Bioaccumulation, transfer, and impacts of microplastics in aquatic food chains
Abstract
Plastics have become a pervasive global contaminant since the mid-20th century, causing harm to organisms at all levels. Preventative measures to reduce plastic pollution and awareness-raising campaigns about the damaging effects of plastic debris on the environment and its inhabitants are crucial; however, most plastic assessments focus on singular trophic levels. Microplastics, tiny plastic particles ranging from 25 μm to 5 mm, have emerged as a widespread form of pollution found in ecosystems worldwide. They can enter the environment directly or through the breakdown of larger plastic debris and are thought to be mistaken for food by foraging animals. This leads to microplastics circulating through ecosystems via direct and indirect consumption, ultimately impacting even higher-order predators. Here, we assess the impacts of microplastics on Chlorophyll a concentrations, algal community structure, copepod survivorship, and fish behavior in experimental trials, in addition to simulated top-predator foraging success on plastic-exposed fish. Our results indicate that microplastics have detrimental effects on algal growth and copepod survival. We also observed the trophic transmission of small plastic spheres from copepods to fish predators, highlighting a concerning pathway for microplastic pollution within aquatic ecosystems, where fish consumed plastics through direct and indirect means. Primary consumers, like copepods, face dual pressures from top-down forcing, as they are preferred over plastic particles as food sources, and bottom-up resource depletion, as algal food supplies can be limited by microplastic exposure. Our findings demonstrate the system-wide impacts that can occur when microplastics are included in food chains and underscore the urgent need for comprehensive strategies to mitigate the entry of plastic debris into aquatic ecosystems.
Keywords
INTRODUCTION
The widespread use of plastics for durable and single-use products has spurred their entry into the environment as waste, making them now a ubiquitous feature in all habitats and a global threat to wildlife[1-4]. The momentum of plastic production persists without weakening: half of all plastics ever produced were manufactured in approximately the last 15 years[5]. Plastics are petroleum-based products, and the most common debris found in the environment is comprised of Low-density Polyethylene, High-density Polyethylene (HDPE), Polypropylene, or Polystyrene[1,6,7]. While plastics enter the environment in many forms and size/length classifications, we focus here on microplastics (25 μm - 5 mm)[8,9] and their role as resources (substrate, food, and a form of satiation) in food webs.
Microplastics enter the environment as either primary contaminants, such as industrially manufactured microbeads from personal care products[1,6], or secondary contaminants resulting from the breakdown of larger plastic pieces through weathering processes[1,10]. The size of microplastics influences their bioaccessibility, the degree to which they can be incorporated into organisms, and what trophic levels they impact[11].
The ingestion of microplastics can happen through direct[12] or indirect pathways of consumption[11]. Direct consumption occurs when organisms confuse plastics for digestible food items and intentionally eat them[12], acquire them while indiscriminately filter feeding[13,14], or incidentally swallow them while preening or drinking[15]. Indirect feeding occurs when organisms eat a prey item that has interacted with (consumed or become entangled in) microplastics[11], promoting the accumulation of microplastics in higher-level organisms[16]. Foundational works on contaminants in the environment provide evidence of biomagnification of dissolved chemicals and heavy metals in food webs[17-19]. As microplastics degrade in the environment or within an organism following consumption[20], they can release toxic chemicals[21]. Many of these compounds are endocrine-disrupting and induce metabolic disorders, oxidative stress, and altered gene expression[21,22]. Plastic congeners released into the environment can also bioaccumulate and biomagnify, similar to traditional chemical contaminants found in the environment, such as mercury and organochlorines[23-25]. Moreover, plastic debris attracts and accumulates other hazards on their surfaces, such as heavy metals, viruses, antibiotics, and other toxic chemicals[26]. As weathered plastics settle into sediments, their adsorbed pollutants are transported along with them[26]. Thus, plastic particles could be considered transport vehicles that move harmful molecules around habitat compartments[26].
Plastics and their congeners have similar risks to traditional chemical contaminants, but there are additional hazards associated with their physical attributes like entanglement, blockages, and taking up physical space in the gut that induces the feeling of satiation. The high density and uncompressible volume of plastics can physically block or impede the movement of digestate through the gastrointestinal (GI) tract[27]. Total blockages of the GIT can cause starvation, weakness, and death[28]. Besides blockages, plastics can cause abrasions and ulcerations as they move through the GIT[28]. Therefore, in addition to the well-documented biological impacts of chemical disruption extensively covered in the ecotoxicology literature, plastics pose an even greater danger associated with their morphological properties.
Traditionally, assessments of microplastics have focused on organisms that consume the particles; however, basal species like algae must also be considered[29]. Algae can grow in clumped or aggregated colonies, known as biofilms, on the surfaces of rocks, other organisms, sediments, and the water’s surface[30]. Algal productivity can increase measurably when hard substrates for growth are abundant[30]. Microplastics could provide the surface area necessary for biofilms to form and proliferate in aquatic systems when other substrates are limited[29]. Biofilms on microplastics increase the adsorption of pollutants[31] and increase the surface area of microplastics through the degradation of the surfaces they colonize, increasing the potential for plastic toxicity exposure[29]. Chemicals infuse into the tissues of algae that colonize the microplastics, which, in turn, can negatively impact photosynthesis[29]. Consequently, the productivity of biofilm-forming algae exposed to microplastics is ambiguous, as the toxic properties of the particles could undermine the significance of spatial resources for autotrophs[32].
The presence of biofilms on microplastics can disguise their identity and encourage the ingestion of plastics by organisms[29]. For example, Savoca et al. found seabirds responded positively to the dimethyl sulfide (DMS) signature emitted by biofilms on microplastics and preferentially consumed biofilm-wrapped plastics[33]. Procter et al. found similar DMS signature preferences in copepod foragers[34].
Copepods occur in most freshwater and marine ecosystems and are important dietary items for many consumers, accounting for over 90 % of the diet of some species[35]. Copepods are widely used as prey for fish larvae in aquaculture[36,37] and are readily available for purchase and use in laboratory settings. Previous works documented the ingestion of microplastics by copepods[38-41] and their immediate, second-order consumers like jellyfish[42] and mysid shrimp[43]. This evidence suggests that copepods can and will consume microplastics, and their predators - including many species of fish[44-46] - also ingest plastics when they feed on copepods, acquiring plastics through indirect pathways.
Microplastic impacts on fish are of particular concern to human societies because seafood accounts for ~17% of all animal protein that we eat[47] and commercially fished species have been reported to contain microplastics[48,49]. The consequences of plastic in our food chain parallel those reported in wildlife: accumulations of microplastics in the body can induce blockages and physiochemical disorders associated with the biotransformation of toxic chemicals[50,51]. Thus, plastics in food webs are an important modern contaminant worthy of study regardless of the target population (human or wildlife) of concern. Here, we investigate the transport of plastics in food webs and their impacts on three trophic levels: basal species (chlorophytic algae), primary consumers (copepods), and secondary consumers (fish), with assertions for impacts on top predators - a fourth trophic level.
EXPERIMENTAL
Exposure replicate arrangements
Over 17 months, we performed 7 types of experiments: 126 replicates across 3 distinct trial periods with 3-5 replicate jars per treatment [Table 1]. We performed trials under various combinations of environmental conditions [artificial seawater (Instant Ocean at 25-38 psu) vs. freshwater (distilled water)], copepod consumers (small-bodied marine copepods - Apocyclops panamensis, large-bodied marine copepods - Tigriopus californicus and freshwater Cyclops sp.), and four different types of plastic - either microspheres [high-density fluorescently-labeled polyethylene spheres in orange and yellow, with diameters of 25, 70, and 550 µm (UVPMS-BY2-1.00 & UVPMS-BO & S-BY2, Cospheric, Galeta, CA, USA), hereafter referred to as “polyspheres”] or microfibers [~3 mm long 500 µm diameter polystyrene, shaved from 10 mm beads
Treatment codes and components for algal growth and copepod survivorship trials
Treatment ID | N | Tween80 | Microplastic type | Plastics in jar | Plastics surface area (mm2) | Surface area in jar (mm2) |
MT | 9 | Yes | Smallest polyspheres | 174,250 | 0.002 | 342 |
CT | 9 | No | None | 0 | 0 | 0 |
Ms | 15 | Yes | Small polyspheres | 7,940 | 0.015 | 122 |
Ml | 15 | Yes | Large polyspheres | 16 | 0.95 | 160 |
Mf | 15 | Yes | Microfibers | 4 | 12.3 | 60 |
C | 15 | Yes | None | 0 | 0 | 0 |
Polyspheres achieved neutral buoyancy in a concentrated stock solution of 0.1 g of plastic in 50 g of water with the addition of 0.1 g of the surfactant Tween 80 (See Supplementary Materials for Tween toxicity trial information). All stock solutions remained in centrifuge tubes away from ambient light to reduce the likelihood of algal growth. To standardize the quantity of plastic available in all trials, we used a consistent weight of microplastic (0.1 g) to make the concentrated stock solutions, regardless of shape or size. To estimate the surface area of each plastic treatment (surface area in jar), we used the midrange for the diameter and number of polyspheres[52], along with the calculated surface area and density (Equation 1 in the Supplementary Materials) for individual polyspheres and plastic microfibers[52]. In doing this, we estimated that the surface area of small polyspheres was approximately 65 times greater than that of large spheres and large polyspheres had about 13 times the surface area of plastic microfibers [Table 1]. For comparison’s sake, we assumed that the surface area, density, and number of microplastics in control treatments (without experimental plastics added) were zero and confirmed this in all trials. In separate
All trials occurred in an environmental chamber, which remained at a steady temperature (25 °C) with lights programmed on a 16:8 light: dark sequence (432-watt 4-bulb fluorescent fixture over a wire rack) and 1 h of twilight (100-watt UV bulb) on each side of the simulated day. We kept two copepod populations in separate housing glass jars (2 L, Mason) to mitigate the possibility of both populations crashing simultaneously. The housing jars were aerated by a glass pipet (13-665G, Fisher Scientific, Waltham, MA, USA) linked with silicone tubing to a 4-valve aeration pump (ActiveAqua, Chico, CA, USA) by metal 4-way air control valves (Bnafes, Mississauga, ON, CA). Aeration ensured that the water remained oxygenated, and we adjusted bubble rates to allow for air to constantly be diffused into the water, but not so turbulent that copepods could not orient themselves (40-200 bubbles/min). We supplemented copepods with algae for food and provided an IceCap Pod Habitat (CoralVue, Slidell, LA, USA) for shelter.
There were 3-5 replicate jars for each respective treatment in all trials. Every trial occurred in a 200 mL glass jar fitted with a cork top (7 oz Pudding Jar, Folinstall, Houston, TX, USA) that we drilled a hole in to fit a glass pipet linked to our aerator system. All treatments contained a commercially available mix of four different algal species (Nannochloropsis gaditana, Tetraselmis sp., Isochrysis galbana, and Thalassiosira weissflogii, Algaebarn, Commerce City, CO, USA). Some algae were more likely to persist as single-celled, swimming individual cells, while others had a propensity to develop into clumps of biofilm that could adhere and, subsequently, slough off substrates, like the glass walls of the jar or microplastic particles.
Primary producer and consumer assessments
All experimental exposure trials (algal, copepod consumers, fish predators) required a running stock of algae for subsistence. For the basal trophic level, productivity (Chlorophyll a concentration) and the composition (living, dead, single-celled, and clumping) of the algal community were assessed and we considered these to be estimates of net primary productivity. For the first-order consumers, we used copepods and tracked their survivorship. Additionally, copepod and plastic prey were maintained in the presence of algae as a food source or growth substrate before being offered to fish during feeding trials.
To test for algal productivity in the presence of microplastics, we allowed primary producers to grow in replicated jars for twelve days. Each algal trial consisted of twelve sample jars: three of each treatment, randomly arranged to reduce error from spatial bias. The jars contained 147 mL of water, 1.5 mL of experimental stock, and 1.5 mL of algal stock. Every three days, 50 mL of water from each jar was filtered on a pre-muffled glass fiber filter (1820-047, Whatman 1.6 μm, Tisch Scientific, Cleves, OH, USA). We wrapped filters in aluminum foil and froze them for downstream processing. After filtering, 49 mL of water, 0.5 mL of experimental stock, and 0.5 mL of stock algae were added back to each jar to replace the water volume and initial treatment concentrations. We filtered the sample jars every three days (21 days total), yielding repeated filter metrics that we averaged for each replicate jar.
We used three genera of copepods in our trials. For the initial Tween80 toxicity trials [Supplementary Materials], we used the small-bodied marine Apocyclops panamensis and the smallest-sized (25 mm diameter) polyspheres. In primary consumer trials, we used the large-bodied marine Tigriopus californicus, with the large polyspheres (550 mm diameter) and the small polyspheres (70 mm diameter). For the predator fish feeding trials, we used the large-bodied freshwater Cyclops sp. (Carolina Biological, Burlington, NC, USA), the small (70 mm diameter) and large (550 mm diameter) polyspheres. To ensure sufficient algae for copepod feeding, we first cultivated algae in the replicate jars for ten days without copepods, then introduced 20 adult copepods to each replicate (excluding controls). On that day, and every 3-4 days over the next eleven days, we performed a filtration with replacement to ensure no copepods were lost in the
Primary productivity
To estimate algal relative net primary productivity (RNPP), we calculated the Chlorophyll a concentration for each sample jar. We extracted Chlorophyll a from algal filtrate on previously frozen filters in 90% acetone (Certified ACS grade, Fisher Scientific, Waltham, MA, USA) and agitated them in individual centrifuge tubes by hand (shaken for 3-5 min) until the filter was completely degraded, then centrifuged at 3,000 rpm for 5 min (RCF = 2,006 g)[54]. A Spectrophotometer (Thermo Scientific Spectronic Genesys 2, Waltham, MA, USA) measured the absorbance of 2 mL of supernatant for each sample at 470, 645, 662, and 750 nm. We used a correction factor to estimate Chlorophyll a concentrations to account for the light transmission of the solvent[55]. To understand the relative ecological impact (if present) of microplastics on algal communities, we calculated the average percent change in [Chlorophyll a] between each filtration period - a quantification of the change in primary productivity (NPP). Accounting for copepod consumption of algae, the percent change was divided by the number of copepods in the jar during the later filtration period, giving a comparable value for the RNPP.
Algal community phenotypes
In addition to Chlorophyll a concentrations, we assessed the components of the algal community for any changes in algal-type frequencies. We viewed a subsample of each replicate algal treatment on a hemocytometer and enumerated the types of algae that were present (single cells, clumps, living, and dead). On every filtration day, we transferred 1 mL aliquots of water from each replicate jar to a glass spot plate with 1 mL of 0.4% Trypan Blue to stain (dead) cells for differentiation between living and dead cells[56]. We flooded the surface of the hemocytometer with 20 μL stain-treated algal aliquot and counted the number of single cells, clumped cells, living (unstained), and dead (stained) cells, then multiplied by 5,000 to estimate the frequencies of each type of cell per mL in each replicate jar[57].
Secondary consumer assessments
Fish collection and housing
We collected Eastern blacknose dace (Rhinichthys atratulus) from Sand Spring Run in Frostburg, Maryland, USA, from April-August 2023, following IACUC protocols and collection permit requirements (FSU:PFHE-01 SOP, FSU:IACUC2021-003, 2022-04, MD State 202259). Baited (sardines) minnow traps soaked in the creek for 4 to 24 hours and were emptied at 4 h intervals. We transferred fish from traps to the laboratory facility in coolers filled with water from the creek within 15 min after recovery. The fish remained aerated in the cooler of creek water for ~48 h in the same environmental chamber in which copepod trials occurred. Over this acclimation period, we added holding tank water (tanks the fish lived in while awaiting trials) to the cooler every 8 h until the volume tripled. After acclimation and removal of any visibly diseased or unusual individuals (humanely euthanized according to IACUC protocol: FSU: PFHE-01 SOP, FSU: IACUC2021-003, 2022-04), we moved experimental candidate fish to one of two identical 50 gal aquaria in the environmental chamber. Each holding tank contained ~45 gal of water and was constantly filtered using a Lifegard Hang-On Filter (Lifegard Aquatics, Santa Fe Springs, CA). We replenished the water in the tank with distilled tap water as needed to abate evaporation and provided fish in the holding tanks with copepods every other day to habituate fish to the size and movements of a digestible, natural prey item. A third 25 gal tank housed fish for a 48 h starvation period that preceded each trial. We transferred fish between acclimation, housing, and starvation tanks using 2 mm nylon mesh 12 cm × 8 cm wire and rubber-handled fish nets.
Fish predatory trials
Individual fish were placed into one of four identical 3 L square, glass aquaria that contained 2 L of water and were allowed 60 min to acclimate to the new environment (aerated but unfiltered/uncirculated water replaced for each trial). All trials were recorded on digital cameras (AKASO EK7000, Frederick, MD), positioned at two angles (one camera facing the front of the tank and the other facing the right-hand side to get 90-degree angle views on the tank) for 30 min, and we analyzed the fish feeding behavior for both angles to accumulate data for feeding behavior.
Following acclimation to the video arena tanks, predatory fish were offered food items: copepods, polyspheres, or a combination of the two. Some copepods had been cultured in the presence of polyspheres (either large 550 mm or small 70 mm), so we had four levels of possible plastic exposure for the fish. The levels of exposure for the fish included: no plastic (copepods only), indirect plastic exposure (copepods cultured in the presence of polyspheres), direct plastic exposure (copepods and polyspheres in the tank combined), and direct + indirect exposure (copepods cultured with plastic combined with polyspheres). Different combinations of direct and indirect exposure were associated with the two different sizes of polyspheres, so we completed trials using 14 different experimental treatments [Table 2]. We performed eight trials for each treatment, resulting in 112 trials assessing fish feeding behavior. When a fish was offered copepods, we placed 15 in the experimental tank. When plastics were offered to fish, we added 10 mL of the same experimental stocks of large (550 mm) and/or small (70 mm) polyspheres used for the previous algal and copepod trials.
Treatments of the fish feeding trials
Code | N | Copepod exposure | Plastic type | Plastics in tank | Plastics total SA (mm2) | Copepods in tank |
C | 8 | Control | None | 0 | 0 | 15 |
CwL | 8 | Control | Large PS | 110 | 104 | 15 |
CwS | 8 | Control | Small PS | 53,000 | 814 | 15 |
CwM | 8 | Control | Large PS & small PS | 55 & 26,500 | 50 & 407 | 15 |
LeC | 8 | Large PS | None | 0 | 0 | 15 |
LeCwL | 8 | Large PS | Large PS | 110 | 104 | 15 |
LeCwS | 8 | Large PS | Small PS | 53,000 | 814 | 15 |
LeCwM | 8 | Large PS | Large PS & small PS | 55 & 26,500 | 50 & 407 | 15 |
SeC | 8 | Small PS | None | 0 | 0 | 15 |
SeCwL | 8 | Small PS | Large PS | 110 | 104 | 15 |
SeCwM | 8 | Small PS | Large PS & small PS | 55 & 26,500 | 50 & 407 | 15 |
L | 8 | None | Large PS | 110 | 104 | 0 |
S | 8 | None | Small PS | 53,000 | 814 | 0 |
M | 8 | None | Large PS & small PS | 55 & 26,500 | 50 & 407 | 0 |
When the 30-min feeding trials were over, we attempted to capture the fish from the experimental tank using forceps while wearing a bird puppet (Folkmanis Ostrich Stage Puppet, Emeryville, CA, USA) to simulate the appearance and movement of an avian predator[58-60]. The puppet was held motionless above the tank for 10 s, and then we attempted to capture the fish every 5 s for up to 1 min (up to twelve possible capture attempts). If we did not successfully capture the fish using the forceps and puppet within 1 min, we removed the fish with a clean net. After completing the feeding trial and predation attempt, each fish was humanely euthanized following IACUC protocols (FSU: PFHE-01 SOP, FSU: IACUC2021-003, 2022-04) and frozen for later dissection.
Video analysis of fish trials
We inspected videos from both angles of all fish feeding trials (n = 224) to document several behaviors. We used lunges as a proxy for feeding behavior[61,62] and swimming activity in response to a predator for the likelihood of predation. The number of lunges and the time code of each lunge was recorded. A lunge was defined as a quick and direct forward motion. Using time code data, we calculated the antecedent time to the first lunge (latent time) to compare the general activity level of a fish, and the mean time between lunges to determine feeding rates. Further, we counted how many capture attempts were necessary to catch the fish (if caught at all in the one-minute time limit) and recorded the total number of seconds the fish moved while the bird puppet was present out of the 70 s possible. From these data, we calculated the proportion of time the fish moved in response to the puppet as a proxy for fish evasion behavior[63].
Fish gut content assessments
Following each trial, the fish were immediately frozen, and later, we thawed and dissected each to evaluate their gut contents. We removed the alimentary canal during dissection, cut it longitudinally, and spread it flat on a glass petri dish. The contents were wetted in distilled water, scraped from the gut lumen using a glass microscope slide, and transferred to a fresh glass petri dish for inspection under a dissecting microscope (Olympus SZ61, Center Valley, PA, USA). We enumerated the number of plastics, type of plastics (large/small polyspheres, fibers, and any environmental contaminants), and number of copepods consumed by each fish. Further, we visualized the gut contents under fluorescent microscopy to ensure we accurately documented all plastics. All dissecting and microscopy supplies were thoroughly cleaned between each fish to avoid any potential cross-contamination. Fish carcasses were re-frozen and saved for future assessments. To compare the number of items consumed relative to their frequencies in our experimental trials, we calculated the expected frequencies by determining the likely encounter rate of fish based on hypothetical respiration rates to estimate the volume of water that was passed over the gills [Supplementary Materials].
Statistical analysis
We ran Kruskal-Wallis tests to assess differences in the standardized net change in primary productivity and the algal community composition to evaluate the treatment effects for microplastic exposure. We evaluated whether there were differences in the frequencies of living vs. dead and single vs. clumped algal cells following exposure to microplastics with Student’s t-tests. Because our treatments contained a wide range of plastic particles, we transformed plastic number data when assessing for the influence of surface area or plastic quantities when seeking trends for plastic influence on algal communities. By taking the log of the number of plastics in each replicate jar and the square root of the total plastic surface area in each jar, we were able to meet assumptions of equal variance and better assess the relationships of these variables with the RNPP. Kruskal-Wallis tests assessed whether there were treatment-associated changes in copepod survivorship. We also ran Kruskal-Wallis tests to assess whether there were differences in fish feeding and predation response behaviors. Post hoc tests correcting p for the frequencies of pairwise assessments (Tukey’s HSD) elucidated the differences among treatments when Kruskal-Wallis tests yielded significant results (P < 0.05). Finally, we compared the frequency of consumption of copepods and polyspheres relative to their abundance in experimental tanks with Chi-square analyses and linear regression to learn whether fish preferentially targeted or avoided certain objects.
RESULTS AND DISCUSSION
Algal exposure trials
We detected decreased RNPP (percent change of Chlorophyll a content standardized by copepod density) in treatments where algae were exposed to any type of polysphere, but not in plastic microfiber treatments (F = 3.16, DF = 3, P = 0.029, Figure 1). Because we used equivalent plastic weights (i.e., volume) in each trial, the volume of plastic did not differ between treatment types [Table 2]. However, the surface areas to which algae could adhere and from which plastic congeners could leach differed, as shape and diameter varied among the different microplastic types used here. Algal RNPP was changed along the range of surface areas tested here (F = 6.61, P = 0.012, R2 = 0.070) and based on the total number of plastics in each replicate jar
Figure 1. RNPP estimates (proportional change in Chlorophyll a concentrations divided by the number of copepod consumers) for control and plastic (microfibers, 70 mm plastic spheres, and 550 mm plastic spheres) exposure trials. Letters above the standard error bars indicate significant differences in RNPP based on Tukey’s HSD pairwise post hoc analyses. RNPP: Relative net primary productivity; PS: polyspheres.
Figure 2. Algal community composition (represented by live single cells) of treatments with microplastics (M) and those without (C). Letters above the standard error bars represent significantly different means.
Unlike previous assessments of primary producers exposed to plastics[29], our findings suggest that the microplastics used at four different surface area to volume ratios (polyspheres with diameters of 25, 70, and 550 µm, and microfibers that were ~3,000 µm long × 500 µm diameter; Table 1) inhibited algal growth. We hypothesized an increased surface area from plastics would increase algal productivity, as they provided more substrate. Consequently, we expected the number of cells and concentration of Chlorophyll a would increase as trials with microplastics progressed. On the contrary, we found lower RNPP when polyspheres were present. However, the experimentally exposed plastic microfibers did not inhibit or promote algal growth, with productivity falling between the controls and the polysphere treatments [Figure 1]. These unexpected findings in the treatments of polyspheres could stem from plastic toxicity that reduced the fitness of algae growing on the plastics as biofilm or simply from being in water where plastics were abundant[21].
Because live single cells within the community are the phenotypic opposite of biofilms - algae that form aggregated clumps on the surfaces of water or floating/submerged objects[30] - it appears that biofilms that attempt to colonize the surfaces of microplastics do not survive, and the remaining single motile algal cells are what predominantly remain in the algal community [Figure 2]. These results could reflect the toxicity of the microplastics that biofilms formed on; however, we cannot speak to the toxic impacts in the context of our study.
Copepod feeding treatments
Algae served as the base of the aquatic food chains assessed here, and while evaluating the impacts of microplastics on algal communities, we also assessed whether these conditions influenced the survivorship of primary consumers. The survivorship of copepods (mean percent change) over a 3- or 4-day period differed by treatment (DF = 3, P = 0.007, Figure 3), where the population sizes of copepods in the control treatments increased more than those exposed to polyspheres (small and large) but were similar to those in the plastic microfiber trials.
Figure 3. Relative survivorship of the copepods (mean % change over 3-4 day periods) for control and plastic (microfibers, 70 mm plastic spheres, and 550 mm plastic spheres) exposure trials. Letters above the standard error bars indicate significant differences based on Tukey’s HSD pairwise post hoc analyses. PS: Polyspheres.
Because the presence of plastics limited algal growth and plastics can release/adsorb toxic compounds[21], our findings of overall lower copepod survival rates are compelling. While this result was relatively straightforward for polysphere exposures, copepods in plastic microfiber treatments had similar survival rates to control treatments without plastics. Consequently, the questionable responses to plastic microfibers justified their exclusion from trials at higher trophic levels.
Copepod populations could decrease in the presence of microplastics if: the plastic, when consumed, physically blocks the GIT and leads to the starvation of the copepods; the consumed plastics are toxic and impair the function of internal systems, ultimately reducing the fitness of the copepods; consumed biofilms growing on the plastics are toxic to the copepods and fitness is reduced or lost; or chemicals leeching from on/in the plastics are toxic to copepods. Because our findings align with those of the primary producer assessments, increased algal growth in the control and plastic microfiber treatments could support a larger population of copepod food (i.e., algae). We suggest that the numerical response observed here occurred as a consequence of increased nutritional resources in the absence of polyspheres. In polysphere-exposed treatments, there were fewer algae, and although a combined effect of toxicity and starvation on copepod survival is possible, we could not directly assess that here. However, we assert that the differences in the algal abundance are more impactful to copepod suppression than plastic-associated direct toxicity - whether that is associated with toxic effects remains to be definitively assessed.
Fish feeding trials
Foraging behavior
In this system, fish represented second-order consumers: a key link to understanding the potential transfer of microplastics between basal and higher trophic levels. By assessing three components of foraging behavior (feeding latency, feeding frequency, and consumed items), we could address the likelihood of fish transferring plastics to other organisms that might consume them, such as birds and humans. We detected no difference in feeding latency during the 30-min feeding trials (F = 0.91, DF = 13, P = 0.549). Thus, initial feeding attempts occurred at similar times regardless of the type of foods (copepods, plastics, or a combination) we offered.
We evaluated the number of lunges as an indicator of intentional feeding behavior as opposed to passive consumption of plastics. We detected a difference in fish lunging behavior between those with and without copepod prey items present. Fish lunge rates were higher (F = 9.64, DF = 13, P < 0.001, Figure 4) whenever copepods were present (with and without plastics) compared with trials where only plastics were offered as food. This suggests that fish feeding activity increases when visual cues such as the jerky movements of swimming copepods[61] are present.
Figure 4. The number of lunges a fish made in a 30-min trial period exposed to different plastic and copepod combinations. Three experimental conditions: trials involving only plastics (dark bars), trials involving copepods alone but with prior exposure to plastics (light bars), and trials involving a combination of plastics and copepods, with and without prior exposure to plastics (hatched bars), are presented. The outer x-axis contains labels for the type of plastic that was offered to the fish, and the inner label indicates prior exposures of the copepods offered (L: large plastic spheres; S: small plastic spheres; NP: no plastic; NC: no copepod). Letters above the standard error bars indicate significant differences based on Tukey’s HSD pairwise post hoc analyses.
Gut content analyses
Within the gut contents of fish exposed to different combinations of copepods and microplastics, we compared the frequencies of total items consumed, copepods consumed, and polysphere combinations (large, small, or a mixture of the two). The number of copepods present in the guts of fish increased with the number of lunges (P < 0.0001, R2 = 0.406, Figure 5), indicating lunging was a good proxy for intentional feeding behavior. However, the number of copepods consumed did not vary (P = 0.3877, R2 = 0.022) when plastics were visible (95% CI = 5.6-6.9) compared to when copepods were present alone but had been previously exposed to plastics (95% CI = 6.0-8.6), or when copepods had not been exposed to plastic (95% CI = 4.7-8.5). No copepods were recovered from the guts of fish for trials where copepods were not offered as food items (plastics-only trials). Despite the potential protection of copepods from dilution effects associated with microplastics, it is notable that fish did not exhibit reduced consumption of copepods in the presence of polyspheres. This observation suggests a dual response from fish: not only do they recognize copepods as viable food sources and increase their predation efforts, but they also display a preference for copepods over microplastics. While this phenomenon may not have immediate implications for fish health or fitness in terms of energetics, the diminished copepod populations responding to microplastic exposure could precipitate numerical declines in fish populations.
Similar to our findings for copepod consumption, we detected a positive relationship between the number of plastics consumed by a fish and the number of lunges it made during a 30-min feeding trial, regardless of copepod presence (all trials combined: F = 23.78, DF = 1, P < 0.001, R2 = 0.116, Figure 6). We note of particular interest, even when not directly exposed to small polyspheres, fish offered copepods that had been maintained in the presence of small polyspheres contained those plastics (F = 6.27, DF = 13, P < 0.001, Supplementary Figure 2). Thus, it appears that copepods are capable of transferring small polyspheres into the food chain. These results echo our observations, where we documented polyspheres passing through the gut of copepods (McHale, pers. obs. July 2022).
Figure 6. The number of experimental polyspheres consumed by fish during a 30-min feeding trial with and without copepods.
In trials where fish were offered copepods and both types of polyspheres (70 and 550 mm), we found an equal proportion of the two polysphere sizes present in their guts. There were more small polyspheres than large ones available in the mixed plastic trials [Table 2]; thus, we conclude the large polyspheres could have been actively targeted as food, and the small polyspheres were consumed passively during lunging actions or indirectly through activities related to respiration. Small polyspheres could adhere to the gill rakers during respiration and be eliminated or swallowed by the gills.
Within the gut contents, we find not only our experimental plastics but also environmental fiber contaminants from the ambient laboratory at a consistent rate [Supplementary Figure 1]. Microplastics have been found in every natural habitat where anyone has looked for them[64], so it was not surprising that we found non-experimental contaminants inside the fish during diet assessments. All environmental plastics found in this research were fibers, which is consistent with other evaluations of high-order consumers such as avian top predators[65-67]. In many instances, including our study, microfiber inclusion is widespread and shows little pattern of contamination that would suggest a specific route of transmittance. Nonetheless, we did not observe environmental plastics in our copepod samples. We believe they originated in the aquaria where fish were held before the feeding trials, or acquired in the stream prior to our fish collections. Because these fibers were so different in appearance from the fluorescently labeled plastics that we created our experimental plastic microfibers from, they were easy to identify. We could not determine the composition of the environmental fibers; thus, we cannot rule out the possibility that they are comprised of material other than plastic (cellulose, cotton, linen, etc.).
In addition to environmental fibers, large and small polyspheres were easily detected in the fish digestate during gut content analyses with the aid of brightfield and fluorescent microscopy. There was no evidence that copepods trophically transmitted the large (550 mm) polyspheres, but we enumerated small (70 µm) polyspheres in several trials where fish were only indirectly exposed (18.75% of applicable trials). Our study design was imperfectly nested and not all combinations of plastics and copepods could be assessed. Notably, we did not expose fish to a combination of small polyspheres and copepods that had previously been exposed to small polyspheres. We expected we would not be able to successfully disentangle the number of plastics consumed from each type of food item; however, we suggest that, if tested here, we would have seen even higher frequencies of small polyspheres in that treatment.
The inclusion of small polyspheres in the gut contents of fish that were not directly exposed to them indicates the copepods had consumed some small polyspheres, which were then transferred to fish consumers, an indirect means of plastic transmission. These findings agree with previous works, and personal observations (McHale, pers. obs. July 2022) documenting copepods ingesting microplastics[38-41]. Thus, even if higher-order predators are not intentionally eating plastics, our study demonstrates a pathway for plastic consumption through indirect means[11].
Should lunge frequencies, as opposed to feeding preferences, control the number of copepods (digestible food) and microplastics (non-digestible, potentially toxic foods), we would expect a positive association between the two. We found a weak positive relationship between copepods and plastics consumed
Previous works have indicated that the presence of plastics in fish could be the result of passive collection during respiration[68-70]. Because blacknose dace has gill rakers that are likely ineffective at collecting microplastics, the contaminants are probably expelled from the mouth. Our research has shown that the fish recognize microplastics as non-food items and avoid them. However, incidental consumption of these materials may occur while attempting to catch intended prey, such as copepods. Our findings indicate that fish primarily consume plastics unintentionally while foraging, and the quantity of microplastics in their gut is correlated with the number of consumed copepods [Supplementary Figure 3]. This suggests that incidental ingestion of microplastics is the primary cause of plastic accumulation in the guts of fish.
Top predator exposure trials
We used a bird puppet to simulate the shape and size of a predator following each feeding trial to learn whether the responses of fish when threatened by a predator changed based on fish diet (copepods, polyspheres, or a combination of the two). The number of capture attempts differed between the copepod-only trials and the small polyspheres trials, with all other trials falling into an intermediate range [Figure 7]. We also found no difference in the proportion of time the fish spent moving during the predation period among treatments (F = 1.24, DF = 13, P = 0.263). Further, the feeding activity (lunge rates) was not associated with the number of capture attempts (F = 1.93, DF = 1, P = 0.167) or the proportion of time moving (F = 2.87, DF = 1, P = 0.093). The number of plastics consumed had a weak positive relationship with the number of capture attempts (F = 4.09, DF = 1, P = 0.046, R2 = 0.0358), but was not reflected in the proportion of time spent moving (F = 1.47, DF = 1, P = 0.227, R2 = 0.013). Thus, fish that had been exposed to small plastics were harder to catch than those that had consumed copepods and had no plastic exposure.
Figure 7. Predator evasion success based on capture attempts for fish feeding trials (lower axis title), taking into consideration the type of prey (copepods, plastics, and a combination) that fish were exposed to. Three experimental conditions: trials involving only plastics (dark bars), trials involving copepods alone but with prior exposure to plastics (light bars), and trials involving a combination of plastics and copepods, with and without prior exposure to plastics (hatched bars), are presented. The outer x-axis contains labels for the type of plastic that was offered to the fish, and the inner label indicates prior exposures of the copepods offered (L: large plastic spheres; S: small plastic spheres; NP: no plastic; NC: no copepod). Letters above the standard error bars indicate significant differences based on Tukey’s HSD pairwise post hoc analyses.
In the short term, the exposure and consumption of plastics could have limited yet positive impacts on fish’s ability to respond to predators. If this trend is constant in the wild, we would expect no increase in predation on fish that consume plastics. Thus, the survivorship of fish exposed to plastics is not different from fish that did not consume plastics. However, based on our study, we cannot speak to the potential toxic impacts of plastics on the fish.
CONCLUSIONS
Ecotoxicology is a well-established field that extensively models the movement and trophic transfer of chemicals in the environment[71]. Previous works thoroughly document the impacts of these contaminants on individuals, populations, communities, and ecosystems[72-74]. However, plastics introduce additional complexities, as they can not only contain toxic congeners but also act as physical objects, causing direct damage and facilitating the transfer of other harmful substances through food webs[75]. Thus, there is precedent to expand on our knowledge of the bioaccumulation and transfer of hazardous chemicals that dissolve in water to also include contaminants that attract, magnify, and take up physical space in the environment and the organism.
Here, we demonstrate evidence of both bottom-up and top-down forcing on intermediate trophic levels, with an emphasis on first-order consumers. Algal growth was inhibited by exposure to plastic, limiting copepods’ food resources and reproductive ability. Should this be the case in the field, these critical components of food webs would have less to eat while still experiencing similar rates of predation pressure, as we documented in our fish feeding trials. Thus, plastics in the environment could negatively impact primary consumer populations in multiple ways. This, in turn, can impact interaction strengths in copepod-containing food webs and the productivity of their carnivorous consumers.
Predators that rely on copepods as prey can also experience impacts as a result of plastic exposure. With fewer copepods to feed on, fish populations would respond with lower growth rates and declines in fitness. Direct yet unintentional consumption of plastics could further inhibit fish population success. While not directly assessed here, due to the short duration of fish exposures in captivity, we suspect that, should fish have been maintained long enough in our exposure trials, their survivorship or reproductive ability could decline in the presence of plastics. Blockages of the gut and perceived satiation from a stomach full of plastics[28] could perpetuate ineffective feeding when microplastics are included in the mix of potential food items over a long period of time.
Our assessments of behavioral changes in fishes exposed to various combinations of copepods and polyspheres also allude to the potential impacts on top predators, like avian and mammalian piscivores. Because fish that were exposed to polyspheres were harder to catch, they might successfully evade predators while in good condition, which was the case in our trials. As such, small pulses of plastic in natural environments could provide higher proportions of plastic-averse prey items into the food web. However, if the condition of the fish changes due to long-term starvation and potential toxic effects from plastic exposure, they could become easier to catch. Similarly, scavengers consuming deceased fish that succumbed to starvation or toxic impacts from plastic exposure are at high risk for accumulating high levels of consumed microplastics. Therefore, plastics impact every level of the food web through direct physical interactions or indirectly through reduced food sources.
Microplastics are consumed by many organisms worldwide, including species eaten by humans, such as commercial fish and shellfish[50]. If microplastics are in our food sources, they can be passed to humans through trophic transfer[50]. This raises further concern because, like the lower organisms assessed here, humans can suffer from the physical and toxicological impacts of consumed plastics[51].
Microplastics have been reported in all environments, from the open ocean, to soils, and to the atmosphere[64]. The production of plastic materials is expected to increase as durable and single-use products have few economically appropriate alternatives. The combination of increasing plastic production and its durability signifies that it will take decades to centuries for the plastics we are currently using to break down[52]. Thus, there is a need to study plastics and their impacts on food webs (natural and human-based), in addition to engineering solutions and the adoption of biodegradable materials that will resist the negative impacts associated with their accumulation in our food sources.
Plastics are an integral, ubiquitous part of our modern society and there is no evidence that they will be easily replaced by more sustainable products[76,77]. Thus, there is a need for clear and thorough evaluations of their real and perceived impacts, their biological implications, and how they move through environments so we can devise mitigation strategies to deal with this omnipresent and persistent contaminant.
DECLARATIONS
Acknowledgments
We would like to thank our institution, College, and Department for their support and guidance. Patrycia Przybyl assisted with performing pilot studies for algal and copepod assessments. Ethan Weaver helped collect copepods and Zach Barnard assisted with fish collections and dissections. Additionally, many thanks to Dr. Cody Kent and Dr. Richard Raesly from the Department of Biology at Frostburg State University for helpful reviews of data analysis and manuscript composition.
Authors’ contributions
Conceptualization: Sheehan KL
Project execution, data curation: McHale ME
Formal analysis, investigation, writing - original draft preparation, writing - review and editing: McHale ME, Sheehan KL
Both authors have read and agreed to the published version of the manuscript.
Availability of data and materials
Data and metadata will be available on ResearchGate following the final acceptance and publication of this manuscript.
Financial support and sponsorship
This project was made possible through two major sources of funding at Frostburg State University: the FSU Incentive Fund and the FSU Foundation.
Conflicts of interest
Both authors declared that there are no conflicts of interest.
Ethical approval and consent to participate
All animal study subjects were collected and treated humanely in accordance with state, local, and international instructions for animal use and care.
Consent for publication
Not applicable.
Copyright
© The Author(s) 2024.
Supplementary Materials
REFERENCES
1. Cole M, Lindeque P, Halsband C, Galloway TS. Microplastics as contaminants in the marine environment: a review. Mar Pollut Bull 2011;62:2588-97.
2. Hale RC, Seeley ME, La Guardia MJ, Mai L, Zeng EY. A global perspective on microplastics. JGR Oceans 2020;125:e2018JC014719.
3. Stubbins A, Law KL, Muñoz SE, Bianchi TS, Zhu L. Plastics in the earth system. Science 2021;373:51-5.
4. MacLeod M, Arp HPH, Tekman MB, Jahnke A. The global threat from plastic pollution. Science 2021;373:61-5.
5. National Geographic. The world’s plastic pollution crisis explained. Available from: https://www.nationalgeographic.com/environment/article/plastic-pollution. [Last accessed on 23 May 2024].
7. Kumar M, Chen H, Sarsaiya S, et al. Current research trends on micro- and nano-plastics as an emerging threat to global environment: a review. J Hazard Mater 2021;409:124967.
8. Gigault J, Halle AT, Baudrimont M, et al. Current opinion: what is a nanoplastic? Environ Pollut 2018;235:1030-4.
9. Lechthaler S, Waldschläger K, Stauch G, Schüttrumpf H. The way of macroplastic through the environment. Environments 2020;7:73.
10. Peng J, Wang J, Cai L. Current understanding of microplastics in the environment: Occurrence, fate, risks, and what we should do. Integr Environ Assess Manag 2017;13:476-82.
11. Miller ME, Hamann M, Kroon FJ. Bioaccumulation and biomagnification of microplastics in marine organisms: a review and meta-analysis of current data. PLoS One 2020;15:e0240792.
12. Egbeocha C, Malek S, Emenike C, Milow P. Feasting on microplastics: ingestion by and effects on marine organisms. Aquat Biol 2018;27:93-106.
13. Fossi MC, Coppola D, Baini M, et al. Large filter feeding marine organisms as indicators of microplastic in the pelagic environment: the case studies of the Mediterranean basking shark (Cetorhinus maximus) and fin whale (Balaenoptera physalus). Mar Environ Res 2014;100:17-24.
14. Zantis LJ, Bosker T, Lawler F, et al. Assessing microplastic exposure of large marine filter-feeders. Sci Total Environ 2022;818:151815.
15. Bessa F, Ratcliffe N, Otero V, et al. Microplastics in gentoo penguins from the Antarctic region. Sci Rep 2019;9:14191.
16. Kuhn S, Bravo Rebolledo EL, van Franeker JA. Deleterious effects of litter on marine life. In: Bergmann M, Gutow L, Klages M, editors. Marine anthropogenic litter. Cham: Springer; 2015. pp. 75-116.
17. Monteiro LR, Costa V, Furness RW, Santos RS. Mercury concentrations in prey fish indicate enhanced bioaccumulation in mesopelagic environments. Mar Ecol Prog Ser 1996;141:21-5.
18. Morel FMM, Kraepiel AML, Amyot M. The chemical cycle and bioaccumulation of mercury. Annu Rev Ecol Syst 1998;29:543-66.
19. Chopra AK, Sharma MK, Chamoli S. Bioaccumulation of organochlorine pesticides in aquatic system--an overview. Environ Monit Assess 2011;173:905-16.
20. Sheehan KL, Lawson P, Emerson B. Fate of plastics in cattle digestive systems. J Agric Saf Health 2022;28:205-14.
21. Ma H, Pu S, Liu S, Bai Y, Mandal S, Xing B. Microplastics in aquatic environments: toxicity to trigger ecological consequences. Environ Pollut 2020;261:114089.
22. Solleiro-Villavicencio H, Gomez-De León CT, Del Río-Araiza VH, Morales-Montor J. The detrimental effect of microplastics on critical periods of development in the neuroendocrine system. Birth Defects Res 2020;112:1326-40.
23. Kidd KA, Hesslein RH, Ross BJ, Koczanski K, Stephens GR, Muir DCG. Bioaccumulation of organochlorines through a remote freshwater food web in the Canadian Arctic. Environ Pollut 1998;102:91-103.
24. Borgå K, Gabrielsen GW, Skaare JU. Biomagnification of organochlorines along a Barents Sea food chain. Environ Pollut 2001;113:187-98.
25. McIntyre JK, Beauchamp DA. Age and trophic position dominate bioaccumulation of mercury and organochlorines in the food web of Lake Washington. Sci Total Environ 2007;372:571-84.
26. Kyriakopoulos GL, Zamparas MG, Kapsalis VC. Investigating the human impacts and the environmental consequences of microplastics disposal into water resources. Sustainability 2022;14:828.
27. Jovanović B. Ingestion of microplastics by fish and its potential consequences from a physical perspective. Integr Environ Assess Manag 2017;13:510-5.
28. Wright SL, Thompson RC, Galloway TS. The physical impacts of microplastics on marine organisms: a review. Environ Pollut 2013;178:483-92.
29. Nava V, Leoni B. A critical review of interactions between microplastics, microalgae and aquatic ecosystem function. Water Res 2021;188:116476.
30. Schnurr PJ, Allen DG. Factors affecting algae biofilm growth and lipid production: a review. Renewable and Sustainable Energy Reviews 2015;52:418-29.
31. Wang J, Guo X, Xue J. Biofilm-developed microplastics as vectors of pollutants in aquatic environments. Environ Sci Technol 2021;55:12780-90.
32. Zhang C, Chen X, Wang J, Tan L. Toxic effects of microplastic on marine microalgae Skeletonema costatum: interactions between microplastic and algae. Environ Pollut 2017;220:1282-8.
33. Savoca MS, Wohlfeil ME, Ebeler SE, Nevitt GA. Marine plastic debris emits a keystone infochemical for olfactory foraging seabirds. Sci Adv 2016;2:e1600395.
34. Procter J, Hopkins FE, Fileman ES, Lindeque PK. Smells good enough to eat: Dimethyl sulfide (DMS) enhances copepod ingestion of microplastics. Mar Pollut Bull 2019;138:1-6.
35. Turner JT. The importance of small planktonic copepods and their roles in pelagic marine food webs. Zool Stud 2004;43:255-66. Available from:
36. Støttrup JG. The elusive copepods: their production and suitability in marine aquaculture: copepods in marine aquaculture J G Støttrup. Aquac Res 2000;31:703-11.
37. Ajiboye OO, Yakubu AF, Adams TE, Olaji ED, Nwogu NA. A review of the use of copepods in marine fish larviculture. Rev Fish Biol Fisheries 2011;21:225-46.
38. Cole M, Lindeque P, Fileman E, et al. Microplastic ingestion by zooplankton. Environ Sci Technol 2013;47:6646-55.
39. Cole M, Lindeque P, Fileman E, Halsband C, Galloway TS. The impact of polystyrene microplastics on feeding, function and fecundity in the marine copepod Calanus helgolandicus. Environ Sci Technol 2015;49:1130-7.
40. Cole M, Coppock R, Lindeque PK, et al. Effects of nylon microplastic on feeding, lipid accumulation, and moulting in a coldwater copepod. Environ Sci Technol 2019;53:7075-82.
41. Avery-Gomm S, O’Hara PD, Kleine L, Bowes V, Wilson LK, Barry KL. Northern fulmars as biological monitors of trends of plastic pollution in the eastern North Pacific. Mar Pollut Bull 2012;64:1776-81.
42. Costa E, Piazza V, Lavorano S, Faimali M, Garaventa F, Gambardella C. Trophic transfer of microplastics from copepods to jellyfish in the marine environment. Front Environ Sci 2020;8:571732.
43. Setälä O, Fleming-Lehtinen V, Lehtiniemi M. Ingestion and transfer of microplastics in the planktonic food web. Environ Pollut 2014;185:77-83.
44. Hansson L, Gyllström M, Ståhl-delbanco A, Svensson M. Responses to fish predation and nutrients by plankton at different levels of taxonomic resolution. Freshw Biol 2004;49:1538-50.
45. Rasdi NW, Qin JG. Improvement of copepod nutritional quality as live food for aquaculture: a review. Aquac Res 2016;47:1-20.
46. Manning CG, Foster SJ, Vincent ACJ. A review of the diets and feeding behaviours of a family of biologically diverse marine fishes (Family Syngnathidae). Rev Fish Biol Fisheries 2019;29:197-221.
47. Smith M, Love DC, Rochman CM, Neff RA. Microplastics in seafood and the implications for human health. Curr Environ Health Rep 2018;5:375-86.
48. Neves D, Sobral P, Ferreira JL, Pereira T. Ingestion of microplastics by commercial fish off the Portuguese coast. Mar Pollut Bull 2015;101:119-26.
49. Cox KD, Covernton GA, Davies HL, Dower JF, Juanes F, Dudas SE. Human consumption of microplastics. Environ Sci Technol 2019;53:7068-74.
50. Pironti C, Ricciardi M, Motta O, Miele Y, Proto A, Montano L. Microplastics in the environment: intake through the food web, human exposure and toxicological effects. Toxics 2021;9:224.
51. Yee MS, Hii LW, Looi CK, et al. Impact of microplastics and nanoplastics on human health. Nanomaterials 2021;11:496.
52. Hamid F, Bhatti MS, Anuar N, Anuar N, Mohan P, Periathamby A. Worldwide distribution and abundance of microplastic: how dire is the situation? Waste Manag Res 2018;36:873-97.
53. Cospheric. Technical information sheet: suspension of hydrophobic particles in aqueous solution. 2017. Available from: https://www.cospheric.com/images/PDFs/Suspension-of-Hydrophobic-Particles-in-Aqueous-Solution-1.pdf. [Last accessed on 23 May 2024].
54. Rinawati M, Sari LA, Pursetyo KT. Chlorophyll and carotenoids analysis spectrophotometer using method on microalgae. IOP Conf Ser Earth Environ Sci 2020;441:012056.
55. Lichtenthaler HK, Buschmann C. Chlorophylls and carotenoids: measurement and characterization by UV-VIS spectroscopy. Curr Protoc Food Anal Chem 2001;1:F4.3.1-8.
58. Tave D, Toya LA, Hutson AM. Behavioral observations of the endangered Rio Grande silvery minnow in a conservation aquaculture facility. Croat J Fish 2018;76:7-26.
59. Pich JM, Belden A, Carlson BE. Individual variation in boldness in turtles is consistent across assay conditions and behavioural measures. Behav 2019;156:1039-56.
60. Hammond TT, Jacobs LE, Curtis MJ, Trotman EM, Swaisgood RR, Shier DM. Early life experience with predators impacts development, behavior, and post-translocation outcomes in an endangered amphibian. Anim Conserv 2023;27:23-36.
62. Ross RM, Krise WF, Redell LA, Bennett RM. Effects of dissolved carbon dioxide on the physiology and behavior of fish in artificial streams. Environ Toxicol 2001;16:84-95.
63. Tosetto L, Williamson JE, Brown C. Trophic transfer of microplastics does not affect fish personality. Anim Behav 2017;123:159-67.
64. Rozman U, Turk T, Skalar T, et al. An extensive characterization of various environmentally relevant microplastics - material properties, leaching and ecotoxicity testing. Sci Total Environ 2021;773:145576.
65. Zhao S, Zhu L, Li D. Microscopic anthropogenic litter in terrestrial birds from Shanghai, China: not only plastics but also natural fibers. Sci Total Environ 2016;550:1110-5.
66. Zhu C, Li D, Sun Y, et al. Plastic debris in marine birds from an island located in the South China Sea. Mar Pollut Bull 2019;149:110566.
67. Sheehan KL, Patel P, Mertz Z. Parasites and plastics in and on stranded northern gannets, Morus bassanus. Matters 2021;7:e202105000003.
68. Li B, Liang W, Liu QX, et al. Fish ingest microplastics unintentionally. Environ Sci Technol 2021;55:10471-9.
69. Yin X, Wu J, Liu Y, et al. Accumulation of microplastics in fish guts and gills from a large natural lake: selective or non-selective? Environ Pollut 2022;309:119785.
70. Liang W, Li B, Jong MC, et al. Process-oriented impacts of microplastic fibers on behavior and histology of fish. J Hazard Mater 2023;448:130856.
71. Saidon NB, Szabó R, Budai P, Lehel J. Trophic transfer and biomagnification potential of environmental contaminants (heavy metals) in aquatic ecosystems. Environ Pollut 2024;340:122815.
72. Bashir I, Lone FA, Bhat RA, Mir SA, Dar ZA, Dar SA. Concerns and threats of contamination on aquatic ecosystems. In: Hakeem K, Bhat R, Qadri H, editors. Bioremediation and biotechnology. Cham: Springer; 2020. pp. 1-26.
73. Naidu R, Biswas B, Willett IR, et al. Chemical pollution: a growing peril and potential catastrophic risk to humanity. Environ Int 2021;156:106616.
74. Williams RS, Brownlow A, Baillie A, et al. Spatiotemporal trends spanning three decades show toxic levels of chemical contaminants in marine mammals. Environ Sci Technol 2023;57:20736-49.
75. Huang W, Song B, Liang J, et al. Microplastics and associated contaminants in the aquatic environment: A review on their ecotoxicological effects, trophic transfer, and potential impacts to human health. J Hazard Mater 2021;405:124187.
76. Thompson RC, Swan SH, Moore CJ, vom Saal FS. Our plastic age. Philos Trans R Soc Lond B Biol Sci 2009;364:1973-6.
Cite This Article
Export citation file: BibTeX | EndNote | RIS
OAE Style
McHale ME, Sheehan KL. Bioaccumulation, transfer, and impacts of microplastics in aquatic food chains. J Environ Expo Assess 2024;3:15. http://dx.doi.org/10.20517/jeea.2023.49
AMA Style
McHale ME, Sheehan KL. Bioaccumulation, transfer, and impacts of microplastics in aquatic food chains. Journal of Environmental Exposure Assessment. 2024; 3(3): 15. http://dx.doi.org/10.20517/jeea.2023.49
Chicago/Turabian Style
Marykate E. McHale, Kate L. Sheehan. 2024. "Bioaccumulation, transfer, and impacts of microplastics in aquatic food chains" Journal of Environmental Exposure Assessment. 3, no.3: 15. http://dx.doi.org/10.20517/jeea.2023.49
ACS Style
McHale, ME.; Sheehan KL. Bioaccumulation, transfer, and impacts of microplastics in aquatic food chains. J. Environ. Expo. Assess. 2024, 3, 15. http://dx.doi.org/10.20517/jeea.2023.49
About This Article
Special Issue
Copyright
Data & Comments
Data
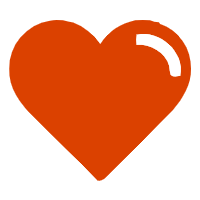
Comments
Comments must be written in English. Spam, offensive content, impersonation, and private information will not be permitted. If any comment is reported and identified as inappropriate content by OAE staff, the comment will be removed without notice. If you have any queries or need any help, please contact us at support@oaepublish.com.