A look at MERCs as UPRmt regulatory hubs in age-associated heart diseases
Abstract
With the increase in life expectancy globally, the challenge of dealing with aging becomes more prominent. Aging is a risk factor for several diseases, including cardiovascular disease. Mitochondria, which have long been studied in relation to aging, play a crucial role in maintaining cellular homeostasis. However, there is a limitation in interorganellar communication as organisms age. The unfolded protein response in mitochondria (UPRmt) is activated during stress to maintain mitochondrial homeostasis and prevent the accumulation of damaged mitochondria. This response involves signaling from the mitochondria to the nucleus, leading to transcriptional changes. In the context of aging heart, this review explores the role of mitochondria in terms of function and morphology. It also discusses the impact of UPRmt on cardiac diseases such as heart failure, acute myocardial infarction, and dilated cardiomyopathy. The review also highlights the potential role of mitochondria-endoplasmic reticulum contact sites (MERCs) in modulating UPRmt during aging. Finally, it provides an update on molecules that induce UPRmt activity, potentially benefiting the aging heart with cardiac disease.
Keywords
INTRODUCTION
Proteotoxic stress is a common challenge for all organisms, and several mechanisms are involved in the defense against stress. Unfolded protein responses (UPRs) are triggered by the accumulation of unfolded or misfolded proteins in cells and occur in response to an imbalance between local protein biosynthesis, loading, and folding in different subcellular compartments, including the endoplasmic reticulum (UPRER), mitochondria (UPRmt), and cytoplasm (UPRcyto) through distinct mechanisms[1]. Maintenance of proteostasis requires a close association with energy conversion to support proper protein folding. UPR identifies misfolded proteins within the ER but is triggered by cytosolic protein aggregation, while the integrated stress response (ISR) is activated by stress signals originating from both the ER and the cytosol[2].
The ER senses and responds to cellular stress triggered by metabolic imbalances or protein folding during the progression of various diseases[3]. UPRRE is activated upon alteration of the ER chaperone-protease transcription network to promote the recovery of organelle homeostasis[4] through two phases: (a) reducing protein synthesis and increasing degradation of misfolded proteins; and (b) initiating transcriptional regulation of target genes responsible for global control of proteostasis[2]. In general, UPRER is controlled by three protein sensors, inositol-requiring enzyme 1 (IRE), PKR-like endoplasmic reticulum kinase (PERK), and activating transcription factor 6 (ATF6), which modulate a selective response (adaptive or signaling pathway) to preserve cellular physiology[3]. Meanwhile, UPRcyto is induced by misfolded cytosolic proteins (e.g., VHL, tumor suppressor gene) or non-glycosylated viral protein precursors that do not enter the secretory pathway at all[5,6]; this response is characterized by the action and regulation of multiple chaperone proteins, such as heat shock proteins (Hsp70 and Hsp90) and a subset of J-domain chaperone proteins in the cytosol[1].
Mitochondria are involved in several cellular functions, including energy generation, programmed cell death, regulation of calcium (Ca2+) levels, and lipid metabolism[7]. Yet, these mechanisms are impaired in the presence of reactive oxygen species (ROS), electron transport chain (ETC) uncoupling, and aberrant protein expression that promotes mitochondrial dysfunction[8]. This is more evident with advancing age and governs the establishment and progression of age-related diseases[9]. In addition, mitochondrial homeostasis is maintained by distinct mechanisms of mitochondrial quality control, and one activated response to resolve stress within the mitochondria is UPRmt. Conversely, when organelle damage is severe, signals for mitochondrial destruction by mitophagy are sent, thus preventing further damage to the mitochondrial network and allowing the cell to recover its homeostasis[10].
UPRmt is a nuclear transcriptional response that maintains or restores mitochondrial proteostasis via a mitohormetic response in the mitochondria. This response upregulates glycolysis and downregulates the expression of respiratory chain subunits to balance cellular energy and unload mitochondrial translation and folding machinery[11]. Notably, in aged organisms with multimorbidity, the UPRmt is activated, extending life expectancy. For example, in Caenorhabditis elegans, it promotes protein homeostasis by alleviating protein aggregation in neurons and increasing nematode longevity[12]. However, partly because of UPRmt activation, OSCP (a subunit of Complex V) loss promotes the opening of the mitochondrial permeability transition pore (mPTP) and shortens the lifespan of nematodes[13]. Derived from this double-edged sword, in this review, we describe the effects of UPRmt in cardiac aging models, owing to the enormous impact that cardiovascular diseases (CVDs) have on patients' quality of life and disability, coupled with the close connection between cellular senescence and fatal cardiac outcomes in most CVDs in elderly individuals[14], making early detection of cardiac degeneration, preventive measures, and treatment of these diseases imperative to promote healthy aging[15].
Through different disciplines, we have been able to obtain clues regarding the existence of interorganellar contacts. Using biochemistry, we have obtained the co-purification of membranes of different organelles; cell biology provides visualization of close associations and interorganellar links, whereas cell physiology describes cellular functions that require local interactions of adjacent organelles[16]. Thus, the harmonization of these findings reveals a diversity of nanoscale structures with distinctive functions but with shared characteristics among organelle contacts[17]. So, based on these observations, and after employing specialized, high-resolution detection methods, we have been able to visualize the different contacts, envisioning a new field of study that we call contactology[16].
In particular, the connections between mitochondria and ER allow efficient communication that promotes cellular homeostasis through mitochondria-ER contact sites (MERCs) at different levels. For example, loss of MERCs is linked to decreased mitochondrial membrane potential and increased ROS in senescent vascular smooth muscle cells[18]. In neurons, decreased MERCs interaction induces mitophagy, Ca2+ dysregulation, and respiratory capacity, leading to neurodegeneration[19]. In skeletal muscle, depletion of MERCs leads to alterations in mitochondrial lipidomics and Ca+ influx, which are associated with instability of the mitochondrial-RE junction due to loss of tethering proteins[20,21]. Therefore, these studies highlight the structure and regulation of MERCs as potential targets to improve mitochondrial function. Moreover, in different pathological situations, such as diabetes and obesity, as well as in the field of cellular senescence[22,23], there has been growing interest in the study of MERCs. However, the relationship between MERCs, UPRmt, and aging, especially in the development of CVDs, remains unclear. Therefore, in this review, we link UPRmt changes to communication through MERCs during aging, recalling that these microdomains harbor UPRER (as recently reviewed[24,25]), with an outstanding debt on what occurs in the aging heart.
OVERVIEW ON AGING
Aging is an accumulation of life's consequences, such as molecular and cellular damage, leading to functional decline, chronic diseases, and ultimately mortality[26]. This process is constantly evolving in numerical terms; by 2030, one in six people in the world will be 60 years old or older. In 2050, the global population of people aged 60 years and over will be 2.1 billion, double by 1.4 billion in 2020. Notably,
Throughout history, there have been many questions about the aging process; however, to date, we do not have clear and definitive answers. In light of this, the Aging Biomarker Consortium (ABC) argues that if we can accurately determine our biological age (rather than chronological age), identify exactly why some people are more susceptible to certain age-related diseases (such as heart disease), and assess whether the efficacy of geroprotective treatments is universal, we would have the opportunity to establish biomarkers for aging and predict the transition to a pathological state[27]. However, even if we were close to achieving this, multiple aspects of aging would be involved, and attention to only one aspect would counteract the progress of others.
As we have described, the increase in the elderly population has been fruitful thanks to medical interventions that have helped prevent the death of elders from age-related diseases; however, simultaneously, the number of older adults suffering from diseases has increased, so the ratio of quality of life to longevity is decreasing. Undoubtedly, the strategy that has set the standard for moving toward healthy aging is intervention with an anti-aging approach that slows the onset and progression of age-related diseases that (theoretically) would enable the life quality vs. longevity balance to be restored. However, it is very difficult to achieve a balance between both factors - living many years without deterioration while maintaining good physical and mental health.
Traditionally, age-related diseases have been investigated separately; however, this approach must be modified to slow the deterioration associated with aging. Geroscience is an interdisciplinary field dedicated to understanding the complex relationship between aging and age-related diseases. It has a boom and greater scope as an integrative scientific field, whose goals can be summarized as follows: (1) to identify biological processes and pathways that contribute to, influence, and/or regulate the deterioration of aging; and (2) to have an integrated view of potential targets to develop therapeutic strategies that help limit disease progression with age[28]. To achieve these goals, we started with different markers that helped to lay the molecular foundation for aging.
In their most recent publication, López-Otín et al. added three new features (deactivated macroautophagy, chronic inflammation, and dysbiosis)[29] to those already enunciated ten years ago[30]. These hallmarks are grouped into the following categories: (1) primary, which is the main cause of age-associated molecular damage; (2) antagonistic, which determines the beneficial and harmful effects on the health of the organism; and (3) integrative, which involves the alteration of intercellular communication[31]. Each of these categories has specific study targets that have helped corroborate the premise of interdependence in these hallmarks; that is, the experimental attenuation or accentuation of a specific marker often affects other traits, underscoring that aging is a complex process that must be conceived as a whole[29].
One of the main hallmarks of aging, perhaps the most widely studied one, is mitochondrial dysfunction. This is because mitochondria are especially necessary in tissues with high energy demand, such as the heart, which continuously requires adenosine triphosphate (ATP) to maintain its activity. Conversely, malfunction is considered a key factor in CVDs, leading to decreased ATP production, increased ROS levels, apoptosis, and mitochondrial dynamic imbalance[32]. In addition to its undeniable involvement in impaired proteostasis, it leads to destabilization of respiratory complexes and reduced organelle turnover (see below).
Significant strides have been made in the field of aging to the point that aging is an unequivocal risk factor for the development of many chronic diseases. This arduous research has facilitated the identification of therapeutic targets for preventing and treating aging and its diseases, as well as the development of useful pharmacological agents for clinical approval in the future[33], as has been the case with senotherapy for various age-related chronic diseases, including heart disease[34-36].
MITOCHONDRION AND CARDIAC AGING
Mitochondria play an important role in aging, but the link between mitochondrial dysfunction and age-associated diseases remains unclear[37]. Cardiac aging is associated with the maladaptation of cellular metabolism, cardiomyocyte senescence, decreased angiogenesis, and increased fibrosis, which together induce cardiac remodeling and dysfunction[38]. Structural and functional changes in the aging heart have been documented in the literature (please review[39-41]). Therefore, we focused on describing mitochondrial changes related to their function, morphology, and dynamics in aging organisms [Figure 1].
Figure 1. Structural and functional changes in aging mitochondria. With age, both mitochondrial populations undergo alterations in metabolism, which contributes to the development of cardiovascular diseases (CVDs). Subsarcolemmal mitochondria (SSM, located below the membrane) also show lamelliform, swollen cristae, and an increased presence of calcium (Ca2+). Interfibrillar mitochondria (IFM, which resides between myofibrils) have tubular cristae, reduced bioenergetic capacity, and less respiration. Additionally, both mitochondrial types showed higher capacity for mitochondrial fission. Created using BioRender (www.app.biorender.com).
Bioenergetic mitochondrial
Although mitochondrial dysfunction is believed to change with age, ambiguous results have been reported. For example, mitochondrial respiration in cardiomyocytes isolated from 20-month-old rats is similar to that in young rats[42]. In contrast, mitochondria from old mice (2 years old) showed reduced oxygen consumption during oxidative phosphorylation (OxPhos) of complexes I and II, as well as depolarized mitochondria with reduced ATP production[43] and evident ROS production[42,43]. Transcriptional analyses have highlighted significant reductions in the expression of ETC genes throughout their lifespans[44]. Furthermore, systematic analysis of the respiratory activity of 33 different tissues in 20-month-old mice of both sexes revealed that mitochondrial activity was very low in the heart, adipose tissue, and kidneys compared to that in young mice[45]. This compendium robustly complements transcriptomic and proteomic analyses of changes recorded in rodent tissues[46,47] and patients as they age[48,49]. The structure of the heart is intimately connected to the presence of two mitochondrial populations with different activities[50]. Interfibrillar mitochondria (IFM) have been described to be less abundant and defective in respiration[51]. However, during ischemia-reperfusion (IR) in adult rats, mitochondrial swelling in the presence of Ca2+ was greater in the subsarcolemmal (SSM) than in the IFMs[52]. Furthermore, Complex III of SSMs and IFMs is reported to be dysfunctional in adult and old animals after 25 min of global ischemia; however, in IFMs from the aged heart, ischemic damage in Complex III was additive to age[53]. Therefore, mitochondrial susceptibility is strongly influenced by age and oxidative stress.
Structural differences between these two types of mitochondria have also been observed in older organisms. In particular, the cristae (folds of the mitochondrial inner membrane) of SSMs are lamelliform and tubular, whereas those of IFMs are mostly tubular[50]. Strikingly, in numerical terms, there was no significant difference in the protein yield (mg/g heart tissue) of SSM and IFM between the 3- and
Furthermore, the morphological arrangement of the cristae determines the energetic capacity of the mitochondria as they host ETC complexes that are responsible for establishing the driving proton force to produce ATP[40,55]. Thus, senescence-induced cardiomyocytes treated with doxorubicin exhibited proton-sink malfunction. Oddly, increased confinement of the enzyme to the cristae was detected by single-molecule monitoring of ATP synthase, indicating that the spatiotemporal organization of ATP synthase coordinates with dysfunction in ATP production during senescence[56]. Indeed, cristae are maintained by optic atrophy protein 1 (OPA1) and the mitochondrial contact site and cristae organizing system (MICOS), which exhibit changes with advanced age. For instance, cardiomyocytes from 6-year-old sheep show low expression in mic60 (a.k.a. Mitofilin) and opa1, in correlation with the scarce presence of cristae with their wider membranes, as well as significant mitochondrial fragmentation[57].
In a study of cellular senescence induced by doxorubicin in human induced pluripotent stem cell-derived cardiomyocytes (hiPSC-CMs) and cardiomyocyte primary cultures, mitochondria with a swollen morphology, truncated crests, and lower density were observed, as well as a higher width/length ratio, indicating changes in their shape compared to non-senescent cells[58]. In contrast, three-dimensional analysis of the ultrastructure of mitochondria from 2-year-old mice revealed a smaller number of mitochondria with a lower and more circular mitochondrial area, with a reduced number of ridges and evident loss of their cristae folds due to aging[37]. Similarly, brown adipose tissue from old rats (2 years old) showed increased mitochondrial volume and surface area, along with reduced volume, area, and perimeter of mitochondrial cristae[59], indicating ultrastructural changes in mitochondria with aging.
Mitochondrial dynamics
Mitochondrial plasticity refers to the ability of mitochondria to fuse, divide, and contact other organelles to maintain the flow of metabolites and perform cellular functions. Fusion/fission is activated in response to stimuli that alter the bioenergetic capacity of the mitochondria to maintain homeostasis. Moreover, these dynamics determine many aspects of an organism's health span, such as longevity, stress resistance, and disease response[60]. Although imbalances in mitochondrial dynamics are evident in old age (e.g., increased mitochondrial fission), this scenario is accentuated when damage/disease conditions are present. In an IR model of old Wistar rats (22-24 months old), low levels of mitofusin 2 (Mfn2) and increased dynamin-related protein 1 (Drp1) were observed, which improved after co-treatment with nicotinamide mononucleotide and melatonin[61]. In addition, increased mitochondrial elongation enables the survival of old animals through the regulation of longevity pathways such as insulin-like growth factor 1 (IGF-1) signaling[62] or transcription factor B (TFEB, a major regulator of autophagy and lysosomal biogenesis)[63].
On the other hand, peroxisome proliferator-activated receptor gamma (PPARγ) coactivator 1α (PGC-1α) interacts with leucine-rich pentatricopeptide repeat-containing (LRPPRC) protein in the nucleus and regulates the mitochondrial biosynthesis of nuclear gene expression. Nevertheless, this pathway is closely related to UPRmt in SH-SY5Y neuroblastoma cells (a cell line widely used to study the pathogenesis of Parkinson’s disease), specifically yeast mitochondrial escape 1-like-1 (YME1L), mitochondrial caseinolytic protease P (ClpP), and heat shock proteins A1 and E1 (HspA1 and HspE1) after 24 h of MMP+ treatment when PGC-1α is overexpressed[64]. Lastly, impaired expression of type II iodothyronine deiodinase (DIO2, a member of the selenoprotein family that localizes mainly in the myocardial ER) in hiPSC-CMs severely affects genes involved in mitochondrial biogenesis (e.g., PGC1α), mitochondrial dynamics (e.g., Mfn2), in UPRmt (e.g., activating transcription factor 4, ATF4, and Hsp70), as well as maximal respiration and reserve respiratory capacity, suggesting a key role of DIO2 as a regulator of mitochondrial function in human cardiomyocytes with significant implications in age-related CVDs[65].
Mitochondrial ROS generation
Broadly, mitochondrial dysregulation is characterized by the accumulation of damaged and dysfunctional mitochondria that promote the generation of more ROS, thereby perpetuating mitochondrial damage[9]. Distinctly, the aging heart exhibits decreased OxPhos (especially in state 3 respiration) associated with Complexes I and IV, as well as increased electron leakage and robust mitochondrial production of ROS that contribute to oxidative damage (particularly affecting IFM)[66], which, in concert, contributes to the establishment and progression of age-related CVDs such as heart failure (HF), acute myocardial infarction (AMI), and cardiomyopathies. Thus, mitochondria remain an attractive target not only because they function as the powerhouse of the cell but also because they are a central signaling hub for controlling the rate and quality of aging through the activation of UPRmt. In the next section, we update our knowledge on the impact of this response on mitochondrial function.
UPRmt SIGNALING AND ITS ROLE IN MITOCHONDRIAL FUNCTION AND STRESS RESPONSE
UPRmt is a transcriptional response triggered by mitochondrial defects during OxPhos, ROS production, ETC dysfunction, imbalance between mitochondrial and nuclear proteins, unfolded proteins, and exogenous stress (e.g., hypoxia, pathogen infections, and xenobiotics)[67,68]. This response was initially investigated in depth in Caenorhabditis elegans[69,70] with relevant implications for nematode longevity[71,72]. However, in the early 2000s, it was described that COS-7 cells transfected with a misfolded mutant of ornithine trans-carbamylase, involved in the urea cycle, accumulated this protein and thus overexpressed nuclear-encoded mitochondrial chaperones and proteases in response to mitochondrial stress[73].
Different mechanisms promote UPRmt activation, with the canonical ISR axis being the most studied, involving four protein kinases that uniquely respond to stress signals, which phosphorylate eukaryotic translational initiation factor 2α (eIF2α) at one of the three subunits of the eIF2 complex. These kinases include PERK, heme-regulated inhibitor (HRI), general control non-derepressible 2 kinase (GCN2), and double-stranded RNA-dependent protein kinase (PKR)[2,74]. Broadly speaking, the goal of UPRmt is to reduce the global translation rate by favoring the translation of mitochondrial proteases and chaperones to alleviate and promote recovery from mitochondrial stress [Figure 2]. In Caenorhabditis elegans, the UPRmt is orchestrated by the nuclear accumulation of activating transcription factor associated with stress-1
Figure 2. UPRmt signaling pathway and its role in preserving mitochondrial integrity in mammals. UPRmt acts in the defense against mitochondrial stress through mitochondrial stress-nucleus communication. The unfolded proteins in the matrix and/or IMS initiate the activation of different proteins involved in UPRmt. Following this signal, the development of this response is initiated through the activation of proteins such as JNK, SIRT3, eIF2α, and ERα, which, in turn send signals to the nucleus to induce UPRmt via other proteins (e.g., CHOP) and transcription factors (e.g., c-Jun, FOXO3, NRF1, OMI/HTRA2) to promote the transcription of genes involved in halting mitochondrial damage and preserving mitochondria in their healthy state. IMS: Intermembrane space; JNK: Jun N-terminal kinase; SIRT3: Sirtuin 3; eIF2α: eukaryotic translational initiation factor 2α; ER-α: Estrogen receptor α; CHOP: CCAAT/enhancer binding proteins (C/EBP) homologous protein; FOXO3: Forkhead box O3; NRF1: Nuclear respiratory factor 1; OMI/HTRA2: High-temperature protein A2. Created using BioRender (www.app.biorender.com).
When the efficiency of the mitochondrial machinery is reduced in mammals, the activation of eIF2α/ATF4/CCAAT/enhancer binding protein (C/EBP) homologous protein (CHOP) signaling is initiated [Figure 2]. This signaling pathway encodes chaperones (e.g., Hsp10 and Hsp60), antioxidant enzymes, ClpP proteases, and the mitochondrial Lon protease (LonP1). Mechanistically, when misfolded proteins accumulate in the mitochondrial matrix, they are cleaved by ClpP or LonP1 and converted into small peptides[8,69,78], which in turn activate Jun N-terminal kinase (JNK) while PKR activates CHOP, and subsequently induce the transcription factor c-Jun[79]. Oddly, the CHOP element (GC/ATTGCA) is the mitochondrial stress response element that, in association with C/EBPβ, upregulates the expression of mitochondrial stress genes, such as YME1L, in response to the accumulation of unfolded proteins in the mitochondrial matrix. In contrast, mitochondrial protein genes (such as LonP1) do not have a CHOP element and are therefore not upregulated[73,80]. Furthermore, when specific stress occurs in the matrix, eIF2α is phosphorylated by the GCN2 kinase, increasing the translation of CHOP and activating transcription factor 5 (ATF5) [Figure 2].
Overproduction of ROS and the accumulation of unfolded proteins in the intermembrane space (IMS) activate estrogen receptor-α (ER-α), which triggers the activation of high-temperature protein A2 (HtrA2/Omi), a nuclear-encoded serine protease located in the IMS. HtrA2/Omi is released from the mitochondria into the cytosol and nucleus in response to stress, inducing caspase-independent and caspase-dependent apoptosis. In contrast, under normal conditions, Omi1/HtrA2 is restricted to IMS, where protease activity is involved in the degradation of undesirable proteins to maintain mitochondrial proteostasis[81]. In addition, protein quality control depends on HtrA2/Omi and proteasome upregulation, which increases the transcription of nuclear respiratory factor 1 (NRF-1), thereby protecting the mitochondria by preventing mitochondrial potential loss [Figure 2][78]. However, a low mitochondrial membrane potential can activate UPRmt to help stress-resilient cells restore mitochondrial function[82,83]. For instance, treatment with auraptene increases the expression of junctional proteins (e.g., occludin and claudin-5), which prevent pathogens from crossing into the brain. This protective response is accompanied by an increase in ATF5, LonP1, Hsp60, CHOP, and antioxidant enzymes regulated by NF-E2-related factor 2 (Nrf2)[84].
UPRmt signaling and aging
It is widely reported that in Caenorhabditis elegans, ATFS-1 acts as the master regulator of UPRmt; however, its activation alone is not sufficient to extend lifespan[72]. In contrast, the first studies linking UPRmt to aging dated back over 10 years ago in Surf1 knockout mice. Specifically, LonP1 levels were found to be increased, Hsp60 and ClpP levels were not modified, and CHOP levels tended to increase in the heart, although not significantly. However, all of these proteins were higher in the skeletal muscle of Surf1 knockout mice[85]. In fact, forced expression of UPRmt genes in muscles was sufficient to preserve mitochondrial function and delay age-related locomotor impairment[86]. LonP1 deficiency induces altered cardiac ventricular development and cardiomyocytes are characterized by mitochondrial swelling, loss of cristae, and protein aggregates[87]. In the kidneys of aged humans and mice, reduced LonP1 expression is linked to renal fibrosis and mitochondrial dysregulation, which is alleviated after LonP1 is overexpressed[88].
In the Surf1 knockout mice, the expression of genes involved in UPRmt in skeletal muscle, as well as the expression of Nrf2 and heme-oxygenase-1 (HO-1), does not differ from that of the wild-type[85]. In this regard, in a nematode model of the CL2006 strain expressing β-amyloid, Lycium barbarum treatment induced less follicle-stimulating hormone receptor-1-mediated activation of UPRmt and inhibited ROS production via upregulation of the antioxidant system, thereby ameliorating mitochondrial damage and reducing the progression of Alzheimer’s disease[89]. These data indicate that loading of misfolded proteins into the mitochondrial matrix also activates the antioxidant-UPRmt axis through activation of sirtuin 3 (SIRT-3). SIRT3 deacetylates the transcription factor forkhead box O3a (FOXO3a), causing its nuclear transport to the nucleus, where it activates the transcription of antioxidant enzymes such as catalase and superoxide dismutase 2. However, it remains unclear how SIRT3 is associated with the expression of UPRmt-related genes[76,90]. In Figure 3, we summarize the signaling molecules involved in UPRmt activation and the differences between damaged young cardiomyocytes and injured aged cardiomyocytes. UPRmt proteins are common in cardiomyocytes of both age groups, and their impact on mitochondrial function is also represented. Given a brief glimpse of the signaling pathways involved and best documented in UPRmt, in the following paragraphs, we describe their linkage to CVDs and aging.
Figure 3. Proteins that induce UPRmt activation in young and aged damaged cardiomyocytes and their role in mitochondrial function. Proteins that promote UPRmt activation in young, damaged cardiomyocytes are illustrated in blue, and aged cardiomyocytes in yellow. Proteins common to both age groups, which activate UPRmt, are represented in green. In young, damaged myocytes, mitochondrial respiration and biogenesis are decreased, while mitophagy is increased (pink squares). In contrast, in aged cardiac cells, mitochondrial bioenergetics improve and mitophagy decreases following UPRmt activation by the Lon1 peptidase (LonP1), yeast mitochondrial escape 1-like-1 (YME1L), and fibroblast growth factor 21/sirtuin-3 (FGF21/SIRT3, purple squares). HSP: Heat shock protein; ATF5: transcription factor-5; FUNDC1: FUN14 domain containing 1; DIO2: type II deiodinase; ClpP: mitochondrial caseinolytic protease P; Oma1: stress-activated protease 1 m-AAA; CHOP: C/EBP homologous protein; HtrA2: high-temperature requirement protein A2; DDIT3: DNA damage-inducible transcript 3; UBL5: ubiquitin-like protein 5; OPA1: optic atrophy protein 1; Fis1: mitochondrial fission protein 1. Created using BioRender (www.app.biorender.com).
UPRmt IN AGE-RELATED CARDIOVASCULAR DISEASES
The development of diseases is an inherent and inevitable fact that occurs when organisms age, a scenario that worsens when there is gradual deterioration of mitochondrial function that contributes to reduced cellular function. It is at this time that the UPRmt is responsible for promoting the repair and recovery of mitochondrial homeostasis through compensatory processes related to proteostasis and the antioxidant system, thus achieving the regulation of mitochondrial dysfunction in age-related diseases[91,92]. Indeed, it has been suggested that moderate mitochondrial stress and subsequent activation of UPRmt delay the aging process and thereby is a therapeutic target for the treatment of age-associated diseases[93].
Specifically, the heart and its specialized cells have high mitochondrial abundance. Consequently, many CVDs such as HF, hypertension, and IR injury are associated with defects in OxPhos and mitochondrial structural changes[94], thus promoting endothelial dysfunction, inflammation, and cardiomyocyte death[8]. In the face of these damage events, activation of UPRmt could improve mitochondrial function and myocardial contractility, reduce cardiomyocyte mortality, and ameliorate the processes of fibrosis and pathological remodeling[95]. Increased risk of CVDs is associated with metabolic syndrome, and trimethylamine N-oxide (TMAO) is linked to these disorders. Research has shown that TMAO promotes metabolic dysfunction by binding to and directly activating the PERK/FOXO1 pathway[96]. In healthy humans, plasma TMAO is higher in older individuals (64 ± 7 years) than in younger individuals (22 ± 2 years), which is inversely related to brachial artery dilatation. Indeed, dietary supplementation for six months with TMAO induces an aging-like impairment in endothelium-dependent dilatation of the carotid artery in young mice (8 weeks of age)[97].
Changes in cardiac metabolism are closely associated with the onset and progression of CVDs. In this light, the increase in mitochondrial bioenergetics reduces apoptosis and tissue damage, suggesting that the rise in UPRmt in cardiomyopathies is also related to this response. Accordingly, silencing of DIO2 in hiPSC-CMs induces the downregulation of genes involved in mitochondrial biogenesis (e.g., PGC1α and PPARα) and fatty acid metabolism (e.g., acetyl-CoA carboxylase α and β, and fatty acid synthase), which are key factors in the regulation of energy metabolism. Consequently, UPRmt activation and mitochondrial bioenergetics decrease, inducing ROS production and reducing cell viability[65]. In addition, the activation of UPRmt by oligomycin induces glucose and oxygen consumption in neurons under hypoxic stress[98]. In line with this, it was observed in cardiac IR mice that ATF5 activation significantly induces the transcription of several UPRmt-linked proteins and upregulation of mitochondrial and glycolysis genes that collectively ameliorate cardiac damage compared to ATF5 knockout mice[11]. It has also been reported that the LonP1/ATF4 axis activates UPRmt, which reduces hepatic steatosis and increases oxidative metabolism, leading to reduced fat mass and improved mitochondrial function in skeletal muscle[99], a phenomenon that could also occur in cardiac muscle. Thus, UPRmt activation plays a crucial role in the regulation of glucose and fatty acid metabolism in cardiomyocytes, especially under stressful conditions. These data emphasize the possibility of using UPRmt activation as a treatment for cardiometabolic diseases and even slowing the progression of these age-related diseases. At the time of writing this review, a manuscript on the involvement of UPRmt in CVDs was published (please review[100]); however, the influence of age on UPRmt has not been discussed. In the following sections, we describe the mechanisms of this response in the three most prevalent cardiac diseases in the elderly.
Heart failure
According to the American Heart Association, Heart failure (HF) is a syndrome in which the heart pumps an insufficient amount of blood to meet whole-body needs. Without delving deeper, HF with preserved ejection fraction (EF) (pEF, i.e., diastolic HF) and reduced EF (rEF, i.e., systolic HF) presents with symptoms such as shortness of breath, fatigue, congestion, and peripheral edema[101]. Regardless of the HF type, age is a major risk factor for disease progression and is more prevalent in older women. In addition, the constant structural and functional changes that the heart undergoes with increasing age support disease progression[102,103].
Mitochondrial dysfunction (including impaired ATP production, oxidative stress, and cell death) and ER stress have been identified as pathogenic cooperators in HF progression[104]. For example, mice with cardiac dysfunction and pulmonary edema, in parallel with an increase in glucose-regulated protein 78, Derlin-1, and CHOP, as well as a full accumulation of misfolded and polyubiquitinated proteins, confirmed increased ER stress[105]. In a cardiometabolic stress-induced HFpEF model derived from the intake of a high-fat diet supplemented with Nω-nitro-L-arginine methyl ester (L-NAME), UPR dysfunction was observed owing to increased nitrosative stress associated with increased nitric oxide production by inducible nitric oxide synthase[106]. In the same model, the expression of STIP1 homology and U-box-containing protein 1 (STUB1, which transcriptionally regulates Xbps1) decreased, mitochondrial redox balance was altered, and ferroptosis increased, events that were mitigated by imeglimine[107], underlining the relevance of UPR in HF progression.
Some proteases and chaperones involved in the UPRmt undergo important changes during HF. For example, low LonP1 expression has been observed in pressure overload-induced HF in young mice (10-12 weeks old), which is related to increased oxidative damage, impaired mitochondrial respiration, and contractile dysfunction[104]. Recent data show that boosting UPRmt with nicotinamide riboside mitigates mitochondrial and contractile dysfunction in vitro and in mice subjected to chronic hemodynamic overload (8-10 weeks old), suggesting a protective role for this response in stressed hearts[108]. Furthermore, in 8-week-old mice with pressure overload-induced HF, overexpression of YME1L induced cardioprotection through reduced mitochondrial fragmentation, enhanced respiratory control, and increased mitochondrial ATP, in association with more efficient fatty acid storage and utilization. These improvements positively impact cardiac function as EF improves and chamber diameter decreases[109].
Although not in the context of HF, the LonP1/ClpP proteolytic quality control axis extinguishes ROS in depolarized mitochondria by degrading the ROS-generating domain of Complex I in SH-SY5Y and HeLa cells[110]. However, UPRmt does not always play a protective role; in this regard, 7-week-old Hsp60 knockout mice exhibit premature death due to chamber dilation, ventricular wall thinning, and pulmonary edema. After weeks of evolution, EF, shortening fraction, and systolic function progressively worsened. In addition, rodents with HF presented abnormal accumulation of mitochondrial proteins, leading to their death[111,112].
Using a reporter system (hsp-6::GFP) in Caenorhabditis elegans, metolazone (a diuretic used in congestive HF) induced the expression of mitochondrial chaperones and significantly extended the longevity of worms through ATFS-1 and ubiquitin-like protein-5 (ULB-5)[93]. In patients with an EF of less than 35% and an average age of 40 years, reduced expression of mortalin, YMEIL1, LonP1, and stress-activated protease 1 m-AAA (Oma1) was observed[113]. Moreover, a recent study reported that patients with HF (57 ± 1 years old) had a positive correlation between UPRmt and zinc and a negative correlation between iron and YME1L protease, leading to the activation and inhibition of UPRmt, respectively, which could affect the acceleration of HF[114]. Finally, in samples from patients with an average age of 73 years and diagnosed with aortic stenosis (a clinical model of chronic pressure overload), a significant increase in ClpP and CHOP expression was reported to be associated with reduced death cardiomyocytes[108]. These data suggest that the UPRmt is a timely area of study that limits pathological cardiac stress during aging.
Acute myocardial infarct
Advances in Acute myocardial infarct (AMI) prevention have resulted in a significant decline in its incidence; however, the mortality rate is high, especially in elderly patients, as in HF[115]. AMI is characterized by a sudden decrease or interruption of coronary flow and oxygen delivery to the tissue caused by an obstruction in the coronary arteries (atherothrombosis), which triggers inflammation and apoptosis and promotes tissue damage and loss of contractile function[116,117].
In older rodents and elderly patients (60-89 years old), a lower capacity to tolerate ischemia was observed[67,118], indicating that age positively influences the outcome of AMI. Oxidative stress, Ca2+ overload, inflammation, and activation of apoptosis have been identified as central players in the pathological process of the disease. Mitochondrial dysfunction is strongly associated with AMI development and progression. Recent studies have identified genes differentially expressed in mitochondria (MitoDEGs) as potential biomarkers in AMI patients, reinforcing the importance of mitochondrial function in this pathology[116].
Proteases and chaperones involved in UPRmt have been extensively explored because of their cardioprotective properties in murine AMI models. For example, LonP1 protects 6-8-month-old mice from IR through mitochondrial redox balance and bioenergetic reprogramming[119]. Instead, pharmacological activation of UPRmt with oligomycin or doxycycline does not protect 12-20-week-old ATF5 knockout mice undergoing cardiac IR, indicating that cardioprotection is ATF5-dependent[11]. Similarly, mice undergoing 24 h of reperfusion and treated with oligomycin exhibited reduced infarct size, improved contractile function, and decreased markers of cardiac damage through FUN14 domain containing 1 (FUNDC1, an adaptor of mitophagy) as a mediator upstream of UPRmt[120], indicating that activation of this response could be a strategic target for cardioprotection.
LonP1 was one of the first proteins to show interesting changes during aging, prompting further study. Early studies with oligonucleotide-based arrays explored the transcriptional response in the gastrocnemius muscle of 30-month-old mice. This study showed that 13% of genes involved in mitochondrial turnover and function were altered. Interestingly, the induction of 76% caloric restriction from 2 to 30 months of age prevents the activation of stress response genes and the processing of damaged or misfolded proteins during aging[121]. Years later, using gene expression analysis of heart samples from old mice (30 months old) undergoing exercise, an increase in the half-life (17%) of these mice was found to be associated with overexpression of energy metabolism genes, such as LonP1, compared to sedentary mice[122].
Although not related to aging, dietary preconditioning for 2 h per day for 7 days was sufficient to improve the outcome of kidney injury in young rats (8-week-old). These benefits are associated with reduced mitochondrial fragmentation and modulation of LonP1-regulated UPRmt[123]. Despite its poor clinical application, these results suggest that the implementation of strategies such as exercise or caloric restriction are timely options that would aid in the recovery of LonP1 transcription, protein abundance, and activity levels that are ablated in aged cells. Finally, the expression and protein levels of HtrA2/Omi significantly increased in old rats’ hearts (20-24 months old), promoting greater apoptosis through the endogenous regulator of cell death X-linked apoptosis inhibitory protein. Cytosolic HtrA2/Omi levels were higher in the aged group subjected to IR than in the heart of adult rats[124]. Notwithstanding the progress in our knowledge of UPRmt and aging, the relationship between both variables and AMI remains scarce, and new studies are required.
Dilated cardiomyopathy
Left ventricular enlargement and global deterioration of systolic function (EF < 45%) in the absence of coronary artery disease or increased load are the defining features of Dilated cardiomyopathy (DCM)[125]. In addition, impaired metabolic energy and mitochondrial dysfunction follow systolic dysfunction and eventually HF[126].
DCM patients aged 59 years have low expression of DNA damage-inducible transcript 3 and UBL-5, the regulatory elements of UPRmt[113]. Sprague-Dawley rats with neurogenic hypertension-induced DCM exhibit mitochondrial changes at morphological and bioenergetic levels that are associated with an increased presence of ROS. Specifically, mitochondrial fission 1 protein levels increase, whereas OPA1 levels decrease, suggesting lower mitochondrial biogenesis[127]. Indeed, low OPA1 levels are associated with altered mitochondrial dynamics in the presence of small mitochondria with dilated cristae, inflammation, and epithelial senescence in the skeletal muscles of 18-month-old mice and sedentary older adults (53-82 years old)[128]. Furthermore, OPA1 deletion promotes ER stress in primary myoblasts and skeletal muscles of 40-week-old mice[20].
Notably, following the import of OPA1 from the nucleus into the mitochondria, the maintenance of normal mitochondrial morphology depends on the proteolytic processing of this protein. In particular, protease YME1L mediates the turnover of OPA1, thus limiting its total accumulation[129]. Deletion of YME1L induces the proteolysis of OPA1 by Oma1, leading to mitochondrial fragmentation, cell death, fibrosis, and ventricular remodeling, thereby promoting DCM[111]. Moreover, under conditions of metabolic stress, OPA1 processing is dispensable for prolonging lifespan and protecting old OxPhos-deficient Cox-/- mice against mitochondrial cardiomyopathy. Interestingly, this protective framework is related to enhanced communication between the mitochondria and ER[129].
Direct evidence for the involvement of UPRmt in DCM is presented in cardiac-specific DARS2-deficient mice that progressively develop DCM due to increased mitochondrial proteostatic stress and low mitochondrial respiration; however, when the ClpP protease is absent, partial attenuation of the disease and increased longevity of the mice are observed[130]. In the context of senescence, the palmitic/oleic acid combination induces a senescent phenotype in HepG2 cells characterized by oxidative stress, inflammation, and UPRmt by increasing hsp90 and hsp60 expression and decreasing clpP, events that are prevented by lipoic acid treatment[131]. This suggests a relevant role for ClpP in aging, and in the establishment and progression of age-related diseases.
Exercise upregulates fibroblast growth factor 21 (FGF21) in individuals aged 20-60 years, thereby enhancing its cardiometabolic activity. Furthermore, in 26-week-old mice, routine exercise increased Klotho expression through the FGF21/SIRT-3 signaling pathway, thereby reversing mitochondrial dysfunction, oxidative stress, and cardiac fibrosis in mice with diabetic cardiomyopathy[126]. Indeed, overexpression of SIRT-3 protects mitochondria from post-ischemic brain dysfunction through activation of the UPRmt via the FOXO3/Sphk1 pathway. Interestingly, inhibition of both pathways alleviates the favorable influence of SIRT-3 on neuronal function and mitochondrial behavior[132]. Like SIRT-3, SIRT-1 also declines with age; in light of this, 50-68-year-old patients with DCM show significant dysregulation of SIRT-1, but AMP-activated protein kinase (AMPK) phosphorylation is sex-dependent. Oddly, the protein levels of SIRT-3 and HSP60 are lower in male patients with DCM, suggesting that alterations in mitochondrial homeostasis are age-associated and that men with DCM are more susceptible[133]. Although there are few studies on UPRmt in aging, it is possible that SIRT-3 and -1 activation may modify this response and positively influence age-associated CVDs[134]. Figure 4 summarizes the UPRmt pathways involved in CVDs during aging.
Figure 4. Mechanisms involved in the activation of UPRmt in the development of age-associated CVDs. The progression of cardiovascular disease (CVDs) is accompanied by overproduction of ROS, accumulation of unfolded proteins, changes in mitochondrial dynamics, fibrosis, and cardiomyocyte death. UPRmt, as a response to mitochondrial damage, has emerged as a therapeutic target for the treatment of age-associated CVDs as it modulates the mitochondrial repair and recovery processes. In heart failure (HF), decreased LonP1, HSP70/60/9, and YME1L proteases have been reported to cause the accumulation of misfolded proteins inside the mitochondria and, thus, the activation of UPRmt. In acute myocardial infarction (AMI), in addition to being characterized by calcium (Ca2+) overload, inflammation, and oxidative stress, a decrease in LonP1 has also been observed, which activates UPRmt through the p-eIF2α-ATF5 pathway, which induces the latter to enter the nucleus. In dilated cardiomyopathy (DCM), decreased mitochondrial NAD-dependent Sirtuin-3/1 deacetylases (SIRT-3 and -1) and HSP60 activate UPRmt. In the face of this damage, the implementation of strategies (such as exercise and diet) has successfully mitigated UPRmt by modulating the response of LonP1, YME1L, and SIRT-3 in elderly patients with CVDs. ROS: Reactive oxygen species; LonP1: Lon1 peptidase; HSP: Heat shock protein; YME1L: yeast mitochondrial escape protein type 1; p-eIF2α: phosphorylation of eukaryotic initiation factor 2 alpha; ATF5: transcription factor-5. Created using BioRender (www.app.biorender.com).
MERCs AS REGULATORY PLATFORMS OF THE UPRmt IN CARDIAC AGING
UPRmt is a mitochondria-targeted protein modification program that upregulates proteins related to or located in the mitochondria[120]. However, impaired mitochondrial function and integrity activate UPRmt, which in turn is regulated by mitochondrial-nucleus communication[93].
Organelles have a continuous, tight, and functional interaction with each other owing to membrane contact sites (MCSs), a new insight provided by cell biology into organelle function[17]. Nevertheless, interorganellar contactology is a very complex field, as each of these interactions is facilitated by specific and endogenous tethering and spacer proteins that, at the same time, are multifunctional and not exclusive to MCSs[135]. They not only create a physical bridge but also serve distinct cellular functions such as lipid transport, Ca2+ signaling, and organelle inheritance[136,137]. Importantly, proteins found within MCSs can exist as single polypeptide chains or complexes of multiple proteins (monomeric, multimeric, or heteromeric). Moreover, they often possess specific organelle membrane-binding or transmembrane domains[137].
Mitochondria and ER exchange signals to maintain cellular homeostasis through microdomains known as MERCs, usually in the range of 10-80 nm. These platforms differ from mitochondria-associated membranes (MAMs), which are membranes obtained in the laboratory by ultracentrifugation in which proteins that could be part of their machinery can be explored[31]. MERCs are also involved in the regulation of mitochondrial function and ER stress[24]; however, they also depend on features such as size, density, and thickness[20]. For example, in mice transfected with a linker transgene designed for cardiac muscles, an increased number of MERCs was observed. This resulted in the mitochondrial remodeling of large and dense clusters in cardiomyocytes. In addition, vulnerability to acute adrenergic stress, cell death, and mitochondrial Ca2+ overload was lower in adult female mice undergoing IR[135]. Meanwhile, OPA1 deficiency in skeletal muscle promotes increased communication of MERCs in accordance with the increased expression of proteins involved in Ca2+ exchange. Simultaneously, OPA1 deficiency increases ATF4-mediated ER stress, indicating that mitochondrial stress regulates MERC formation through different mechanisms, such as the ISR pathway[20].
MERC dysfunction is closely related to obesity, type II diabetes, CVDs, and aging, which are chronic illnesses characterized by increased oxidative and ER stress[20,31]. In this regard, β-nicotinamide mononucleotide ameliorated mitochondrial dysfunction and ER stress in the liver of mice on a chronic high-fat diet, which is related to increased MERCs interaction, helped improve intracellular ATP, and mitigated lipid metabolic alterations in these mice[138]. In Mfn2 knockout oocytes, the upregulation of genes related to ER is linked to abnormal mitochondrial-ER interactions. Although Mfn2 knockout offspring exhibit hyperglycemia, mitochondrial function and ER alterations do not change in the liver or skeletal muscle[22].
In the hearts of lipopolysaccharide-treated Beclin-1 knockout mice, the function of MAMs decreases. Remarkably, when mice were treated with a peptide activator of this protein, cardiac function improved through the restoration of MAMs[139]. In DCM, impaired metabolic function and mitochondria-RE interactions have important implications for diastolic dysfunction[140]. In contrast, HF mice with marked sinoatrial node dysfunction (16 weeks old) exhibit an abnormal mitochondrial structure and an increased distance from MERCs, leading to Ca2+ mishandling and mitochondrial dysfunction[141].
Collectively, we assert that MAM deficiency and UPRER perturbation are coupled with diastolic dysfunction in CVDs[140]; however, our MERCs-UPRmt-aging triad remains an unsolved crossword puzzle [Figure 5]. In this respect, a couple of studies have led the way in exploring their study. For instance, SIRT-1 is a well-characterized regulator of longevity, but recent findings have shown its enrichment in MERCs and activation of UPRmt in adipose tissue. Downstream of this response, lipid regulation is triggered in MERCs through cooperation between clusterin and SIRT-1. Noticeably, the expression of hsp9, lonp1, and Hsf1 were increased in MERCs isolated from adipose tissue with Adipo-SIRT-1, whereas clusterin deficiency reduced the expression of these genes in MERCs isolated from adipose tissue of SIRT-1 knockout[142].
Figure 5. Proteins involved in mitochondrial unfolded protein response (UPRmt) activation and regulation by MERCs in cardiac aging. Convergence between the endoplasmic reticulum (ER)-mitochondria-nucleus organelles maintains cellular homeostasis. Thus, dysfunction of this communion is intimately associated with the development of cardiovascular disease (CVDs) during aging. In particular, tie proteins (LonP1 and Sirt1) enhance communication between mitochondria-RE contact sites (MERCs), both of which are nuclear-encoded and subsequently translated, identified, distributed, and inserted as anchor proteins between MERCs. Overexpression of Sirt1 and LonP1 increases MERCs and, in parallel, activates UPRmt. SIRT1 regulates energy homeostasis, promotes mitohormesis, and prevents ER stress, whereas LonP1 decreases mitochondrial fragmentation, activates the unfolded protein response of endoplasmic reticulum unfolded protein response (UPRRE), maintains mitochondrial integrity, and decreases oxidative damage. Created using BioRender (www.app.biorender.com).
Nevertheless, it was recently demonstrated that LonP1 deficiency in 10-week-old mouse cardiomyocytes promoted alterations in the integrity of MAMs and induced an increased expression of YME1L. Incredibly, UPRRE activation is more sensitive than UPRmt activation following the loss of LonP1 in cardiac cells. In addition, the loss of this UPRmt protease dramatically shortens life expectancy, with a half-life of 60 weeks, owing to cardiac remodeling and dysfunction, leading to DCM and HF. Thus, the authors indicate that, on the one hand, LonP1 functions as an anchoring protein in MAMs and that, in addition, it is indispensable for cardiac function[4]. Experimental evidence for the involvement of proteins related to UPRmt and their regulation by MAMs/MERCs in aging should be gathered, which will open a gap for further in-depth studies.
UPRmt-TARGETED STRATEGIES TO RESTORE MITOCHONDRIAL FUNCTION IN AGE-RELATED CVDs
The search for therapeutic strategies targeting mitochondrial quality control, specifically UPRmt, has become a topic of current interest. To this end, the implementation of different molecules (natural and/or synthetic) that modulate UPRmt to improve mitochondrial function and reduce cardiomyocyte damage is a novel tool, but little is known about aging. Table 1 lists some examples of molecules that have been studied in cardiac models, specifying their involvement in aging models.
Natural compounds modulate UPRmt in models of cardiac damage and aging
Compound/doses | Diseases induction | Animal model | Mechanism | Aging | Reference |
Oligomycin (no doses) | IR | Not specified | FUNDC1-induced activation of UPRmt | No | [120] |
Apigenin (25 mg/Kg/day, for 4 weeks, via gavage) |
Doxorubicin-induced CM | B6.129-Sirt1tm1Mcby/LguJ | Activation of UPRmt-related genes through ATF5/SIRT-1 pathway | Yes | [144] |
CTPR3 lentivirus (8-9 μL/spot, 1.2 × 1012 vg/mL), via intracardiac. |
HF | C57BL/6J male mice (8-10-week-old) | Upregulation of UPRmt-related genes via ATF5/SIRT-1 axis | Yes | [154] |
Zishen Tonyang Houxue (10-80 μg/mL) |
HR | Sinoatrial node cells | UPRmt gene activation is dependent of β-tubulin abundance | No | [155] |
Baicalein (5, 10, and 20 μM for 2 h) |
PA-induced myocardial injury | AC16 cell line | Downregulation of UPRmt by increasing Nrf2 signaling | Yes | [153] |
Salvianolic acid (10, 30, and 60 mg/Kg) |
LPS-induced heart injury | C57BL/6 mice | Activation and nuclear translocation of ATF5 promote UPRmt upregulation | Yes | [143] |
THC (50 mg/Kg/day for 4 weeks, via gavage) |
HF | C57BL/6 male mice (8-10-week-old) |
Positive regulation of PGC-1α/ATF5, ClpP, and LonP1 axis activating UPRmt | No | [95] |
Xingang (XYT) tablet (0.48 g/Kg/day for 7 days) |
HF | C57BL/6 male mice (8-10-week-old) |
Through RIPK3/FUNDC1 pathway increases UPRmt activation and mitophagy | No | [145] |
Quercetin (10, 30, and 50 mg/Kg/day) |
AMI | C57BL/6 male mice (7-9-week-old) |
Induces downregulation of DNA-PKcs, which slows aberrant UPRmt activation through inhibition of ATF5, ClpP, CHOP, and Hsp60 | No | [156] |
Metformin (300 mg/Kg/day for 10 weeks, via gavage) |
HF | SHR male rats | Increased expression of ATF5, LonP1, and Hsp60 through HSF-1/UPRmt signaling | No | [157] |
Choline (7 mg/Kg/day for 12 weeks, via i.p.) |
HF | Sprague-Dawley male rats (8-10-week-old) |
SIRT-3/AMPK-dependent UPRmt induction | No | [158] |
To describe examples of natural compounds, activation of FUNDC1 by oligomycin in IR activates UPRmt-related genes, such as hsp60, hsp10, lonp1, and clpP, which improves mitochondrial dynamics and alleviates myocardial damage[120]. In mice with lipopolysaccharide-induced cardiac injury, the activation of ATF5 by salvianolic acid results in its transcription and translocation to the nucleus, activating UPRmt[143]. Apigenin increases ATF5 and SIRT-1 expression, thereby reducing doxorubicin-induced cardiomyopathy[144]. Tetrahydrocurcumin (THC), the main metabolite of curcumin, induces cardioprotection in mice with HF by increasing the ATF5/CHOP signaling cascade and the ClpP and LonP1 proteases. Strikingly, inhibiting PGC-1α abrogates the protective effect of THC and the activation of UPRmt[95]. Meanwhile, treatment of HF mice with Xingang tablet (XYT) promoted upregulation of ATF5 and LonP1, resulting in improved cardiac function and structure. Simultaneously, FUNDC1-regulated mitophagy is initiated, which ameliorates mitochondrial dysfunction in HF mice[145].
Although not in the heart, these natural compounds have been considered anti-aging agents. For example, salvianolic acid improves mitochondrial function and NAD+ metabolism through SIRT1 activation during skin aging[146] and dementia[147]. Apigenin reduces skeletal muscle atrophy by increasing mitochondrial function, reducing apoptosis[148], and upregulating SIRT1 in aged oocytes[149]. Moreover, baicalein ameliorates aortic structural deterioration in aged rats induced by D-galactose[150], age-related Parkinson’s disease[151], and osteoporosis through FOXO-1 signaling[152]. Its effect on cardiomyocytes and UPRmt has only been described in palmitic acid-treated AC16 cells, in which increased Nrf2, inhibition of UPRmt, and alleviation of mitochondrial damage are related to the positive effect of baicalein[153]. Taken together, these studies suggest that natural compounds can activate UPRmt and induce homeostasis of mitochondrial function.
Furthermore, after transfection with lentivirus overexpressing C1q-tumor necrosis factor-related protein 3 (CTRP3) in HF mice, mitochondrial function and cardiomyocyte survival improved through the activation of the ATF5/SIRT-1 pathway[154]. Conversely, downregulation of UPRmt improved mitochondrial function and morphology in cardiomyocytes subjected to hypoxia/reoxygenation by enhanced therapy with the Chinese medicine Zishen Tongyang Huoxue (TYHX) decoction with β-tubulin stability[155]. In the same vein, a recent study indicated that in ischemia, the expression of the DNA-dependent protein kinase (DNA-Pkcs) catalytic subunit increased, but SIRT-5 decreased, leading to cardiac tissue damage. However, in mice treated with quercetin, aberrant UPRmt is arrested by a decrease in DNA-Pkcs[156]. Finally, other strategies that have been implemented include the use of metformin, which increases ATF5, LonP1, and Hsp60 in spontaneously hypertensive rats, and is associated with improved cardiac function, less fibrosis, and recovery of cellular proteostasis modulated by HSF-1/UPRmt[157]. Meanwhile, choline treatment ameliorates mitochondrial dysfunction through UPRmt in cardiac hypertrophy, although negative regulation of SIRT-3 inhibits the protective role of choline by low AMPK activation and thus impairs UPRmt[158]. Despite the significant advances in UPRmt as a possible biomarker of CVDs and the compounds that may have an effect on this pathway, more information is needed on what would occur in aged organisms since, as we have described, the effects may be different with respect to young and adult organisms.
CONCLUSION
The present review highlights UPRmt as a timely and innovative field of study in aging, whose response would allow the maintenance of mitochondrial homeostasis in aging organisms. In the coming years, we expect that scientific research will be directed toward describing the benefits of interorganellar communication in regulating UPRmt during aging, especially when suffering from chronic diseases such as heart disease. There is also a need to expand our knowledge about the possible association of changes in interorganellar contactology and UPRmt in the canonical mechanism of multiple therapeutic interventions that have been shown to help slow down the establishment of aging-related diseases and increase the lifespan of organisms.
DECLARATIONS
Authors’ contributions
Contributed intellectually and equally to the manuscript: Navarrete-Anastasio G, Ceja-Galicia ZA, Zazueta C, Silva-Palacios A
Reviewed the draft and critically revised the paper: Silva-Palacios A
All authors approved the final manuscript.
Availability of data and materials
Not applicable.
Financial support and sponsorship
None.
Conflicts of interest
All authors declared that there are no conflicts of interest.
Ethical approval and consent to participate
Not applicable.
Consent for publication
Not applicable.
Copyright
© The Author(s) 2025.
REFERENCES
1. Sheng Y, Yang G, Markovich Z, Han SM, Xiao R. Distinct temporal actions of different types of unfolded protein responses during aging. J Cell Physiol. 2021;236:5069-79.
2. Kohler A, Kohler V. Better together: interorganellar communication in the regulation of proteostasis. Contact. 2024;7:25152564241272245.
3. Dana AH, Alejandro SP. Role of sulforaphane in endoplasmic reticulum homeostasis through regulation of the antioxidant response. Life Sci. 2022;299:120554.
4. Li Y, Huang D, Jia L, et al. LonP1 links mitochondria-ER interaction to regulate heart function. Research. 2023;6:0175.
5. Metzger MB, Michaelis S. Analysis of quality control substrates in distinct cellular compartments reveals a unique role for Rpn4p in tolerating misfolded membrane proteins. Mol Biol Cell. 2009;20:1006-19.
6. Ciplys E, Samuel D, Juozapaitis M, Sasnauskas K, Slibinskas R. Overexpression of human virus surface glycoprotein precursors induces cytosolic unfolded protein response in Saccharomyces cerevisiae. Microb Cell Fact. 2011;10:37.
8. Cilleros-Holgado P, Gómez-Fernández D, Piñero-Pérez R, et al. mtUPR modulation as a therapeutic target for primary and secondary mitochondrial diseases. Int J Mol Sci. 2023;24:1482.
9. Ali MA, Gioscia-Ryan R, Yang D, Sutton NR, Tyrrell DJ. Cardiovascular aging: spotlight on mitochondria. Am J Physiol Heart Circ Physiol. 2024;326:H317-33.
10. Eckl EM, Ziegemann O, Krumwiede L, Fessler E, Jae LT. Sensing, signaling and surviving mitochondrial stress. Cell Mol Life Sci. 2021;78:5925-51.
11. Wang YT, Lim Y, McCall MN, et al. Cardioprotection by the mitochondrial unfolded protein response requires ATF5. Am J Physiol Heart Circ Physiol. 2019;317:H472-8.
12. Bar-Ziv R, Dutta N, Hruby A, et al. Glial-derived mitochondrial signals affect neuronal proteostasis and aging. Sci Adv. 2023;9:eadi1411.
13. Angeli S, Foulger A, Chamoli M, et al. The mitochondrial permeability transition pore activates the mitochondrial unfolded protein response and promotes aging. Elife. 2021;10:e63453.
14. Evangelou K, Vasileiou PVS, Papaspyropoulos A, et al. Cellular senescence and cardiovascular diseases: moving to the "heart" of the problem. Physiol Rev. 2023;103:609-47.
15. Zhang W, Che Y, Tang X, et al. A biomarker framework for cardiac aging: the Aging Biomarker Consortium consensus statement. Life Med. 2023;2:lnad035.
16. Csordás G, Weaver D, Hajnóczky G. Endoplasmic reticulum-mitochondrial contactology: structure and signaling functions. Trends Cell Biol. 2018;28:523-40.
17. Voeltz GK, Sawyer EM, Hajnóczky G, Prinz WA. Making the connection: How membrane contact sites have changed our view of organelle biology. Cell. 2024;187:257-70.
18. He H, Zeng B, Wu X, et al. Higher matrix stiffness promotes VSMC senescence by affecting mitochondria-ER contact sites and mitochondria/ER dysfunction. FASEB J. 2023;37:e23318.
19. Maddison DC, Malik B, Amadio L, et al. COPI-regulated mitochondria-ER contact site formation maintains axonal integrity. Cell Rep. 2023;42:112883.
20. Hinton A Jr, Katti P, Mungai M, et al. ATF4-dependent increase in mitochondrial-endoplasmic reticulum tethering following OPA1 deletion in skeletal muscle. J Cell Physiol. 2024;239:e31204.
21. Janer A, Morris JL, Krols M, et al. ESYT1 tethers the ER to mitochondria and is required for mitochondrial lipid and calcium homeostasis. Life Sci Alliance. 2024;7:e202302335.
22. Garcia BM, Machado TS, Carvalho KF, et al. Mice born to females with oocyte-specific deletion of mitofusin 2 have increased weight gain and impaired glucose homeostasis. Mol Hum Reprod. 2020;26:938-52.
23. Ziegler DV, Martin N, Bernard D. Cellular senescence links mitochondria-ER contacts and aging. Commun Biol. 2021;4:1323.
24. Silva-Palacios A, Zazueta C, Pedraza-Chaverri J. ER membranes associated with mitochondria: possible therapeutic targets in heart-associated diseases. Pharmacol Res. 2020;156:104758.
25. Casas-Martinez JC, Samali A, McDonagh B. Redox regulation of UPR signalling and mitochondrial ER contact sites. Cell Mol Life Sci. 2024;81:250.
26. Moqri M, Herzog C, Poganik JR, et al. Biomarkers of aging for the identification and evaluation of longevity interventions. Cell. 2023;186:3758-75.
27. Bao H, Cao J, Chen M, et al; Aging Biomarker Consortium. Biomarkers of aging. Sci China Life Sci. 2023;66:893-1066.
28. Sierra F, Kohanski R. Geroscience and the trans-NIH Geroscience Interest Group, GSIG. Geroscience. 2017;39:1-5.
29. López-Otín C, Blasco MA, Partridge L, Serrano M, Kroemer G. Hallmarks of aging: an expanding universe. Cell. 2023;186:243-78.
30. López-Otín C, Blasco MA, Partridge L, Serrano M, Kroemer G. The hallmarks of aging. Cell. 2013;153:1194-217.
31. Gil-Hernández A, Silva-Palacios A. Relevance of endoplasmic reticulum and mitochondria interactions in age-associated diseases. Ageing Res Rev. 2020;64:101193.
32. Camacho-Encina M, Booth LK, Redgrave RE, Folaranmi O, Spyridopoulos I, Richardson GD. Cellular senescence, mitochondrial dysfunction, and their link to cardiovascular disease. Cells. 2024;13:353.
33. Guo J, Huang X, Dou L, et al. Aging and aging-related diseases: from molecular mechanisms to interventions and treatments. Signal Transduct Target Ther. 2022;7:391.
34. Xu M, Pirtskhalava T, Farr JN, et al. Senolytics improve physical function and increase lifespan in old age. Nat Med. 2018;24:1246-56.
35. Jia K, Dai Y, Liu A, et al. Senolytic agent navitoclax inhibits angiotensin II-induced heart failure in mice. J Cardiovasc Pharmacol. 2020;76:452-60.
36. Sunderland P, Alshammari L, Ambrose E, Torella D, Ellison-hughes GM. Senolytics rejuvenate the reparative activity of human cardiomyocytes and endothelial cells. J Cardiovasc Aging. 2023;3:21.
37. Vue Z, Neikirk K, Vang L, et al. Three-dimensional mitochondria reconstructions of murine cardiac muscle changes in size across aging. Am J Physiol Heart Circ Physiol. 2023;325:H965-82.
38. Tang X, Li PH, Chen HZ. Cardiomyocyte senescence and cellular communications within myocardial microenvironments. Front Endocrinol. 2020;11:280.
39. Paneni F, Diaz Cañestro C, Libby P, Lüscher TF, Camici GG. The aging cardiovascular system: understanding it at the cellular and clinical levels. J Am Coll Cardiol. 2017;69:1952-67.
40. Lu X, Gong Y, Hu W, et al. Ultrastructural and proteomic profiling of mitochondria-associated endoplasmic reticulum membranes reveal aging signatures in striated muscle. Cell Death Dis. 2022;13:296.
41. Nieto M, Konigsberg M, Silva-Palacios A. Quercetin and dasatinib, two powerful senolytics in age-related cardiovascular disease. Biogerontology. 2024;25:71-82.
42. Charles AL, Charloux A, Vogel T, et al. Cumulative deleterious effects of tetrahydrocannabinoid (THC) and ethanol on mitochondrial respiration and reactive oxygen species production are enhanced in old isolated cardiac mitochondria. Int J Mol Sci. 2024;25:1835.
43. Rosa FLL, de Souza IIA, Monnerat G, Campos de Carvalho AC, Maciel L. Aging triggers mitochondrial dysfunction in mice. Int J Mol Sci. 2023;24:10591.
44. Schaum N, Lehallier B, Hahn O, et al. Ageing hallmarks exhibit organ-specific temporal signatures. Nature. 2020;583:596-602.
45. Sarver DC, Saqib M, Chen F, Wong GW. Mitochondrial respiration atlas reveals differential changes in mitochondrial function across sex and age. Elife. 2024;13:e96926.
46. Muris Consortium. A single-cell transcriptomic atlas characterizes ageing tissues in the mouse. Nature. 2020;583:590-5.
47. Zhang MJ, Pisco AO, Darmanis S, Zou J. Mouse aging cell atlas analysis reveals global and cell type-specific aging signatures. Elife. 2021;10:62293.
48. Lehallier B, Gate D, Schaum N, et al. Undulating changes in human plasma proteome profiles across the lifespan. Nat Med. 2019;25:1843-50.
49. Oh HS, Rutledge J, Nachun D, et al. Organ aging signatures in the plasma proteome track health and disease. Nature. 2023;624:164-72.
50. Lesnefsky EJ, Chen Q, Hoppel CL. Mitochondrial metabolism in aging heart. Circ Res. 2016;118:1593-611.
51. Fannin SW, Lesnefsky EJ, Slabe TJ, Hassan MO, Hoppel CL. Aging selectively decreases oxidative capacity in rat heart interfibrillar mitochondria. Arch Biochem Biophys. 1999;372:399-407.
52. García-Niño WR, Correa F, Rodríguez-Barrena JI, et al. Cardioprotective kinase signaling to subsarcolemmal and interfibrillar mitochondria is mediated by caveolar structures. Basic Res Cardiol. 2017;112:15.
53. Lesnefsky EJ, Gudz TI, Moghaddas S, et al. Aging decreases electron transport complex III activity in heart interfibrillar mitochondria by alteration of the cytochrome c binding site. J Mol Cell Cardiol. 2001;33:37-47.
54. Chen Q, Thompson J, Hu Y, Lesnefsky EJ. Aging-induced mitochondrial dysfunction: two distinct populations of mitochondria versus a combined population. Am J Physiol Heart Circ Physiol. 2024;326:H385-95.
55. Cogliati S, Enriquez JA, Scorrano L. Mitochondrial cristae: where beauty meets functionality. Trends Biochem Sci. 2016;41:261-73.
56. Morris S, Schmelter F, Molina-Riquelme I, et al. Decreased ATP synthase activity is linked to altered spatiotemporal organisation of ATP Synthase in a cellular cardiomyocyte senescent model. bioRxiv 2024.
57. Wang G, Luo Y, Hu J, Wang J, Liu X, Li S. Effects of aging on expression of Mic60 and OPA1 and mitochondrial morphology in myocardium of Tibetan sheep. Animals. 2020;10:2160.
58. Morris S, Molina-Riquelme I, Barrientos G, et al. Inner mitochondrial membrane structure and fusion dynamics are altered in senescent human iPSC-derived and primary rat cardiomyocytes. Biochim Biophys Acta Bioenergy. 2023;1864:148949.
59. Crabtree A, Neikirk K, Marshall AG, et al. Defining mitochondrial cristae morphology changes induced by aging in brown adipose tissue. Adv Biol. 2024;8:e2300186.
60. Campbell D, Zuryn S. The mechanisms and roles of mitochondrial dynamics in C. elegans. Semin Cell Dev Biol. 2024;156:266-75.
61. Mokhtari B, Hosseini L, Høilund-Carlsen PF, Salehinasab R, Rajabi M, Badalzadeh R. The additive effects of nicotinamide mononucleotide and melatonin on mitochondrial biogenesis and fission/fusion, autophagy, and microRNA-499 in the aged rat heart with reperfusion injury. Naunyn Schmiedebergs Arch Pharmacol. 2023;396:1701-11.
62. Chaudhari SN, Kipreos ET. Increased mitochondrial fusion allows the survival of older animals in diverse C. elegans longevity pathways. Nat Commun. 2017;8:182.
63. Liu YJ, McIntyre RL, Janssens GE, et al. Mitochondrial translation and dynamics synergistically extend lifespan in C. elegans through HLH-30. J Cell Biol. 2020;219:e201907067.
64. Cai Y, Shen H, Weng H, et al. Overexpression of PGC-1α influences the mitochondrial unfolded protein response (mtUPR) induced by MPP+ in human SH-SY5Y neuroblastoma cells. Sci Rep. 2020;10:10444.
65. Bomer N, Pavez-Giani MG, Deiman FE, et al. Selenoprotein DIO2 is a regulator of mitochondrial function, morphology and UPRmt in human cardiomyocytes. Int J Mol Sci. 2021;22:11906.
66. Mone P, Agyapong ED, Morciano G, et al. Dysfunctional mitochondria elicit bioenergetic decline in the aged heart. J Cardiovasc Aging. 2024;4:13.
67. Guo Z, Wang M, Ying X, et al. Caloric restriction increases the resistance of aged heart to myocardial ischemia/reperfusion injury via modulating AMPK-SIRT1-PGC1a energy metabolism pathway. Sci Rep. 2023;13:2045.
68. Shen G, Liu W, Xu L, Wang LL. Mitochondrial unfolded protein response and its roles in stem cells. Stem Cells Dev. 2020;29:627-37.
69. Yoneda T, Benedetti C, Urano F, Clark SG, Harding HP, Ron D. Compartment-specific perturbation of protein handling activates genes encoding mitochondrial chaperones. J Cell Sci. 2004;117:4055-66.
70. Nargund AM, Pellegrino MW, Fiorese CJ, Baker BM, Haynes CM. Mitochondrial import efficiency of ATFS-1 regulates mitochondrial UPR activation. Science. 2012;337:587-90.
71. Mouchiroud L, Houtkooper RH, Moullan N, et al. The NAD+/sirtuin pathway modulates longevity through activation of mitochondrial UPR and FOXO signaling. Cell. 2013;154:430-41.
72. Bennett CF, Vander Wende H, Simko M, et al. Activation of the mitochondrial unfolded protein response does not predict longevity in Caenorhabditis elegans. Nat Commun. 2014;5:3483.
73. Zhao Q, Wang J, Levichkin IV, Stasinopoulos S, Ryan MT, Hoogenraad NJ. A mitochondrial specific stress response in mammalian cells. EMBO J. 2002;21:4411-9.
74. Maragkakis M, Malla S, Hatzoglou M, et al. Biology of stress responses in aging. Aging Biol. 2023;1:20230001.
75. Lionaki E, Tavernarakis N. Oxidative stress and mitochondrial protein quality control in aging. J Proteomics. 2013;92:181-94.
76. Torres AK, Fleischhart V, Inestrosa NC. Mitochondrial unfolded protein response (UPRmt): what we know thus far. Front Cell Dev Biol. 2024;12:1405393.
77. Zhu D, Li X, Tian Y. Mitochondrial-to-nuclear communication in aging: an epigenetic perspective. Trends Biochem Sci. 2022;47:645-59.
78. Jovaisaite V, Mouchiroud L, Auwerx J. The mitochondrial unfolded protein response, a conserved stress response pathway with implications in health and disease. J Exp Biol. 2014;217:137-43.
79. Horibe T, Hoogenraad NJ. The chop gene contains an element for the positive regulation of the mitochondrial unfolded protein response. PLoS One. 2007;2:e835.
80. Aldridge JE, Horibe T, Hoogenraad NJ. Discovery of genes activated by the mitochondrial unfolded protein response (mtUPR) and cognate promoter elements. PLoS One. 2007;2:e874.
81. Zhou H, Yuan D, Gao W, et al. Loss of high-temperature requirement protein A2 protease activity induces mitonuclear imbalance via differential regulation of mitochondrial biogenesis in sarcopenia. IUBMB Life. 2020;72:1659-79.
82. Ristow M. Unraveling the truth about antioxidants: mitohormesis explains ROS-induced health benefits. Nat Med. 2014;20:709-11.
83. Rolland SG, Schneid S, Schwarz M, et al. Compromised mitochondrial protein import acts as a signal for UPRmt. Cell Rep. 2019;28:1659-69.e5.
84. Lee MJ, Jang Y, Zhu J, et al. Auraptene enhances junction assembly in cerebrovascular endothelial cells by promoting resilience to mitochondrial stress through activation of antioxidant enzymes and mtUPR. Antioxidants. 2021;10:475.
85. Pulliam DA, Deepa SS, Liu Y, et al. Complex IV-deficient Surf1-/- mice initiate mitochondrial stress responses. Biochem J. 2014;462:359-71.
86. Owusu-Ansah E, Song W, Perrimon N. Muscle mitohormesis promotes longevity via systemic repression of insulin signaling. Cell. 2013;155:699-712.
87. Zhao K, Huang X, Zhao W, Lu B, Yang Z. LONP1-mediated mitochondrial quality control safeguards metabolic shifts in heart development. Development. 2022;149:dev200458.
88. Zhang C, Shen S, Xu L, et al. LONP1 alleviates ageing-related renal fibrosis by maintaining mitochondrial homeostasis. J Cell Mol Med. 2024;28:e70090.
89. Meng J, Lv Z, Guo M, et al. A Lycium barbarum extract inhibits β-amyloid toxicity by activating the antioxidant system and mtUPR in a Caenorhabditis elegans model of Alzheimer's disease. FASEB J. 2022;36:e22156.
90. Papa L, Germain D. SirT3 regulates the mitochondrial unfolded protein response. Mol Cell Biol. 2014;34:699-710.
91. Shpilka T, Haynes CM. The mitochondrial UPR: mechanisms, physiological functions and implications in ageing. Nat Rev Mol Cell Biol. 2018;19:109-20.
92. Xu WN, Zheng HL, Yang RZ, et al. The mitochondrial UPR induced by ATF5 attenuates intervertebral disc degeneration via cooperating with mitophagy. Cell Biol Toxicol. 2024;40:16.
93. Ito A, Zhao Q, Tanaka Y, et al. Metolazone upregulates mitochondrial chaperones and extends lifespan in Caenorhabditis elegans. Biogerontology. 2021;22:119-31.
94. Wu J, Zeng Z, Zhang W, et al. Emerging role of SIRT3 in mitochondrial dysfunction and cardiovascular diseases. Free Radic Res. 2019;53:139-49.
95. Zhang B, Tan Y, Zhang Z, et al. Novel PGC-1α/ATF5 axis partly activates UPRmt and mediates cardioprotective role of tetrahydrocurcumin in pathological cardiac hypertrophy. Oxid Med Cell Longev. 2020;2020:9187065.
96. Chen S, Henderson A, Petriello MC, et al. Trimethylamine N-Oxide binds and activates PERK to promote metabolic dysfunction. Cell Metab. 2019;30:1141-51.e5.
97. Brunt VE, Gioscia-Ryan RA, Casso AG, et al. Trimethylamine-N-oxide promotes age-related vascular oxidative stress and endothelial dysfunction in mice and healthy humans. Hypertension. 2020;76:101-12.
98. An H, Zhou B, Hayakawa K, et al. ATF5-mediated mitochondrial unfolded protein response (UPRmt) protects neurons against oxygen-glucose deprivation and cerebral ischemia. Stroke. 2024;55:1904-13.
99. Guo Q, Xu Z, Zhou D, et al. Mitochondrial proteostasis stress in muscle drives a long-range protective response to alleviate dietary obesity independently of ATF4. Sci Adv. 2022;8:eabo0340.
100. Deng J, Wang D, Shi Y, et al. Mitochondrial unfolded protein response mechanism and its cardiovascular protective effects. Biomed Pharmacother. 2024;177:116989.
101. Hinton A Jr, Claypool SM, Neikirk K, et al. Mitochondrial structure and function in human heart failure. Circ Res. 2024;135:372-96.
102. Sun J, Tai S, Guo Y, et al. Sex differences in characteristics and outcomes in elderly heart failure patients with preserved ejection fraction: a post-hoc analysis from TOPCAT. Front Cardiovasc Med. 2021;8:721850.
103. Gharagozloo K, Mehdizadeh M, Heckman G, et al. Heart failure with preserved ejection fraction in the elderly population: basic mechanisms and clinical considerations. Can J Cardiol. 2024;40:1424-44.
104. Hoshino A, Okawa Y, Ariyoshi M, et al. Oxidative post-translational modifications develop LONP1 dysfunction in pressure overload heart failure. Circ Heart Fail. 2014;7:500-9.
105. Bozi LH, Jannig PR, Rolim N, et al. Aerobic exercise training rescues cardiac protein quality control and blunts endoplasmic reticulum stress in heart failure rats. J Cell Mol Med. 2016;20:2208-12.
106. Schiattarella GG, Altamirano F, Tong D, et al. Nitrosative stress drives heart failure with preserved ejection fraction. Nature. 2019;568:351-6.
107. Kitakata H, Endo J, Hashimoto S, et al. Imeglimin prevents heart failure with preserved ejection fraction by recovering the impaired unfolded protein response in mice subjected to cardiometabolic stress. Biochem Biophys Res Commun. 2021;572:185-90.
108. Smyrnias I, Gray SP, Okonko DO, et al. Cardioprotective effect of the mitochondrial unfolded protein response during chronic pressure overload. J Am Coll Cardiol. 2019;73:1795-806.
109. Guo Y, Wang Z, Qin X, et al. Enhancing fatty acid utilization ameliorates mitochondrial fragmentation and cardiac dysfunction via rebalancing optic atrophy 1 processing in the failing heart. Cardiovasc Res. 2018;114:979-91.
110. Pryde KR, Taanman JW, Schapira AH. A LON-ClpP Proteolytic axis degrades complex I to extinguish ROS production in depolarized mitochondria. Cell Rep. 2016;17:2522-31.
111. Wai T, García-Prieto J, Baker MJ, et al. Imbalanced OPA1 processing and mitochondrial fragmentation cause heart failure in mice. Science. 2015;350:aad0116.
112. Fan F, Duan Y, Yang F, et al. Deletion of heat shock protein 60 in adult mouse cardiomyocytes perturbs mitochondrial protein homeostasis and causes heart failure. Cell Death Differ. 2020;27:587-600.
113. Svagusa T, Sikiric S, Milavic M, et al. Heart failure in patients is associated with downregulation of mitochondrial quality control genes. Eur J Clin Invest. 2023;53:e14054.
114. Mirosevic V, Svagusa T, Matic N, et al. Cardiotoxicity of iron and zinc and their association with the mitochondrial unfolded protein response in humans. Int J Mol Sci. 2024;25:9648.
115. Damluji AA, van Diepen S, Katz JN, et al. Mechanical complications of acute myocardial infarction: a scientific statement from the American heart association. Circulation. 2021;144:e16-35.
116. Liu G, Wang M, Lv X, Guan Y, Li J, Xie J. Identification of mitochondria-related gene biomarkers associated with immune infiltration in acute myocardial infarction. iScience. 2024;27:110275.
117. Arroyo-Campuzano M, Gil-Hernández A, Silva-Palacios A. Cardiosome-mediated protection in myocardial ischemia. Clin Chim Acta. 2023;545:117374.
118. Mariani J, Ou R, Bailey M, et al. Tolerance to ischemia and hypoxia is reduced in aged human myocardium. J Thorac Cardiovasc Surg. 2000;120:660-7.
119. Venkatesh S, Li M, Saito T, et al. Mitochondrial LonP1 protects cardiomyocytes from ischemia/reperfusion injury in vivo. J Mol Cell Cardiol. 2019;128:38-50.
120. Ji H, Wang J, Muid D, Song W, Jiang Y, Zhou H. FUNDC1 activates the mitochondrial unfolded protein response to preserve mitochondrial quality control in cardiac ischemia/reperfusion injury. Cell Signal. 2022;92:110249.
121. Lee CK, Klopp RG, Weindruch R, Prolla TA. Gene expression profile of aging and its retardation by caloric restriction. Science. 1999;285:1390-3.
122. Bronikowski AM, Carter PA, Morgan TJ, et al. Lifelong voluntary exercise in the mouse prevents age-related alterations in gene expression in the heart. Physiol Genomics. 2003;12:129-38.
123. Rojas-Morales P, León-Contreras JC, Granados-Pineda J, et al. Protection against renal ischemia and reperfusion injury by short-term time-restricted feeding involves the mitochondrial unfolded protein response. Free Radic Biol Med. 2020;154:75-83.
124. Wang K, Zhang J, Liu J, et al. Variations in the protein level of Omi/HtrA2 in the heart of aged rats may contribute to the increased susceptibility of cardiomyocytes to ischemia/reperfusion injury and cell death: Omi/HtrA2 and aged heart injury. Age. 2013;35:733-46.
125. Li X, Zhang X, Zeng Z, et al. Serum albumin and prognosis in elderly patients with nonischemic dilated cardiomyopathy. J Cardiovasc Med. 2023;24:752-7.
126. Jin L, Geng L, Ying L, et al. FGF21-sirtuin 3 axis confers the protective effects of exercise against diabetic cardiomyopathy by governing mitochondrial integrity. Circulation. 2022;146:1537-57.
127. Nandi SS, Katsurada K, Mahata SK, Patel KP. Neurogenic hypertension mediated mitochondrial abnormality leads to cardiomyopathy: contribution of UPRmt and Norepinephrine-miR-18a-5p-HIF-1α Axis. Front Physiol. 2021;12:718982.
128. Tezze C, Romanello V, Desbats MA, et al. Age-associated loss of OPA1 in muscle impacts muscle mass, metabolic homeostasis, systemic inflammation, and epithelial senescence. Cell Metab. 2017;25:1374-89.e6.
129. Ahola S, Pazurek LA, Mayer F, et al. Opa1 processing is dispensable in mouse development but is protective in mitochondrial cardiomyopathy. Sci Adv. 2024;10:eadp0443.
130. Seiferling D, Szczepanowska K, Becker C, et al. Loss of CLPP alleviates mitochondrial cardiomyopathy without affecting the mammalian UPRmt. EMBO Rep. 2016;17:953-64.
131. Longhitano L, Distefano A, Musso N, et al. (+)-Lipoic acid reduces mitochondrial unfolded protein response and attenuates oxidative stress and aging in an in vitro model of non-alcoholic fatty liver disease. J Transl Med. 2024;22:82.
132. Xiaowei X, Qian X, Dingzhou Z. Sirtuin-3 activates the mitochondrial unfolded protein response and reduces cerebral ischemia/reperfusion injury. Int J Biol Sci. 2023;19:4327-39.
133. Barcena ML, Pozdniakova S, Haritonow N, et al. Dilated cardiomyopathy impairs mitochondrial biogenesis and promotes inflammation in an age- and sex-dependent manner. Aging. 2020;12:24117-33.
134. Dikalova AE, Pandey A, Xiao L, et al. Mitochondrial deacetylase Sirt3 reduces vascular dysfunction and hypertension while Sirt3 depletion in essential hypertension is linked to vascular inflammation and oxidative stress. Circ Res. 2020;126:439-52.
135. Nichtová Z, Fernandez-Sanz C, De La Fuente S, et al. Enhanced mitochondria-SR tethering triggers adaptive cardiac muscle remodeling. Circ Res. 2023;132:e171-87.
136. Bosc C, Broin N, Fanjul M, et al. Autophagy regulates fatty acid availability for oxidative phosphorylation through mitochondria-endoplasmic reticulum contact sites. Nat Commun. 2020;11:4056.
137. Ching C, Maufront J, di Cicco A, Lévy D, Dezi M. Cool-contacts: cryo-electron microscopy of membrane contact sites and their components. Contact. 2024;7:25152564241231364.
138. Li Y, Tian X, Yu Q, et al. Alleviation of hepatic insulin resistance and steatosis with NMN via improving endoplasmic reticulum-Mitochondria miscommunication in the liver of HFD mice. Biomed Pharmacother. 2024;175:116682.
139. Sun Y, Cai Y, Qian S, Chiou H, Zang QS. Beclin-1 improves mitochondria-associated membranes in the heart during endotoxemia. FASEB Bioadv. 2021;3:123-35.
140. McCandless MG, Altara R, Booz GW, Kurdi M. What role do mitochondria have in diastolic dysfunction? Implications for diabetic cardiomyopathy and heart failure with preserved ejection function. J Cardiovasc Pharmacol. 2022;79:399-406.
141. Ren L, Gopireddy RR, Perkins G, et al. Disruption of mitochondria-sarcoplasmic reticulum microdomain connectomics contributes to sinus node dysfunction in heart failure. Proc Natl Acad Sci USA. 2022;119:e2206708119.
142. Zhang P, Konja D, Zhang Y, et al. Clusterin is involved in mediating the metabolic function of adipose SIRT1. iScience. 2022;25:103709.
143. Chen R, Zheng A, Wang Y, et al. Salvianolic acid B improves mitochondrial dysfunction of septic cardiomyopathy via enhancing ATF5-mediated mitochondrial unfolded protein response. Toxicol Appl Pharmacol. 2024;491:117072.
144. Li H, Chen D, Zhang X, et al. Screening of an FDA-approved compound library identifies apigenin for the treatment of myocardial injury. Int J Biol Sci. 2023;19:5233-44.
145. Dong X, Zhuang HW, Wen RJ, et al. Xinyang tablet alleviated cardiac dysfunction in a cardiac pressure overload model by regulating the receptor-interacting serum/three-protein kinase 3/FUN14 domain containing 1-mediated mitochondrial unfolded protein response and mitophagy. J Ethnopharmacol. 2024;330:118152.
146. Sun JM, Liu YX, Liu YD, et al. Salvianolic acid B protects against UVB-induced skin aging via activation of NRF2. Phytomedicine. 2024;130:155676.
147. Ma X, Xu W, Zhang Z, et al. Salvianolic acid B ameliorates cognitive deficits through IGF-1/Akt pathway in rats with vascular dementia. Cell Physiol Biochem. 2017;43:1381-91.
148. Wang D, Yang Y, Zou X, Zhang J, Zheng Z, Wang Z. Antioxidant apigenin relieves age-related muscle atrophy by inhibiting oxidative stress and hyperactive mitophagy and apoptosis in skeletal muscle of mice. J Gerontol A Biol Sci Med Sci. 2020;75:2081-8.
149. Yao X, Guo P, Li YH, et al. Apigenin delays postovulatory oocyte aging by reducing oxidative stress through SIRT1 upregulation. Theriogenology. 2024;218:89-98.
150. Ma Y, Ma Z, Zhang Y, et al. Apigenin and baicalein ameliorate thoracic aortic structural deterioration and cognitive deficit via inhibiting AGEs/RAGE/NF-κB pathway in D-galactose-induced aging rats. Eur J Pharmacol. 2024;976:176660.
151. Wang Y, Wei N, Li X. Preclinical evidence and possible mechanisms of baicalein for rats and mice with parkinson's disease: a systematic review and meta-analysis. Front Aging Neurosci. 2020;12:277.
152. Cai P, Lu Y, Yin Z, Wang X, Zhou X, Li Z. Baicalein ameliorates osteoporosis via AKT/FOXO1 signaling. Aging. 2021;13:17370-9.
153. Guo L, Yang J, Yuan W, et al. Baicalein ameliorated obesity-induced cardiac dysfunction by regulating the mitochondrial unfolded protein response through NRF2 signaling. Phytomedicine. 2024;126:155441.
154. Shi L, Tan Y, Zheng W, et al. CTRP3 alleviates mitochondrial dysfunction and oxidative stress injury in pathological cardiac hypertrophy by activating UPRmt via the SIRT1/ATF5 axis. Cell Death Discov. 2024;10:53.
155. Chang X, Zhou S, Liu J, et al. Zishen Tongyang Huoxue decoction (TYHX) alleviates sinoatrial node cell ischemia/reperfusion injury by directing mitochondrial quality control via the VDAC1-β-tubulin signaling axis. J Ethnopharmacol. 2024;320:117371.
156. Chang X, Zhang Q, Huang Y, et al. Quercetin inhibits necroptosis in cardiomyocytes after ischemia-reperfusion via DNA-PKcs-SIRT5-orchestrated mitochondrial quality control. Phytother Res. 2024;38:2496-517.
157. Xu M, Li LP, He X, et al. Metformin induction of heat shock factor 1 activation and the mitochondrial unfolded protein response alleviate cardiac remodeling in spontaneously hypertensive rats. FASEB J. 2024;38:e23654.
Cite This Article

How to Cite
Download Citation
Export Citation File:
Type of Import
Tips on Downloading Citation
Citation Manager File Format
Type of Import
Direct Import: When the Direct Import option is selected (the default state), a dialogue box will give you the option to Save or Open the downloaded citation data. Choosing Open will either launch your citation manager or give you a choice of applications with which to use the metadata. The Save option saves the file locally for later use.
Indirect Import: When the Indirect Import option is selected, the metadata is displayed and may be copied and pasted as needed.
About This Article
Copyright
Data & Comments
Data
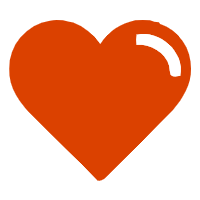
Comments
Comments must be written in English. Spam, offensive content, impersonation, and private information will not be permitted. If any comment is reported and identified as inappropriate content by OAE staff, the comment will be removed without notice. If you have any queries or need any help, please contact us at [email protected].