocatalytic reduction of nitrogen: from haber-bosch to ammonia artificial leaf. Chem 2019, 5, 263-83.
31. Shaw, S.; Lukoyanov, D.; Danyal, K.; Dean, D. R.; Hoffman, B. M.; Seefeldt, L. C. Nitrite and hydroxylamine as nitrogenase substrates: mechanistic implications for the pathway of N2 reduction. J. Am. Chem. Soc. 2014, 136, 12776-83.
32. Zhao, J.; Chen, Z. Single Mo atom supported on defective boron nitride monolayer as an efficient electrocatalyst for nitrogen fixation: a computational study. J. Am. Chem. Soc. 2017, 139, 12480-7.
33. Wang, Z.; Yu, Z.; Zhao, J. Computational screening of a single transition metal atom supported on the C2N monolayer for electrochemical ammonia synthesis. Phys. Chem. Chem. Phys. 2018, 20, 12835-44.
34. Zhao, J.; Zhao, J.; Cai, Q. Single transition metal atom embedded into a MoS2 nanosheet as a promising catalyst for electrochemical ammonia synthesis. Phys. Chem. Chem. Phys. 2018, 20, 9248-55.
35. Zhai, X.; Yan, H.; Ge, G.; et al. The single-Mo-atom-embedded-graphdiyne monolayer with ultra-low onset potential as high efficient electrocatalyst for N2 reduction reaction. Appl. Surf. Sci. 2020, 506, 144941.
36. Liu, S.; Liu, Y.; Gao, X.; et al. Two-dimensional transition metal porphyrin sheets as a promising single-atom-catalyst for dinitrogen electrochemical reduction to ammonia: a theoretical study. J. Phys. Chem. C. 2020, 124, 1492-9.
37. Xu, L.; Yang, L. M.; Ganz, E. Electrocatalytic reduction of N2 using metal-doped borophene. ACS. Appl. Mater. Interfaces. 2021, 13, 14091-101.
38. Li, C.; Liu, X.; Wu, D.; Xu, H.; Fan, G. Theoretical study of transition metal doped α-borophene nanosheet as promising electrocatalyst for electrochemical reduction of N2. Comput. Theor. Chem. 2022, 1213, 113732.
39. Ling, C.; Bai, X.; Ouyang, Y.; Du, A.; Wang, J. Single molybdenum atom anchored on N-doped carbon as a promising electrocatalyst for nitrogen reduction into ammonia at ambient conditions. J. Phys. Chem. C. 2018, 122, 16842-7.
40. Zhao, W.; Zhang, L.; Luo, Q.; et al. Single Mo1(Cr1) atom on nitrogen-doped graphene enables highly selective electroreduction of nitrogen into ammonia. ACS. Catal. 2019, 9, 3419-25.
41. Liu, P.; Fu, C.; Li, Y.; Wei, H. Theoretical screening of single atoms anchored on defective graphene for electrocatalytic N2 reduction reactions: a DFT study. Phys. Chem. Chem. Phys. 2020, 22, 9322-9.
42. Niu, H.; Wang, X.; Shao, C.; Zhang, Z.; Guo, Y. Computational screening single-atom catalysts supported on g-CN for N2 reduction: high activity and selectivity. ACS. Sustainable. Chem. Eng. 2020, 8, 13749-58.
43. Li, L.; Li, B.; Guo, Q.; Li, B. Theoretical screening of single-atom-embedded MoSSe nanosheets for electrocatalytic N2 fixation. J. Phys. Chem. C. 2019, 123, 14501-7.
44. Cui, Q.; Qin, G.; Wang, W.; K, R. G.; Du, A.; Sun, Q. Mo-based 2D MOF as a highly efficient electrocatalyst for reduction of N2 to NH3: a density functional theory study. J. Mater. Chem. A. 2019, 7, 14510-8.
45. Huang, Y.; Yang, T.; Yang, L.; et al. Graphene–boron nitride hybrid-supported single Mo atom electrocatalysts for efficient nitrogen reduction reaction. J. Mater. Chem. A. 2019, 7, 15173-80.
46. Zhou, H. Y.; Li, J. C.; Wen, Z.; Jiang, Q. Tuning the catalytic activity of a single Mo atom supported on graphene for nitrogen reduction via Se atom doping. Phys. Chem. Chem. Phys. 2019, 21, 14583-8.
47. Ou, P.; Zhou, X.; Meng, F.; Chen, C.; Chen, Y.; Song, J. Single molybdenum center supported on N-doped black phosphorus as an efficient electrocatalyst for nitrogen fixation. Nanoscale 2019, 11, 13600-11.
48. Qi, J.; Gao, L.; Wei, F.; Wan, Q.; Lin, S. Design of a high-performance electrocatalyst for N2 conversion to NH3 by trapping single metal atoms on stepped CeO2. ACS. Appl. Mater. Interfaces. 2019, 11, 47525-34.
49. Gao, L.; Wang, F.; Yu, M.; et al. A novel phosphotungstic acid-supported single metal atom catalyst with high activity and selectivity for the synthesis of NH3 from electrochemical N2 reduction: a DFT prediction. J. Mater. Chem. A. 2019, 7, 19838-45.
50. Talib, S. H.; Yu, X.; Lu, Z.; et al. A polyoxometalate cluster-based single-atom catalyst for NH3 synthesis via an enzymatic mechanism. J. Mater. Chem. A. 2022, 10, 6165-77.
51. Li, Q.; Liu, C.; Qiu, S.; et al. Exploration of iron borides as electrochemical catalysts for the nitrogen reduction reaction. J. Mater. Chem. A. 2019, 7, 21507-13.
52. Ma, Z.; Cui, Z.; Xiao, C.; et al. Theoretical screening of efficient single-atom catalysts for nitrogen fixation based on a defective BN monolayer. Nanoscale 2020, 12, 1541-50.
53. Hu, J.; Tian, L.; Wang, H.; et al. Theoretical screening of single-atom electrocatalysts of MXene-supported 3d-metals for efficient nitrogen reduction. Chinese. J. of. Catal. 2023, 52, 252-62.
54. Zhang, N.; Gao, Y.; Ma, L.; et al. Single transition metal atom anchored on g-C3N4 as an electrocatalyst for nitrogen fixation: a computational study. Int. J. Hydrogen. Energy. 2023, 48, 7621-31.
55. Sahoo, S. K.; Heske, J.; Antonietti, M.; Qin, Q.; Oschatz, M.; Kühne, T. D. Electrochemical N2 reduction to ammonia using single Au/Fe atoms supported on nitrogen-doped porous carbon. ACS. Appl. Energy. Mater. 2020, 3, 10061-9.
56. Choi, C.; Back, S.; Kim, N.; Lim, J.; Kim, Y.; Jung, Y. Suppression of hydrogen evolution reaction in electrochemical N2 reduction using single-atom catalysts: a computational guideline. ACS. Catal. 2018, 8, 7517-25.
57. Zhu, H.; Hu, Y.; Wei, S.; Hua, D. Single-metal atom anchored on boron monolayer (β12) as an electrocatalyst for nitrogen reduction into ammonia at ambient conditions: a first-principles study. J. Phys. Chem. C. 2019, 123, 4274-81.
58. Feng, Z.; Tang, Y.; Chen, W.; et al. Graphdiyne coordinated transition metals as single-atom catalysts for nitrogen fixation. Phys. Chem. Chem. Phys. 2020, 22, 9216-24.
59. Xu, Z.; Song, R.; Wang, M.; Zhang, X.; Liu, G.; Qiao, G. Single atom-doped arsenene as electrocatalyst for reducing nitrogen to ammonia: a DFT study. Phys. Chem. Chem. Phys. 2020, 22, 26223-30.
60. Zhang, J.; Yang, H.; Liu, B. Coordination engineering of single-atom catalysts for the oxygen reduction reaction: a review. Adv. Energy. Mater. 2021, 11, 2002473.
61. Ikeda, T.; Aratani, N.; Osuka, A. Synthesis of extremely pi-extended porphyrin tapes from hybrid meso-meso linked porphyrin arrays: an approach towards the conjugation length. Chem. Asian. J. 2009, 4, 1248-56.
62. Nakamura, Y.; Aratani, N.; Shinokubo, H.; et al. A directly fused tetrameric porphyrin sheet and its anomalous electronic properties that arise from the planar cyclooctatetraene core. J. Am. Chem. Soc. 2006, 128, 4119-27.
63. Yang, L.; Chen, F.; Wu, M.; Song, E.; Xiao, B.; Jiang, Q. Mo decoration on graphene edge for nitrogen fixation: a computational investigation. Appl. Surf. Sci. 2021, 568, 150867.
64. Patel, A. M.; Ringe, S.; Siahrostami, S.; Bajdich, M.; Nørskov, J. K.; Kulkarni, A. R. Theoretical approaches to describing the oxygen reduction reaction activity of single-atom catalysts. J. Phys. Chem. C. 2018, 122, 29307-18.
65. Rodriguez, M. M.; Bill, E.; Brennessel, W. W.; Holland, P. L. N2 reduction and hydrogenation to ammonia by a molecular iron-potassium complex. Science 2011, 334, 780-3.
66. Wang, T.; Guo, Z.; Zhang, X.; et al. Recent progress of iron-based electrocatalysts for nitrogen reduction reaction. J. Mater. Sci. Technol. 2023, 140, 121-34.
67. Li, X. F.; Li, Q. K.; Cheng, J.; et al. Conversion of dinitrogen to ammonia by FeN3-embedded graphene. J. Am. Chem. Soc. 2016, 138, 8706-9.
68. Li, B.; Du, W.; Wu, Q.; Dai, Y.; Huang, B.; Ma, Y. Coronene-based 2D metal–organic frameworks: a new family of promising single-atom catalysts for nitrogen reduction reaction. J. Phys. Chem. C. 2021, 125, 20870-6.
69. Sippel, D.; Rohde, M.; Netzer, J.; et al. A bound reaction intermediate sheds light on the mechanism of nitrogenase. Science 2018, 359, 1484-9.
70. Liu, C.; Li, Q.; Zhang, J.; Jin, Y.; Macfarlane, D. R.; Sun, C. Conversion of dinitrogen to ammonia on Ru atoms supported on boron sheets: a DFT study. J. Mater. Chem. A. 2019, 7, 4771-6.
71. Yin, H.; Li, S.; Gan, L.; Wang, P. Pt-embedded in monolayer g-C3N4 as a promising single-atom electrocatalyst for ammonia synthesis. J. Mater. Chem. A. 2019, 7, 11908-14.
72. Chen, Z.; Zhao, J.; Cabrera, C. R.; Chen, Z. Computational screening of efficient single-atom catalysts based on graphitic carbon nitride (g-C3N4) for nitrogen electroreduction. Small. Methods. 2019, 3, 1800368.
73. Wang, M.; Huang, Y.; Ma, F.; et al. Theoretical insights into the mechanism of nitrogen-to-ammonia electroreduction on TM/g-C9N10. Mol. Cataly. 2023, 547, 113391.
74. Saeidi, N.; Esrafili, M. D.; Sardroodi, J. J. Electrochemical reduction of N2 to NH3 using a co-atom stabilized on defective N-doped graphene: a computational study. ChemistrySelect 2019, 4, 12216-26.
75. Wu, J.; Li, J. H.; Yu, Y. X. Single Nb or W atom-embedded BP monolayers as highly selective and stable electrocatalysts for nitrogen fixation with low-onset potentials. ACS. Appl. Mater. Interfaces. 2021, 13, 10026-36.
76. Gao, Y.; Yang, Y.; Hao, L.; et al. Single Nb atom modified anatase TiO2(110) for efficient electrocatalytic nitrogen reduction reaction. Chem. Catal. 2022, 2, 2275-88.
77. Li, Q.; Qiu, S.; Liu, C.; et al. Computational design of single-molybdenum catalysts for the nitrogen reduction reaction. J. Phys. Chem. C. 2019, 123, 2347-52.
78. Jiao, D.; Liu, Y.; Cai, Q.; Zhao, J. Coordination tunes the activity and selectivity of the nitrogen reduction reaction on single-atom iron catalysts: a computational study. J. Mater. Chem. A. 2021, 9, 1240-51.
79. Wang, X.; Yang, L. Efficient modulation of the catalytic performance of electrocatalytic nitrogen reduction with transition metals anchored on N/O-codoped graphene by coordination engineering. J. Mater. Chem. A. 2022, 10, 1481-96.
80. Tang, Y.; Wang, Y.; Cheng, X.; Zhang, H. Single vanadium atom achored on sulfur-doped graphene as an efficient electrocatalyst toward the nitrogen reduction reaction: a computational view. J. Phys. Chem. C. 2023, 127, 24574-82.
81. Di Liberto G, Giordano L, Pacchioni G. Predicting the stability of single-atom catalysts in electrochemical reactions. ACS. Catal. 2024, 14, 45-55.
82. Chu, Z.; Kang, X.; Duan, X. Single metal atom anchored on a CN monolayer as an excellent electrocatalyst for the nitrogen reduction reaction. Phys. Chem. Chem. Phys. 2021, 23, 2658-62.
83. Song, R.; Yang, J.; Wang, M.; et al. Theoretical study on P-coordinated metal atoms embedded in arsenene for the conversion of nitrogen to ammonia. ACS. Omega. 2021, 6, 8662-71.
84. Wang, X.; Zhang, Q.; Hao, W.; Fang, C.; Zhou, J.; Xu, J. A novel porous graphitic carbon nitride (g-C7N3) substrate: prediction of metal-based π–d conjugated nanosheets toward the highly active and selective electrocatalytic nitrogen reduction reaction. J. Mater. Chem. A. 2022, 10, 15036-50.
85. Song, W.; Xie, K.; Wang, J.; Guo, Y.; He, C.; Fu, L. Density functional theory study of transition metal single-atoms anchored on graphyne as efficient electrocatalysts for the nitrogen reduction reaction. Phys. Chem. Chem. Phys. 2021, 23, 10418-28.
86. Zhao, Y.; Qu, J.; Li, H.; et al. Atomically dispersed uranium enables an unprecedentedly high NH3 yield rate. Nano. Lett. 2022, 22, 4475-81.
87. Meng, Q.; Liu, L.; Song, D.; Wang, S.; Qin, R.; Fu, G. Flexible iron clusters promoting ammonia synthesis: a density functional theory prediction. J. Phys. Chem. Lett. 2024, 15, 10623-8.
88. Li, R.; Wang, D. Superiority of dual-atom catalysts in electrocatalysis: one step further than single-atom catalysts. Adv. Energy. Mater. 2022, 12, 2103564.
89. Qian, Y.; Liu, Y.; Zhao, Y.; Zhang, X.; Yu, G. Single vs double atom catalyst for N2 activation in nitrogen reduction reaction: a DFT perspective. EcoMat 2020, 2, e12014.
90. Chen, Z. W.; Yan, J.; Jiang, Q. Single or double: which is the altar of atomic catalysts for nitrogen reduction reaction? Small. Methods. 2019, 3, 1800291.
91. Zhang, X.; Chen, A.; Zhang, Z.; Zhou, Z. Double-atom catalysts: transition metal dimer-anchored C2N monolayers as N2 fixation electrocatalysts. J. Mater. Chem. A. 2018, 6, 18599-604.
92. Guo, R.; Hu, M.; Zhang, W.; He, J. Boosting electrochemical nitrogen reduction performance over binuclear Mo atoms on N-doped nanoporous graphene: a theoretical investigation. Molecules 2019, 24, 1777.
93. Zhang, Z.; Xu, X. g-C3N4-supported metal-pair catalysts toward efficient electrocatalytic nitrogen reduction: a computational evaluation. Adv. Theory. Simul. 2022, 5, 2100579.
94. Yang, Y.; Hu, C.; Shan, J.; et al. Electrocatalytically activating and reducing N2 molecule by tuning activity of local hydrogen radical. Angew. Chem. Int. Ed. Engl. 2023, 62, e202300989.
95. Wu, Y.; He, C.; Zhang, W. Building up a general selection strategy and catalytic performance prediction expressions of heteronuclear double-atom catalysts for N2 reduction. J. Energy. Chem. 2023, 82, 375-86.
96. Zheng, X.; Yao, Y.; Wang, Y.; Liu, Y. Tuning the electronic structure of transition metals embedded in nitrogen-doped graphene for electrocatalytic nitrogen reduction: a first-principles study. Nanoscale 2020, 12, 9696-707.
97. He, T.; Puente, S. A. R.; Du, A. Atomically embedded asymmetrical dual-metal dimers on N-doped graphene for ultra-efficient nitrogen reduction reaction. J. Catal. 2020, 388, 77-83.
98. Li, Y.; Zhang, Q.; Li, C.; et al. Atomically dispersed metal dimer species with selective catalytic activity for nitrogen electrochemical reduction. J. Mater. Chem. A. 2019, 7, 22242-7.
99. Zheng, G.; Li, L.; Hao, S.; Zhang, X.; Tian, Z.; Chen, L. Double atom catalysts: heteronuclear transition metal dimer anchored on nitrogen-doped graphene as superior electrocatalyst for nitrogen reduction reaction. Adv. Theory. Simul. 2020, 3, 2000190.
100. Ma, D.; Zeng, Z.; Liu, L.; Jia, Y. Theoretical screening of the transition metal heteronuclear dimer anchored graphdiyne for electrocatalytic nitrogen reduction. J. Energy. Chem. 2021, 54, 501-9.
101. Cui, C.; Zhang, H.; Cheng, R.; Huang, B.; Luo, Z. On the nature of three-atom metal cluster catalysis for N2 reduction to ammonia. ACS. Catal. 2022, 12, 14964-75.
102. Liu, J. C.; Ma, X. L.; Li, Y.; Wang, Y. G.; Xiao, H.; Li, J. Heterogeneous Fe3 single-cluster catalyst for ammonia synthesis via an associative mechanism. Nat. Commun. 2018, 9, 1610.
103. Chen, Z. W.; Chen, L. X.; Jiang, M.; et al. A triple atom catalyst with ultrahigh loading potential for nitrogen electrochemical reduction. J. Mater. Chem. A. 2020, 8, 15086-93.
104. Guo, R.; An, W.; Liu, M.; et al. Modulating the coordination environment of active site structure for enhanced electrochemical nitrogen reduction: the mechanistic insight and an effective descriptor. Appl. Surf. Sci. 2024, 644, 158799.
105. Han, B.; Meng, H.; Li, F.; Zhao, J. Fe3 cluster anchored on the C2N monolayer for efficient electrochemical nitrogen fixation. Catalysts 2020, 10, 974.
106. Han, B.; Li, F. Regulating the electrocatalytic performance for nitrogen reduction reaction by tuning the N contents in Fe3@NxC20-x (x = 0~4): a DFT exploration. J. Mater. Inf. 2023, 3, 24.
107. Chen, S.; Gao, Y.; Wang, W.; Prezhdo, O. V.; Xu, L. Prediction of three-metal cluster catalysts on two-dimensional W2N3 support with integrated descriptors for electrocatalytic nitrogen reduction. ACS. Nano. , 2023, 1522-32.
108. Pei, W.; Zhang, W.; Yu, X.; et al. Computational design of spatially confined triatomic catalysts for nitrogen reduction reaction. J. Mater. Inf. 2023, 3, 26.
109. Pei, W.; Hou, L.; Yu, X.; et al. Graphitic carbon nitride supported trimeric metal clusters as electrocatalysts for N2 reduction reaction. J. Catal. 2024, 429, 115232.
110. Zhang, H.; Cui, C.; Luo, Z. MoS2-supported Fe2 clusters catalyzing nitrogen reduction reaction to produce ammonia. J. Phys. Chem. C. 2020, 124, 6260-6.
111. Anis, I.; Amin, S.; Rather, G. M.; Dar, M. A. N2 activation and reduction on graphdiyne supported single, double, and triple boron atom catalysts: a first principles investigation. ChemistrySelect 2023, 8, e202300993.
112. Wang, X.; Lin, L.; Li, B. First principles study of double boron atoms supported on graphitic carbon nitride (g-C3N4) for nitrogen electroreduction. Crystals 2022, 12, 1744.
113. Rasool, A.; Anis, I.; Bhat, S. A.; Dar, M. A. Optimizing the NRR activity of single and double boron atom catalysts using a suitable support: a first principles investigation. Phys. Chem. Chem. Phys. 2023, 25, 22275-85.
114. Hu, Y.; Chen, J.; Wei, Z.; He, Q.; Zhao, Y. Recent advances and applications of machine learning in electrocatalysis. J. Mater. Inf. 2023, 3, 18.
115. Lu, Y.; Fang, C.; Zhang, Q.; et al. Single-atom catalytic N2 fixation with integrated descriptors on a novel π-π conjugated graphitic carbon nitride (g-C13N15) platform obtained by self-doping design: prediction of ultrahigh activity and desirable selectivity. J. Power. Sources. 2024, 595, 234030.
116. Lv, X.; Wei, W.; Huang, B.; Dai, Y.; Frauenheim, T. High-throughput screening of synergistic transition metal dual-atom catalysts for efficient nitrogen fixation. Nano. Lett. 2021, 21, 1871-8.
117. Sun, J.; Xia, P.; Lin, Y.; et al. Theoretical exploration of the nitrogen fixation mechanism of two-dimensional dual-metal TM1TM2@C9N4 electrocatalysts. Nanoscale. Horiz. 2023, 8, 211-23.
118. Sun, J.; Chen, A.; Guan, J.; et al. Interpretable machine learning-assisted high-throughput screening for understanding NRR electrocatalyst performance modulation between active center and C-N coordination. Energy. Environ. Mater. 2024, 7, e12693.
119. Zhang, S.; Lu, S.; Zhang, P.; et al. Accelerated discovery of single-atom catalysts for nitrogen fixation via machine learning. Energy. Environ. Mater. 2023, 6, e12304.
120. Zhang, Y.; Wang, Y.; Ma, N.; Fan, J. Directly predicting N2 electroreduction reaction free energy using interpretable machine learning with non-DFT calculated features. J. Energy. Chem. 2024, 97, 139-48.
121. Chen, C.; Cao, J.; Yin, W.; Zhang, Q.; Yao, Y.; Wei, X. Single transition metal atom modified MoSe2 as a promising electrocatalyst for nitrogen fixation: a first-principles study. Chem. Phys. Lett. 2021, 780, 138939.
122. Ma, D.; Zeng, Z.; Liu, L.; Huang, X.; Jia, Y. Computational evaluation of electrocatalytic nitrogen reduction on TM single-, double-, and triple-atom catalysts (TM = Mn, Fe, Co, Ni) based on graphdiyne monolayers. J. Phys. Chem. C. 2019, 123, 19066-76.
123. Ren, C.; Jiang, Q.; Lin, W.; Zhang, Y.; Huang, S.; Ding, K. Density functional theory study of single-atom V, Nb, and Ta catalysts on graphene and carbon nitride for selective nitrogen reduction. ACS. Appl. Nano. Mater. 2020, 3, 5149-59.
124. Luo, Y.; Li, M.; Dai, Y.; et al. Transition metal-modified Co4 clusters supported on graphdiyne as an effective nitrogen reduction reaction electrocatalyst. Inorg. Chem. 2021, 60, 18251-9.
125. Liu, S.; Liu, J. Rational design of highly efficient electrocatalytic single-atom catalysts for nitrogen reduction on nitrogen-doped graphene and g-C2N supports. J. Power. Sources. 2022, 535, 231449.
126. Wang, J.; Luo, Z.; Zhang, X.; et al. Single transition metal atom anchored on VSe2 as electrocatalyst for nitrogen reduction reaction. Appl. Surf. Sci. 2022, 580, 152272.
127. Zhang, Y.; Wang, X.; Liu, T.; et al. Charge and spin communication between dual metal single-atom sites on C2N sheets: regulating electronic spin moments of Fe atoms for N2 activation and reduction. J. Mater. Chem. A. 2022, 10, 23704-11.
128. Nong, W.; Qin, S.; Huang, F.; et al. Designing C3N-supported single atom catalysts for efficient nitrogen reduction based on descriptor of catalytic activity. Carbon 2021, 182, 297-306.
129. Zheng, X.; Liu, Y.; Yao, Y. Trimetallic single-cluster catalysts for electrochemical nitrogen reduction reaction: activity prediction, mechanism, and electronic descriptor. Chem. Eng. J. 2021, 426, 130745.
Cite This Article

How to Cite
Download Citation
Export Citation File:
Type of Import
Tips on Downloading Citation
Citation Manager File Format
Type of Import
Direct Import: When the Direct Import option is selected (the default state), a dialogue box will give you the option to Save or Open the downloaded citation data. Choosing Open will either launch your citation manager or give you a choice of applications with which to use the metadata. The Save option saves the file locally for later use.
Indirect Import: When the Indirect Import option is selected, the metadata is displayed and may be copied and pasted as needed.
About This Article
Special Issue
Copyright
Data & Comments
Data
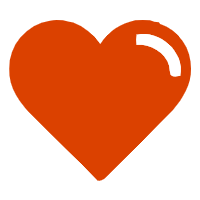
Comments
Comments must be written in English. Spam, offensive content, impersonation, and private information will not be permitted. If any comment is reported and identified as inappropriate content by OAE staff, the comment will be removed without notice. If you have any queries or need any help, please contact us at [email protected].