Bioaccumulation and biotransformation of plasticisers diisononyl phthalate and di(2-ethylhexyl) terephthalate in black soldier fly larvae reared on (micro)plastic-contaminated food waste
Abstract
Food waste is currently used for the production of biogas. However, a reusage of waste is preferred to follow the principles of the circular economy and consider the waste management hierarchy, which can be achieved by rearing black soldier fly (BSF) larvae on such organic waste. Nonetheless, the presence of (micro)plastics and related additive plasticisers might induce chemical safety hazards to the larval applications as feed. Therefore, the bioaccumulation and biotransformation of two plasticisers (diisononyl phthalate (DINP) and di(2-ethylhexyl) terephthalate (DEHT)) in BSF larvae reared on food waste streams contaminated with polyvinyl chloride (PVC) (micro)plastics were investigated. Results showed that BSF larvae appeared to have a moderate intake of DINP during its rearing phase of 10 days (82 - 273 ng/g), while being able to biotransform it into the primary biotransformation product monoisononyl phthalate (MINP) within 24 h. For DEHT, an uptake of 67 - 137 ng/g was measured in the BSF larvae; however, no clear biotransformation pattern was observed. In addition, while no secondary oxidative biotransformation products were found in the larvae, these were measured in the frass, leading to the hypothesis that microorganism-mediated biotransformation of plasticisers occurred. In conclusion, based on the results of this study, BSF larvae could potentially be used safely in the frame of circular economy, when reared on organic substrates contaminated with the same PVC microplastic content and sizes used.
Keywords
INTRODUCTION
The global population recently surpassed the 8 billion mark and is expected to rise to 10 billion people by 2050[1]. Following the increasing world population, the food demand will also rise[2], including a corresponding surge in meat production. This meat production is projected to almost double, from 275 million tonnes in 2007 to 455 million tonnes by 2050[2,3]. As a result, there is currently an increased demand for feed protein sources, such as soybeans and fishmeal, which in turn leads to more deforestation, intensification of land and water use, and overfishing[2,4,5]. Therefore, an alternative, more sustainable feed source is strongly required.
A viable option to address such increasing feed demand without causing a severe environmental impact is currently represented by using insects[6]. Certain species of insects are, in fact, increasingly praised as promising alternative protein sources for human food and animal feed[6,7], with the black soldier fly (BSF, Hermetia illucens L.) being considered one of the most suitable insect species for feed production. This is mostly due to its relatively short holometabolous life cycle (approx. 40-45 days, depending on the substrate used)[8-10], and the suitable nutritional profile of their larvae when used as feedstock[11]. Additionally, BSF larvae are also considered to be beneficial insects, as they can be reared on a wide plethora of organic substrates, from classic animal feed (e.g., chicken feed) to waste streams (e.g., food waste, manure, etc.)[12,13]. However, rearing BSF larvae on such waste streams can lead to potential chemical, physical, and microbiological hazards[9,14,15].
A particularly environmentally relevant type of chemical hazard derives from the potential presence of (micro)plastics in the substrate, and their related additive plasticisers (including legacy phthalates and alternative plasticisers). Such contaminants are typically present in residual waste streams deriving from, e.g., the food industry, supermarkets, and catering services[16]. Currently, these residual food waste streams are used to produce biogas, while the remaining residue is used in agriculture as organic fertiliser, possibly introducing more (micro)plastics into the environment[16-18]. According to the waste hierarchy management and the principles of circular economy, using such food waste streams as a substrate to rear insects for feed, rather than for energy generation, would be both more profitable and environmentally sustainable[19,20]. However, the potential presence and effects of (micro)plastics and related additive compounds on the used insects as feed for livestock animals require thorough investigations.
Polyvinyl chloride (PVC) is used in non-food and food-related applications, including food packaging, such as bottles and foils[21]. This is due to its ease of thermoforming and its flexibility originating from the addition of additives, such as plasticisers, which can go up to 60% of its mass[21,22] and might thus be present in the organic rearing substrate. Previous studies showed that related additive plasticisers (e.g., diisononyl phthalate, DINP, and di(2-ethylhexyl) terephthalate, DEHT) can potentially induce adverse health effects as these substances can be carcinogenic, endocrine disruptors, and/or exhibit anti-androgenic effects in humans and wildlife[23-26]. Although recent studies documented that BSF larvae are not affected by the ingestion of microplastics in terms of growth performance, survival rate, and bioconversion, even when ingestion was proven[27-30], their chemical safety when reared on such contaminated substrates has, to the best of our knowledge, not been explored yet. As a result, the potential impact of additive plasticisers on the chemical safety of BSF larvae is still unknown, impeding the full exploitation of the BSF larvae.
To fill this gap, we report in this study a general screening of the additive composition in different plastic food contact materials in order to select a model plastic (i.e., PVC). Following the screening, BSF larvae were reared on artificially prepared food waste containing different sizes of PVC plastics,
MATERIALS AND METHODS
Chemicals, reagents, and materials
A list of chemicals and materials used in this study is reported in Supplementary Table 1 of the Supplementary Section 1.
Identification of additive compounds in plastic food contact materials
A variety of plastics commonly used as food contact materials [i.e., expanded polystyrene (EPS, n = 3), high-density polyethylene (HDPE, n = 1), low-density polyethylene (LDPE, n = 4), polylactic acid (PLA, n = 5), and polyvinyl chloride (PVC, n = 3)] were purchased from local retailers (i.e., Colruyt Group, Delhaize, Carrefour, and AVA) and B2B companies (i.e., Flexfilm, Euralpack, and Sidaplax) in 2019. To select the most suitable plastic material to perform exposure experiments with BSF larvae, the presence and concentrations of additive compounds (including legacy phthalates - LPs, alternative plasticizers - APs, and organophosphorus flame retardants - PFRs) were investigated via a suspect screening analysis (SSA) followed by a targeted analysis, as detailed described in Supplementary Section 2.
Extraction procedure
The plastic materials used in this study were cut into pieces of approximately 1 cm² using pre-cleaned stainless-steel scissors. An aliquot of 100 mg was extracted in duplicate using 5 mL
Instrumental analysis
For the suspect screening, the samples were analysed using an Agilent 1290 Infinity ultra-high performance liquid chromatography coupled to an Agilent 6530 quadrupole time-of-flight high-resolution mass spectrometer (QToF-MS) equipped with an electrospray ionisation source (ESI) and a Grace Alltima HP C18-EPS column (100 mm 2.1 mm; 3 µm particle size), according to Christia et al.[34] as documented in detail in Supplementary Section 2.2.
Rearing black soldier fly larvae
BSF larvae were obtained from the rearing colony of Centre of Expertise: Sustainable Biomass and Chemistry (Thomas More University of Applied Sciences, Belgium), hatched, and reared for the first eight days, according to Broeckx et al.[35]. Eight days after hatching, approximately 15,000 larvae were transferred to their rearing container (HDPE polymer, not containing the compounds of interest, L = 60 cm, W = 40 cm, H = 20 cm, Engels Logistics NV) containing 10 kg artificial food waste substrate prepared according to Lievens et al.[16]. To this substrate (except for the control), different sizes (macroplastics: 5 cm × 5 cm, mesoplastics: 5 mm × 5 mm, and microplastics: Dv(50) = 279 µm, being the median size) and contents (w = 5.0% for macroplastics and w = 1.0% for meso- and microplastics) of PVC plastics (Flexfilm, selected based on the results and discussion section “Selection of the plastic material and associated additive compounds”) were separately added as performed in a previous study according to Lievens et al.[28]. The used macro- and mesoplastics were cut in the appropriate size using pre-cleaned stainless-steel scissors, while the microplastics were produced using a Spex Freezer/Mill according to Lievens et al.[36]. The rearing experiments lasted for 10 days, starting 8 days after hatching and ending at 17 days after hatching. Afterwards, the larvae were individually collected, washed, and transferred to a clean rearing container (without substrate) to starve them for 2 days. During both the rearing and starvation phases, the larvae were kept at their optimal environmental conditions, being 27 °C with a relative humidity of 60%.
Sampling procedure
During the rearing and starvation phases (i.e., 10 and 2 days long, respectively), approximately 3 g larvae and substrate/residue were collected daily from each rearing container and stored in the freezer (-18 °C) pending further processing. Since BSF larvae live in their substrate, the substrate is continuously mixed with their frass (i.e., the residue). Therefore, it is not possible to distinguish between the two. The substrate/residue samples were lyophilised using a Büchi Lyovapor L-200 for 24 h under 0.200 mbar vacuum and
Quantification of DINP and DEHTP and their biotransformation products in black soldier fly larvae and respective substrate/residue mixtures
Extraction procedure
The quantification of selected plasticisers (i.e., DINP and DEHT, based on the results and discussion section “Selection of the plastic material and associated additive compounds”) and corresponding biotransformation products (MINP, cx-MINP, OH-MINP, MEHTP, cx-MEHTP, and 5-OH-MEHTP) in the exposed BSF larvae and related substrates, was based on the protocols described by Christia et al.,
Gas chromatographic analysis of parent compounds
The parent compounds were analysed following the procedure published by Christia et al.[37] after slight adjustments. More details are reported in Supplementary Section 3.2 and Supplementary Table 2.
Liquid chromatographic analysis of biotransformation products
To quantify the biotransformation products, a modified method using an Agilent 1200 Infinity liquid chromatography coupled to an Agilent 6460 Triple Quadrupole mass spectrometer was applied, according to Bastiaensen et al.[39]. More information is provided in Supplementary Section 3.3 and Supplementary Table 2.
In-house method validation
The in-house validation was performed as described by Christia et al.[37] according to the EMA guidelines, as discussed in Supplementary Section 3.4. The accuracy, recovery, and precision within and between experiments can be found in Supplementary Tables 3 and 4.
Quality control and quality assurance
During sample preparation, two procedural blanks and two QC samples (containing both parent compounds and biotransformation products at 250 ng and 30 ng, respectively) were analysed with each batch of samples (n = 20) to monitor potential background contamination, stability of the instrumental system, and accuracy during sample preparation. If targeted compounds were present in the procedural blanks, their value was subtracted from the actual samples. To determine the limit of quantification (LOQ) of the parent compounds, the standard deviation (SD) of the compound detected in the blank was multiplied by 3 (LOQ = 3 × SD), while for the biotransformation products, the lowest calibration concentration exceeding the mean + 2 × SD was applied. The recoveries of IS, accuracy, and repeatability of the QC samples and the method LOQs for the targeted compounds are reported in Supplementary Table 5. During the instrumental analysis, a quality control (QC) sample containing a mixture of targeted compounds (DINP: 860 ng and DEHT: 100 ng) was injected (after every 20 injections) into the GC-EI, to assess the instrument’s accuracy and precision during the run.
Statistical analysis
The concentrations of targeted compounds were reported in ng/g ww (wet weight). Values below LOQ were replaced by multiplying the detection frequency with the LOQ of the analyte (DF% LOQ). Statistical differences were tested with JMP Pro 16.0.0 (SAS) using a 5% significance level. A one-way ANOVA with a Tukey HSD post hoc test in case of equal variances was performed. If the variances were not equal (determined by an O’Brien test), the Welch’s ANOVA was executed. Lastly, a non-parametric Van der Waerden test with Steel-Dwass All Pairs as post hoc test was performed if the data were not normally distributed. The latter was evaluated by the Shapiro-Wilk test.
RESULTS AND DISCUSSION
Selection of the plastic material and associated additive compounds
A suspect screening workflow was performed to select an appropriate plastic material for the exposure study. In the 16 analysed plastic samples [EPS (n = 3), HDPE (n = 1), LDPE (n = 4), PLA, (n = 5),
For several compounds that matched between the suspect list and the extracted features, reference standards were available. This resulted in 31 prioritised compounds (6 phthalates, 10 alternative plasticisers, and 15 PFRs; Supplementary Table 6) which were, subsequently, analysed using a targeted approach to confirm the assigned compounds and to obtain quantitative data to create more evidence in the selection of the plastic material for the following exposure experiment. Therefore, the same samples (n = 16, extracted and injected in duplicate) were quantitatively analysed for 37 compounds including phthalates (n = 9), alternative plasticisers (n = 11), and PFRs (n = 17) [Figure 1].
Figure 1. Plasticiser and PFR concentrations in plastic food contact materials, extracted and measured in duplicate (n = 2). (A) Concentrations of all targeted compounds (ng/g logarithmic scale) and (B) concentrations of selected plasticisers in the PVC samples (mg/g).
The quantitative analysis revealed a low detection frequency and concentration of PFRs in most samples, except for the PVC materials [Figure 1A]. Nevertheless, in these PVC materials, the overall average concentration of PFRs was relatively low (240 ng/g) compared to the values observed for plasticizers. For the latter group of compounds, the concentrations were notably higher, particularly in the PVC samples, reaching an average plasticiser concentration of 4.4 mg/g. In comparison to PVC, the other plastic types showed an average plasticiser concentration of 845 ng/g. The observed high concentration (> 1 mg/g) of plasticisers in the PVC materials was mainly attributed to acetyl tributyl citrate (ATBC), di(2-ethylhexyl) adipate (DEHA), di(2-ethylhexyl) terephthalate (DEHT), and diisononyl phthalate (DINP) [Figure 1B].
The outcome of these analyses, which identified PVC as the plastic food contact material having the highest concentrations of additives, was confirmed in the literature. PVC plastics are reported as heavily plasticised, particularly with phthalates or adipates[22,42,43]. Furthermore, as shown in Figure 1B, each selected PVC material was characterised by the presence of two main plasticisers, of which at least one exhibited a concentration exceeding 40 mg/g (or w = 4%). As a result, the overall additive content was assumed to be between 15% - 60%, which is in line with the typical PVC formulation[22].
Two of the three investigated PVC materials (PVC-1 and PVC-2) contained DEHT as their primary additive plasticiser. This alternative plasticiser is not found to be toxic or mutagenic. Moreover, it is classified as category IV in the hazard classification system, indicating that it is currently considered chemically safe[42,44-47]. Consequently, this alternative plasticiser is considered the main replacement for the well-known phthalate plasticiser DEHP, generally known for its toxic behaviour, like being carcinogenic and endocrine disruptor[26,48]. Further, DINP was found to be the primary plasticiser in sample PVC-3, which is a commonly used phthalate compound employed for plasticising PVC materials[42,43,49]. Hence, owing to the presence of the phthalate moiety, DINP is more prone to induce adverse health effects compared to DEHT, as it is recognised for its toxicity being carcinogenic, an endocrine disruptor, and anti-androgenic[23,26,50]. To simultaneously investigate the fate of representative phthalates and alternative plasticisers in the BSF larvae reared on a plastic-contaminated substrate, the sample PVC-3, characterised by relatively high concentrations of both DINP (76.3 mg/g) and DEHT (12.5 mg/g), was selected as the most suitable plastic food contact material for the following exposure experiment.
Ingestion and bioaccumulation of DINP and DEHT in black soldier fly larvae
Following the screening of the plastic materials, BSF larvae were reared on an artificial food waste substrate, each spiked with one of three different sizes (micro-, meso-, and macroplastics) and different contents
In a recent study by Lievens et al., it was observed that BSF larvae can only ingest plastic particles smaller than their mouth opening size. Consequently, the plastic particles need to be smaller than approximately 110 µm to be ingested[29]. Since the plastics used in this experiment were generally larger than 110 µm, the results of the exposure study indicate that the BSF larvae were unable to ingest the added (micro)plastics. This is confirmed by the fact that the smallest fraction (i.e., microplastics) in this study exhibited Dv(10), Dv(50), and Dv(90) values of 111, 279, and 703 μm, respectively, meaning that 10, 50, and 90% of the particles are smaller than the respective given size. The uptake of the additive plasticisers could thus solely happen through the migration of the additive plasticisers from the plastics to the substrate/residue mixtures and the ingestion of the substrate by the larvae. Therefore, the migration of the additive plasticisers from the PVC plastics to the substrate was investigated [Figure 2].
Figure 2. Boxplot of DEHT (blue) and DINP (red) in all substrate/residue mixtures (ng/g wet weight) during the rearing experiments of 10 days. The data is based on all samples generated within one treatment (n = 30 per treatment). The control substrate/residue mixture did not contain plastics, while the other substrate/residue mixtures contained macroplastics (w = 5%), or meso- or microplastics (w = 1%). The horizontal white lines represent the median concentration, while statistical differences (P < 0.05) are depicted with an asterisk (*) for DEHT regarding the macroplastics and with two asterisks (**) for DEHT concerning the mesoplastics. The boxes represent the lower and upper quartiles. The whiskers represent the maximum values, while the dots depict the outliers of the dataset.
Based on the obtained results, no significant differences (P = 0.647) were found regarding the median DINP concentrations for any of the substrate/residue mixtures [control (a substrate not containing PVC plastics): 59 ng/g, macroplastics: < LOQ, mesoplastics: 54 ng/g and microplastics: < LOQ]. It can be hypothesised that no significant migration of DINP from the PVC plastics into the substrate occurred, likely due to the low fat content of the substrate (approximately 1%, based on wet weight). This can be confirmed by the high octanol-water partition coefficient of DINP (Log KOW = 9.37), indicating that the compound is highly hydrophobic. Consequently, DINP is expected to be more prevalent in nonpolar (fat-rich) rather than aqueous environments like the substrate used in this study, which had a water content of approximately 75%[51-53].
In contrast to DINP, a significant difference (P < 0.0001) in DEHT concentrations was observed across the different exposure scenarios [Figure 2]. The median concentration of DEHT in the substrate/residue mixture containing macro plastics (54 ng/g) was significantly higher compared to the control (< LOQ) and microplastic (< LOQ) exposure scenarios. Additionally, the median concentration of DEHT in the substrate/residue mixture containing mesoplastics (33 ng/g) was also significantly higher than in the substrates containing microplastics. Given the particle size and the corresponding surface volume ratio, a more pronounced leaching behaviour could be presumed for the microplastics. However, this trend was not noted in the data, partly due to an unforeseen external contamination, which introduced challenges in the data interpretation.
Additionally, based on the lower octanol-water partition coefficient of DEHT (Log KOW= 8.39) compared to DINP, a more pronounced migration of DEHT into a water-rich matrix can be expected[54]. However, the higher potential migration of DEHT is less relevant due to the lower concentrations of DEHT compared to DINP in the PVC plastic. As a result, the overall concentration of DEHT in the substrate remained lower than DINP. Moreover, the migration of these compounds can be considered limited, since the concentrations of both DINP and DEHT found in the substrates were lower than estimated based on the concentration of plasticisers present in the plastic materials and the amount of plastics added to the substrates (i.e., 3.81 mg/g for DINP and 0.62 mg/g for DEHT).
Despite these observations, the concentrations of the same compounds were also determined in the BSF larvae exposed to the different fractions of PVC plastic particles [Figure 3]. The obtained results indicated that the median concentration of DEHT in the BSF larvae reared on a substrate containing mesoplastics
Figure 3. Boxplot of DEHT (blue) and DINP (red) in all BSF larvae (ng/g wet weight) during the rearing experiments of 10 days. The data are based on all larval samples generated (n = 30 per treatment). The control BSF larvae were not exposed to plastics, while the other BSF larvae were exposed to macroplastics (w = 5%), or meso- or microplastics (w = 1%). The horizontal white lines represent the median concentration, while statistical differences (P < 0.05) are depicted with an asterisk (*) for DINP and two asterisks (**) for DEHT. The boxes represent the lower and upper quartiles. The whiskers represent the maximum values, while the dots depict the outliers of the dataset.
BSF larvae reared on a substrate containing mesoplastics exhibited a significantly higher (P = 0.035) median concentration of DINP (284 ng/g) compared to the control larval group (< LOQ). Larvae exposed to macro- and microplastics had DINP concentrations that were respectively < LOQ and 98 ng/g, which were not statistically significant (P > 0.121). As a result, it appears that BSF larvae have the potential to take up additive plasticisers derived from PVC (micro)plastics present in their substrate. However, the uptake was rather limited, caused by the limited migration of the compounds from the plastic materials present in the substrate. Furthermore, it should be noted that based on the results obtained, safety conclusions are difficult to draw, since the current EU feed legislation does not contain maximum limits for these compounds. The only limits present in the EU legislation are migration limits of DINP from packaging materials into food
To the best of our knowledge, no previous studies exposed BSF larvae to DINP and/or DEHT, which limits the ability to make comparisons to literature. Nonetheless, the mean concentration ranges of DINP
In addition, the BSF larvae in this study were exposed to PVC containing DINP and DEHT and not directly to DINP and/or DEHT itself. In contrast, Zhang et al. conducted a study in which fruit flies (Drosophila melanogaster) were directly exposed to DINP. They assessed the impact of DINP on several parameters (e.g., hatching rate, survival, metamorphosis, etc.) of D. melanogaster, and observed that they were negatively affected, showing a reduced growth and a higher mortality rate at exposure concentrations from 0.2% to 0.5%[57,58]. These results were different from the results obtained for the BSF larvae, where no difference in growth or survival was observed between the larvae exposed to PVC containing DINP and the control[28]. However, it has to be noted that in the current study, exposure to DINP and DEHT resulted from migration from PVC plastics. This resulted in a calculated exposure level of 0.076% - 0.38% for DINP, and 0.012% - 0.062% for DEHT, based on the additive content present in the PVC plastics combined with the amount of plastics added to the related substrates. Although the calculated exposure level of DINP was similar, the approach applied in this study led to much lower effective exposure levels (< 0.42 × 10-4%), as the additive compounds are not directly (bio)available to the larvae through the substrate, making it more difficult to induce any effects. These low actual concentrations present for the larvae also explain the lack of a clear difference in accumulation between the different exposure scenarios, confirming that the larvae were not able to ingest the added microplastics.
After measuring the DINP and DEHT concentrations in the BSF larvae and in substrate/residue mixtures, the bioaccumulation factor or BAF (the ratio between the concentrations of DINP and DEHT in the larvae and in their substrate) was determined. The BAFs of both compounds remained < 1 (i.e., < 0.001), indicating that no significant (bio)accumulation occurred. This finding supports the hypothesis that if the compounds were taken up by the BSF larvae, they were biotransformed and/or eliminated shortly after ingestion. However, the mode of potential storage of these lipophilic compounds, such as in the fat body, exuviae, or gut, requires further investigation. The latter could be of crucial importance since BSF larvae seem to be able to excrete compounds such as metals (e.g., cadmium and chromium) by moulting[59,60], but this is not yet known for plasticisers or other organic compounds. Based on the limited accumulation and low concentrations observed in the larvae, it can be cautiously assumed that the chemical safety of BSF larvae was not compromised for use as a feed compound when reared on substrates contaminated with the sizes and fractions of PVC (micro)plastics used in this study. This may be because the plastic particles were too large to be ingested.
Biotransformation and excretion of DINP and DEHTP by black soldier fly larvae
To further elucidate the potential biotransformation and excretion capabilities of DINP and DEHT in BSF larvae, daily sub-samples of BSF larvae and substrate/residue mixtures were analysed for primary (MINP for DINP and MEHTP for DEHT) and secondary (OH-MINP, cx-MINP for DINP and OH-MEHTP, cx-MEHTP for DEHT) biotransformation products. The measured concentrations of DINP and related primary and secondary biotransformation products over the rearing time in all exposure scenarios are shown in Figures 4A-D (substrate/residue mixtures) and Figures 4E-H (BSF larvae).
Figure 4. Variation in the concentrations (ng/g ww) of DINP, primary biotransformation product (MINP), and secondary biotransformation products (OH-MINP and cx-MINP, dashed lines) over time in the substrate/residue mixtures and BSF larvae. Figure A, B, C, and D depict the contents of these compounds in the control and substrate containing macro-, meso-, and microplastics. Figure E, F, G, and H depict the contents of the compounds in BSF larvae reared on the control and substrates containing macro-, meso-, and microplastics, respectively. Vertical dotted lines represent the start of the starvation. The concentration of DINP can be found on the left y-axis, while the concentration of the biotransformation products can be found on the right y-axis. The data (n = 3) are based on wet weight, while no standard deviations are shown to avoid ambiguities.
No clear temporal pattern across the various sizes of PVC plastics emerged for either substrates or larvae. This suggests that the plastic particle sizes did not exert a considerable effect on the uptake and/or biotransformation of DINP present in the used PVC material. On the other hand, the observed uptake of the parent compounds (DINP and DEHT) was limited, as discussed in the previous section, challenging the occurrence of biotransformation products at sufficient concentrations.
For all substrate/residue mixtures [Figure 4A-D], the initial concentration of DINP exhibited a decreasing trend during the first two days of the experiments. In contrast, the concentrations of DINP slightly increased in the BSF larvae [Figure 4E-H]. Additionally, the larvae-initiated biotransformation converts DINP into its primary biotransformation product (MINP), which is shown to occur rapidly in the gastrointestinal tract of humans and rodents[49,61,62]. It is, therefore, assumed that similar hydrolysis also took place in the gut of the BSF larvae, as they started to excrete MINP into the substrate. This excretion happened swiftly, due to a transit time through their gut of approximately 3 h[63], which also resulted in limited time for accumulation in BSF larval biomass.
At 10 and 12 days after hatching (DAH), an unforeseen external contamination with DINP occurred in the macroplastic, and control and mesoplastic exposure scenarios, respectively. This contamination could have been caused by plasticisers present in indoor dust that may have accidentally entered the rearing containers[64,65], or during the handling of the samples during/after harvesting. Nonetheless, this external contamination resulted in an elevated DINP concentration in the substrate, facilitating the uptake of DINP by the larvae and subsequent hydrolysis into MINP. The evidence of this hydrolysis is visible through the increased level of MINP observed in the larvae one day after the contamination event (11 DAH, Figure 4F and 13 DAH, Figure 4E and G). These findings further support the hypothesis that the uptake of DINP is linked to its presence in the substrate, rather than originating from the plastic materials itself, due to the limited probability of the used plastic being ingested by the BSF larvae.
Despite the fact that DINP was hydrolysed into MINP, the secondary oxidative biotransformation products (i.e., OH-MINP and cx-MINP), enzymatically mediated by oxidative reactions of, for instance, Cytochrome P450 enzymes, were generally not detected in the larvae[62,66]. A previous study has, however, demonstrated that BSF larvae possess the capability to metabolise mycotoxins, such as aflatoxin B1, into aflatoxin P1 through the involvement of Cytochrome P450 enzymes[67]. Hence, it can be cautiously assumed that the larvae have the inherent ability to convert DINP into secondary oxidative biotransformation products. However, the absence of these biotransformation products is likely due to low concentrations of the parent compounds together with the short transit time of the larvae, which possibly resulted in concentrations of secondary biotransformation products below the limit of quantification. Consequently, further research with exposure levels at higher concentrations of parent compounds is required to elucidate the extent to which BSF larvae are capable of biotransforming these compounds.
In addition, the concentration of the biotransformation products in the residue generally increased from the start of the starvation (i.e., 17 DAH, Figure 4A-D), across all substrate scenarios. Since the larvae were transferred to clean and empty rearing containers at that time point, only frass was present alongside the larvae. As such, there were no substrate residues, unlike those found in the rearing residue. Interestingly, secondary biotransformation products were detected in the frass during the starvation period, whereas their presence was generally not observed during the rearing phase. This is probably due to a high dilution factor induced by the large amount of present substrate. Hence, it is plausible to assume that the appearance of the secondary biotransformation products in the residue was facilitated by the presence of specific micro-organisms in the frass eventually originated from the BSF larvae.
It has been established that a consortium of saline soil bacteria, including Achromobacter sp., Pseudomonas sp., Brevundimonas sp., etc., among others, can degrade DINP into primary metabolites, such as MINP and MNP (methyl nonyl phthalate), via de-esterifications and -oxidations, respectively[68]. However, the targeted secondary biotransformation products were not detected. Further, based on our in-house database, the same bacterial genera have been sporadically identified in BSF larvae. Consequently, future research is highly recommended to explore the presence of bacterial strains capable of additive degradation in BSF larvae.
In contrast to DINP, the temporal patterns of DEHT did not display a distinct trend, while an unforeseen contamination event occurred likewise for DINP [Supplementary Figure 1]. The larvae exhibited limited uptake of the parent compound, likely attributed to the relatively low concentrations of DEHT present in the PVC materials used. Nonetheless, evidence of the hydrolysis of DEHT was observed, as indicated by the presence of the primary biotransformation product (MEHTP), as displayed in Supplementary Figure 1H
As this study represents the first investigation of DINP and DEHT in BSF larvae using (micro)plastics as exposure vectors, future exposure studies involving higher concentrations of the analytes in a more bioavailable form are required to ascertain the extent to which BSF larvae can perform such biotransformation processes.
Limitations of this study
Despite the valuable insights obtained in this study, several limitations were encountered during its execution and analysis. Firstly, a decision had to be made regarding whether to focus on the temporal monitoring of the targeted compounds or to examine the end of the rearing phase in detail. In this study, the former approach was applied, resulting in the generation of numerous samples (approximately 300). However, due to the limited number of replicates (i.e., three pooled samples at each time point), the data showed considerable biological variation. Moreover, while the use of plastic materials as the exposure vector for additive plasticisers represented a realistic scenario, it also introduced limited migration of the compounds to the substrate, causing the actual exposure concentration of the plastic additives to be lower than intended. Lastly, the particle size used in this experiment was apparently too large to be ingested by the BSF larvae[29]. By combining all these limitations, the interpretation of the results was challenging, making it difficult to draw firm conclusions.
CONCLUSIONS
This study showed for the first time the uptake dynamics of DINP and DEHT in BSF larvae reared on an organic substrate (food waste) contaminated with PVC macro-, meso-, and microplastics. Our results showed that BSF larvae possess the ability to take up these additive plasticisers, and to a certain extent, biotransform and excrete them, thereby limiting their accumulation in the larval biomass (BAF < 0.001). Furthermore, the presence of secondary DINP biotransformation products (OH-MINP and cx-MINP) in the frass suggests the potential involvement of micro-organisms capable of degrading/oxidising these plastics/additives. To elucidate the responsible micro-organisms and the specific pathways involved in this biotransformation, further research is required.
Although this study can be considered a preliminary investigation, it highlights the promising potential of rearing BSF larvae on plastic-contaminated food waste. Nonetheless, additional research is still necessary to assess the chemical safety of BSF larvae when other additives are present in the rearing substrate.
DECLARATIONS
Acknowledgments
We want to acknowledge Centre of Expertise: Sustainable Biomass and Chemistry and, more specifically, Ann Wuyts for providing us with black soldier fly larvae, and the support during the rearing phase.
Authors’ contributions
Conceptualised the study: Lievens S, Van Der Borght M, Covaci A, Poma G
Performed all experiments: Lievens S, Bombeke J, Belova L, Yin S, Fujii Y, Poma G
Wrote the original draft: Lievens S
Proofread and edited the text: Yin S, Belova L, Fujii Y, De Smet J, Van Der Borght M, Covaci A, Poma G
Availability of data and materials
The datasets generated during the current study are available from the corresponding author upon reasonable request.
Financial support and sponsorship
This research was funded via the FWO (Research Foundation Flanders) under grant agreements S008519N (ENTOBIOTA), 12V5222N; LB and SY acknowledge funding through FWO fellowships 11G1821N and 1270521N; respectively. Y.F. acknowledges JSPS overseas research fellowship with grant number 201860307; Lastly, GP was supported by the Exposome Centre of Excellence of the University of Antwerp (BOF grant, Antigoon database number 41222).
Conflicts of interest
All authors declared that there are no conflicts of interest. Giulia Poma is an Editorial Board Member of Journal of Environmental Exposure Assessment
Ethical approval and consent to participate
Not applicable.
Consent for publication
Not applicable.
Copyright
© The Author(s) 2024.
REFERENCES
1. United Nations. Population. Available from: https://www.un.org/en/global-issues/population#:~:text=Our%20growing%20population,and%202%20billion%20since%201998 [Last accessed on 24 Jan 2024].
2. Shumo M, Osuga IM, Khamis FM, et al. The nutritive value of black soldier fly larvae reared on common organic waste streams in Kenya. Sci Rep 2019;9:10110.
3. Halweil B. Meat production continues to rise. Available from: https://www.weltagrarbericht.de/fileadmin/files/weltagrarbericht/worldwatch_meat_2008.pdf [Last accessed on 24 Jan 2024].
4. Barona E, Ramankutty N, Hyman G, Coomes OT. The role of pasture and soybean in deforestation of the Brazilian Amazon. Environ Res Lett 2010;5:024002.
5. Dai P, Luan S, Sui J, et al. Insight into genetic potential for growth and survival of the Pacific white shrimp (Litopenaeus vannamei) in the context of low-protein and low-fishmeal diet use. Aquaculture Research 2022;53:3337-45.
6. van Huis A, Oonincx DGAB. The environmental sustainability of insects as food and feed. A review. Agron Sustain Dev 2017;37:43.
7. Shah AA, Totakul P, Matra M, Cherdthong A, Hanboonsong Y, Wanapat M. Nutritional composition of various insects and potential uses as alternative protein sources in animal diets. Anim Biosci 2022;35:317-31.
8. De Smet J, Wynants E, Cos P, Van Campenhout L. Microbial community dynamics during rearing of black soldier fly larvae (hermetia illucens) and impact on exploitation potential. Appl Environ Microbiol 2018;84:e02722-17.
9. Lievens S, Poma G, De Smet J, Van Campenhout L, Covaci A, Van Der Borght M. Chemical safety of black soldier fly larvae (hermetia illucens), knowledge gaps and recommendations for future research: a critical review. JIFF 2021;7:383-96.
10. Purschke B, Scheibelberger R, Axmann S, Adler A, Jäger H. Impact of substrate contamination with mycotoxins, heavy metals and pesticides on the growth performance and composition of black soldier fly larvae (hermetia illucens) for use in the feed and food value chain. Food Addit Contam Part A Chem Anal Control Expo Risk Assess 2017;34:1410-20.
11. Barragan-fonseca K, Dicke M, van Loon J. Nutritional value of the black soldier fly (hermetia illucens L.) and its suitability as animal feed - a review. JIFF 2017;3:105-20.
12. Miranda CD, Cammack JA, Tomberlin JK. Life-history traits of the black soldier fly, hermetia illucens (L.) (diptera: stratiomyidae), reared on three manure types. Animals 2019;9:281.
13. Spranghers T, Ottoboni M, Klootwijk C, et al. Nutritional composition of black soldier fly (hermetia illucens) prepupae reared on different organic waste substrates. J Sci Food Agric 2017;97:2594-600.
14. O'Connor J, Mickan BS, Siddique KHM, Rinklebe J, Kirkham MB, Bolan NS. Physical, chemical, and microbial contaminants in food waste management for soil application: A review. Environ Pollut 2022;300:118860.
15. Vandeweyer D, De Smet J, Van Looveren N, Van Campenhout L. Biological contaminants in insects as food and feed. JIFF 2021;7:807-22.
16. Lievens S, Slegers T, Mees MA, et al. A simple, rapid and accurate method for the sample preparation and quantification of meso- and microplastics in food and food waste streams. Environ Pollut 2022;307:119511.
17. Mercogliano R, Avio CG, Regoli F, Anastasio A, Colavita G, Santonicola S. Occurrence of microplastics in commercial seafood under the perspective of the human food chain. a review. J Agric Food Chem 2020;68:5296-301.
18. Oliveri Conti G, Ferrante M, Banni M, et al. Micro- and nano-plastics in edible fruit and vegetables. The first diet risks assessment for the general population. Environ Res 2020;187:109677.
19. United States Environmental Protection Agency. Sustainable materials management: non-hazardous materials and waste management hierarchy. Available from: https://www.epa.gov/smm/sustainable-materials-management-non-hazardous-materials-and-waste-management-hierarchy [Last accessed on 24 Jan 2024].
20. Directive 2008/122/EC of the European parliament and of the council. In: Radley-gardner O, Beale H, Zimmermann R, editors. Fundamental Texts On European Private Law. Hart Publishing; 2016.p. 593-605.
21. Marsh K, Bugusu B. Food packaging--roles, materials, and environmental issues. J Food Sci 2007;72:R39-55.
22. Navarro R, Pérez Perrino M, Gómez Tardajos M, Reinecke H. Phthalate plasticizers covalently bound to PVC: plasticization with suppressed migration. Macromolecules 2010;43:2377-81.
23. Chung BY, Choi SM, Roh TH, et al. Risk assessment of phthalates in pharmaceuticals. J Toxicol Environ Health A 2019;82:351-60.
24. Forner-Piquer I, Maradonna F, Gioacchini G, et al. Dose-specific effects of di-isononyl phthalate on the endocannabinoid system and on liver of female zebrafish. Endocrinology 2017;158:3462-76.
25. Kamrin MA. Phthalate risks, phthalate regulation, and public health: a review. J Toxicol Environ Health B Crit Rev 2009;12:157-74.
26. Qadeer A, Kirsten KL, Ajmal Z, Jiang X, Zhao X. Alternative plasticizers as emerging global environmental and health threat: another regrettable substitution? Environ Sci Technol 2022;56:1482-8.
27. Cho S, Kim C, Kim M, Chung H. Effects of microplastics and salinity on food waste processing by black soldier fly (hermetia illucens) larvae. J Ecology Environ 2020;44:7.
28. Lievens S, Poma G, Frooninckx L, et al. Mutual Influence between polyvinyl chloride (micro)plastics and black soldier fly larvae (hermetia illucens L.). Sustainability 2022;14:12109.
29. Lievens S, Vervoort E, Bruno D, et al. Ingestion and excretion dynamics of microplastics by black soldier fly larvae and correlation with mouth opening size. Sci Rep 2023;13:4341.
30. Romano N, Fischer H. Microplastics affected black soldier fly (hermetia illucens) pupation and short chain fatty acids. J Applied Entomology 2021;145:731-6.
31. Caldwell J, Taladriz-Blanco P, Lehner R, et al. The micro-, submicron-, and nanoplastic hunt: a review of detection methods for plastic particles. Chemosphere 2022;293:133514.
32. La Nasa J, Biale G, Fabbri D, Modugno F. A review on challenges and developments of analytical pyrolysis and other thermoanalytical techniques for the quali-quantitative determination of microplastics. J Anal Appl Pyrolysis 2020;149:104841.
33. Malarvannan G, Onghena M, Verstraete S, et al. Phthalate and alternative plasticizers in indwelling medical devices in pediatric intensive care units. J Hazard Mater 2019;363:64-72.
34. Christia C, Poma G, Caballero-Casero N, Covaci A. Suspect screening analysis in house dust from Belgium using high resolution mass spectrometry; prioritization list and newly identified chemicals. Chemosphere 2021;263:127817.
35. Broeckx L, Frooninckx L, Slegers L, et al. Growth of black soldier fly larvae reared on organic side-streams. Sustainability 2021;13:12953.
36. Lievens S, Vervoort E, Poma G, Covaci A, Van Der Borght M. A production and fractionation protocol for polyvinyl chloride microplastics. Methods Protoc 2023;6:15.
37. Christia C, Tang B, Yin SS, et al. Simultaneous determination of legacy and emerging organophosphorus flame retardants and plasticizers in indoor dust using liquid and gas chromatography-tandem mass spectrometry: method development, validation, and application. Anal Bioanal Chem 2019;411:7015-25.
38. Poma G, Yin S, Tang B, Fujii Y, Cuykx M, Covaci A. Occurrence of selected organic contaminants in edible insects and assessment of their chemical safety. Environ Health Perspect 2019;127:127009.
39. Bastiaensen M, Malarvannan G, Gys C, Ait Bamai Y, Araki A, Covaci A. Between- and within-individual variability of urinary phthalate and alternative plasticizer metabolites in spot, morning void and 24-h pooled urine samples. Environ Res 2020;191:110248.
40. European Medicines Agency. Guideline on bioanalytical method validation. Available from: https://www.ema.europa.eu/en/bioanalytical-method-validation-scientific-guideline [Last accessed on 24 Jan 2024].
41. Schymanski EL, Jeon J, Gulde R, et al. Identifying small molecules via high resolution mass spectrometry: communicating confidence. Environ Sci Technol 2014;48:2097-8.
42. Lakeev SN, Maydanova IO, Mullakhmetov RF, Davydova OV. Ester plasticizers for polyvinyl chloride. Russ J Appl Chem 2016;89:1-15.
43. Grosu E. Applications of polyvinylchloride (PVC)/thermoplastic nano-, micro- and macroblends. In: P. M. V, Darie-nita RN, editors. Polyvinylchloride-based Blends. Cham: Springer International Publishing; 2022. pp. 75-89.
44. Bui TT, Giovanoulis G, Cousins AP, Magnér J, Cousins IT, de Wit CA. Human exposure, hazard and risk of alternative plasticizers to phthalate esters. Sci Total Environ 2016;541:451-67.
45. Gadaleta D, Vuković K, Toma C, et al. SAR and QSAR modeling of a large collection of LD50 rat acute oral toxicity data. J Cheminform 2019;11:58.
46. OECD. Sids initial assessment profile. Available from: https://hpvchemicals.oecd.org/ui/handler.axd?id=62533f49-988b-48d3-865f-2af5abb24b33 [Last accessed on 24 Jan 2024].
47. Wirnitzer U, Rickenbacher U, Katerkamp A, Schachtrupp A. Systemic toxicity of di-2-ethylhexyl terephthalate (DEHT) in rodents following four weeks of intravenous exposure. Toxicol Lett 2011;205:8-14.
48. Crespo JE, Balart R, Sanchez L, López J. Substitution of di(2-ethylhexyl) phthalate by di(isononyl) cyclohexane-1,2-dicarboxylate as a plasticizer for industrial vinyl plastisol formulations. J Appl Polym Sci 2007;104:1215-20.
49. Hines CJ, Hopf NB, Deddens JA, Silva MJ, Calafat AM. Occupational exposure to diisononyl phthalate (DiNP) in polyvinyl chloride processing operations. Int Arch Occup Environ Health 2012;85:317-25.
50. Godoi FGA, Forner-Piquer I, Randazzo B, et al. Effects of Di-isononyl phthalate (DiNP) on follicular atresia in zebrafish ovary. Front Endocrinol 2021;12:677853.
51. Cumming H, Rücker C. Octanol-water partition coefficient measurement by a simple 1H NMR method. ACS Omega 2017;2:6244-9.
52. Henkel C, Hüffer T, Hofmann T. Polyvinyl chloride microplastics leach phthalates into the aquatic environment over decades. Environ Sci Technol 2022;56:14507-16.
53. National Center for Biotechnology Information. Diisononyl phthalate. Available from: https://pubchem.ncbi.nlm.nih.gov/compound/Diisononyl-phthalate [Last accessed on 24 Jan 2023].
54. National Center for Biotechnology. Bis(2-ethylhexyl) terephthalate. Available from: https://pubchem.ncbi.nlm.nih.gov/compound/Bis_2-ethylhexyl_-terephthalate [Last accessed on 24 Jan 2023].
55. European Commission. Commission Regulation (EU) No 10/2011 of 14 January 2011 on plastic materials and articles intended to come into contact with food text with EEA relevance. Available from: http://data.europa.eu/eli/reg/2011/10/oj [Last accessed on 24 Jan 2023].
56. Poma G, Fujii Y, Lievens S, et al. Occurrence, patterns, and sources of hazardous organic chemicals in edible insects and insect-based food from the Japanese market. Food Chem Toxicol 2021;154:112311.
57. Zhang Q, Hao LC, Hong Y. Exposure evaluation of diisononyl phthalate in the adults of Drosophila melanogaster: Potential risks in fertility, lifespan, behavior, and modes of action. Comp Biochem Physiol C Toxicol Pharmacol 2020;238:108847.
58. Zhang Q, Hao L, Hong Y. Detrimental effects induced by diisononyl phthalate on development and behavior of Drosophila larva and potential mechanisms. Comp Biochem Physiol C Toxicol Pharmacol 2021;243:108967.
59. Bulak P, Polakowski C, Nowak K, Waśko A, Wiącek D, Bieganowski A. Hermetia illucens as a new and promising species for use in entomoremediation. Sci Total Environ 2018;633:912-9.
60. Gao Q, Wang X, Wang W, Lei C, Zhu F. Influences of chromium and cadmium on the development of black soldier fly larvae. Environ Sci Pollut Res Int 2017;24:8637-44.
61. Saravanabhavan G, Murray J. Human biological monitoring of diisononyl phthalate and diisodecyl phthalate: a review. J Environ Public Health 2012;2012:810501.
62. Silva MJ, Reidy JA, Preau JL Jr, Needham LL, Calafat AM. Oxidative metabolites of diisononyl phthalate as biomarkers for human exposure assessment. Environ Health Perspect 2006;114:1158-61.
63. Gold M, Egger J, Scheidegger A, et al. Estimating black soldier fly larvae biowaste conversion performance by simulation of midgut digestion. Waste Manag 2020;112:40-51.
64. Kubwabo C, Rasmussen PE, Fan X, et al. Analysis of selected phthalates in Canadian indoor dust collected using household vacuum and standardized sampling techniques. Indoor Air 2013;23:506-14.
65. Luongo G, Östman C. Organophosphate and phthalate esters in settled dust from apartment buildings in Stockholm. Indoor Air 2016;26:414-25.
66. Stajnko A, Runkel AA, Kosjek T, et al. Assessment of susceptibility to phthalate and DINCH exposure through CYP and UGT single nucleotide polymorphisms. Environ Int 2022;159:107046.
67. Meijer N, Stoopen G, van der Fels-Klerx HJ, van Loon JJA, Carney J, Bosch G. Aflatoxin B1 conversion by black soldier fly (hermetia illucens) larval enzyme extracts. Toxins 2019;11:532.
Cite This Article
How to Cite
Lievens, S.; Yin S.; Belova L.; Fujii Y.; Bombeke J.; De Smet J.; Van Der Borght M.; Covaci A.; Poma G. Bioaccumulation and biotransformation of plasticisers diisononyl phthalate and di(2-ethylhexyl) terephthalate in black soldier fly larvae reared on (micro)plastic-contaminated food waste. J. Environ. Expo. Assess. 2024, 3, 5. http://dx.doi.org/10.20517/jeea.2023.46
Download Citation
Export Citation File:
Type of Import
Tips on Downloading Citation
Citation Manager File Format
Type of Import
Direct Import: When the Direct Import option is selected (the default state), a dialogue box will give you the option to Save or Open the downloaded citation data. Choosing Open will either launch your citation manager or give you a choice of applications with which to use the metadata. The Save option saves the file locally for later use.
Indirect Import: When the Indirect Import option is selected, the metadata is displayed and may be copied and pasted as needed.
About This Article
Special Issue
Copyright
Data & Comments
Data
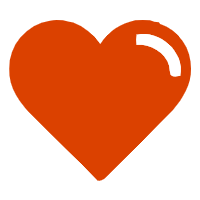
Comments
Comments must be written in English. Spam, offensive content, impersonation, and private information will not be permitted. If any comment is reported and identified as inappropriate content by OAE staff, the comment will be removed without notice. If you have any queries or need any help, please contact us at support@oaepublish.com.